DOI:
10.1039/C5RA03139G
(Paper)
RSC Adv., 2015,
5, 32072-32077
Fabrication of mono-dispersed silica-coated quantum dot-assembled magnetic nanoparticles†
Received
19th February 2015
, Accepted 30th March 2015
First published on 30th March 2015
Abstract
Multifunctional nanoparticles (NPs) with magnetic and luminescent properties have garnered considerable attention in various fields of biomedical and physiological applications. In this study, we report the fabrication of QD-embedded silica NPs with an iron oxide NP core (Fe3O4@SiO2@QDs NPs) that has dual functional properties. The Fe3O4@SiO2@QDs NPs were mono-dispersed in size and exhibited super-paramagnetic and highly fluorescent properties. Most of the Fe3O4@SiO2@QDs NPs were naturally internalized into MDA-MB-231 human breast cancer cells, and the NP containing cells were successfully sorted by utilizing both fluorescence flow cytometry and a magnetic field. Results indicate that the Fe3O4@SiO2@QDs NPs have great potential for multimodal cell separation.
Introduction
Multifunctional nanoparticles (NPs) with both magnetic and luminescent properties have great potential for applications in biomedical research fields. NPs have been exploited in numerous studies including rapid bioseparation,1–5 biolabeling,6 targeted drug delivery,7 and in magnetic resonance imaging (MRI) as dual modal nanoprobes.8 Multifunctional NPs with enhanced luminescent brightness and magnetic behavior can drastically improve the performance of the aforementioned bioapplications.9
Semiconductor nanocrystals, known as quantum dots (QDs), have been considered as promising fluorophores for biomedical research because of their highly fluorescent quantum yield (QY), good photostability, wide absorption spectra, and narrow emission bandwidths.10–12 However, because QDs exhibit hydrophobic properties, surface modification is essential before use in bioapplications.13–15 Among the surface modification methods, silica encapsulation has been widely employed because of its numerous advantages such as compatibility with aqueous environment, easy functionalization, and good chemical stability.3,16–18 However, significant decrease in the QY of the employed QDs is inevitable during the encapsulation process. Recently, several efforts to facilitate the silica encapsulation of QDs within magnetic NPs have been reported, thereby enabling the preparation of multimodal NPs without significant loss in QY.19–21 However, the fabricated NPs revealed poly-dispersed size distribution. For reliable application to biological systems, preparation of nanomaterials that are mono-dispersed, highly fluorescent, and magnetic is crucial.
Recently, we reported on the fabrication of QDs-embedded silica NPs (SiO2@QDs NPs) for biomedical applications.22 The fabrication process yielded an efficient assembly of approximately 500 QDs on a silica NP (approximately 150 nm), without significant loss in QY. The particle size of the SiO2@QDs NPs was uniform and easily tunable by controlling the size of the silica core.
In this study, we describe the preparation of QDs-embedded silica NPs, whose core has an iron oxide NP. These multifunctional NPs, denoted as Fe3O4@SiO2@QDs NPs, are uniform in size and super-paramagnetic with intense fluorescence. Fe3O4@SiO2@QDs NPs were characterized using two kinds of cell separation methods: fluorescence-activated cell sorting (FACS) and magnetic field induced cell separation. These assessments are performed to determine the potential of Fe3O4@SiO2@QDs NPs as a tool for biomedical applications, particularly multimodal bioseparation.
Experimental details
Chemicals
All chemicals were used as received without further purification. A dispersion of Fe3O4 NPs (18 nm in average diameter, oleate-stabilized in toluene) was purchased from Ocean Nanotech, USA. Polyvinylpyrrolidone (PVP-10k), tetraethyl orthosilicate (TEOS), and 3-mercaptopropyltriethoxysilane (MPTS) were purchased from Sigma-Aldrich, Korea. Diethyl ether (anhydrous), dimethylformamide (DMF), dichloromethane (DCM), ethanol (EtOH), and ammonium hydroxide (28 wt% in water) were obtained from Daejung Parm, Korea. A dispersion of QDs (core–multishell structure composed of CdSe@CdS@ZnS, ∼4.5 nm in average diameter, oleate-stabilized in toluene) was purchased from Nanosquare, Korea.
Ligand exchange of oleate-stabilized Fe3O4 NPs with PVP
A 0.2 mL mixture of oleate-stabilized Fe3O4 NPs in toluene was transferred to a 10 mL vial and diluted with 5 mL of DMF–DCM (1
:
1, v/v). Then, 60 mg of PVP was added and refluxed overnight at 100 °C. After the reflux, the reaction mixture was added to diethyl ether (10 mL), and subsequently precipitated to obtain PVP-stabilized Fe3O4 NPs. The precipitate was centrifuged at 4500 rpm for 5 min and then transferred to 6.5 mL of EtOH to facilitate the formation of a stable dispersion of Fe3O4 NPs.
Silica coating on PVP-stabilized Fe3O4 NPs
The silica coating procedure on PVP-stabilized Fe3O4 NPs was based on a modified Stöber method.23,24 A 0.28 mL solution of ammonium hydroxide (28 wt% in water) was added to the EtOH dispersion of PVP-stabilized Fe3O4 NPs (6.5 mL), followed by the addition of TEOS (6.5 μL). The reaction mixture was stirred overnight at 25 °C. The silica-coated Fe3O4 NPs were then centrifuged at 10
000 rpm for 1 h, washed repeatedly with EtOH, and re-dispersed in distilled water. A 4 mL solution of TEOS in EtOH (3 vol%) was added to water containing the silica-coated Fe3O4 NPs, and then stirred for one day. The resulting Fe3O4@SiO2 NPs were centrifuged at 7000 rpm for 10 min and re-dispersed in EtOH.
Preparation of QDs-embedded Fe3O4@SiO2 NPs
Thiol groups were introduced to the surface of Fe3O4@SiO2 NPs (5 mL, 0.6 mg mL−1 suspension in EtOH) by adding MPTS (25 μL) and ammonium hydroxide (25 μL, 28 wt% in water), and by stirring overnight at 25 °C. These thiol-functionalized Fe3O4@SiO2 NPs were then washed three times with EtOH and re-dispersed in EtOH. The resulting NPs (1 mL, 10 mg mL−1 in EtOH) were subsequently added to QDs containing DCM solution (4 mL, 1.75 mg mL−1), and vigorously stirred for 3 min. Subsequently, MPTS (55 μL) and ammonium hydroxide (55 μL, 28 wt% in water) were added to the Fe3O4@SiO2@QDs NPs mixture, and was shaken for 1 h at 25 °C. The resulting mixtures were then washed with EtOH, dispersed in EtOH (5 mL) containing TEOS (55 μL) and ammonium hydroxide (55 μL), and stirred overnight. The obtained Fe3O4@SiO2@QDs NPs were washed three times with EtOH and subsequently dispersed in EtOH.
Characterization of the prepared NPs
Transmission electron microscopy (TEM) images were obtained with a JEOL JEM1010. The hydrodynamic size and the degree of dispersion of the NPs were determined using a Nanosight LM10. Ultraviolet-visible (UV-vis) absorption spectra were obtained with an Optigen 2120UV. Photoluminescence (PL) spectra were obtained with a Perkin-Elmer LS55. The QY of the prepared NPs was obtained with a JASCO FP-6500 equipped with an integrating sphere and a QY calculation program. The fluorescence decay of the prepared NPs was obtained by a time-correlated single photon counting (TCSPC) technique using a 405 nm diode laser at a repetition rate of 10 MHz. Field-dependent magnetization of the Fe3O4-based NPs was performed with a Quantum Design PPMS-14.
Confocal laser microscopy and flow cytometry analysis
For confocal microscopy analysis, MDA-MB-231 cells (1 × 105) were seeded on clean coverslips in a 6-well plate, followed by incubation for 24 h at 37 °C. The cells were rinsed using phosphate buffered saline (PBS), treated with Fe3O4@SiO2@QDs NPs (40 μg mL−1 in PBS), and incubated for 1 h at 37 °C. The cells treated with Fe3O4@SiO2@QDs NPs were fixed by treating with 4% paraformaldehyde solution (Affymetrix, USA) for 15 min. The cells were thoroughly washed three times with PBS and attached to the coverslip using a mounting solution containing 4′,6-diamidino-2-phenylindole (DAPI) solution (Vector Laboratories, USA). Confocal microscopy images were obtained using a Zeiss LSM 510. For magnetic separation of NP-treated cells, each of the two types of NPs, namely, non-magnetic SiO2@red-QDs NPs and magnetic Fe3O4@SiO2@green-QDs NPs (40 μg mL−1 in PBS), were incubated with MDA-MB-231 cells at 37 °C. After 1 h, the NPs-treated cells were harvested and combined. A magnet was located on one side of the well plate, and the floating cells were incubated with the magnet at room temperature for 4 h. After an additional 24 h of incubation at 37 °C in the absence of the magnet, the cells were fixed and analyzed with confocal microscopy. Flow cytometric analysis (BD FACS Calibur-2, USA) was performed with a cell mixture composed of the same numbers of SiO2@red-QDs NP-internalized and SiO2@green-QDs NP-internalized cells. The mixture was analyzed by laser excitation at 488 nm, with 530/30 nm and 661/16 nm emission filters.
Results and discussion
Fe3O4@SiO2@QDs NPs (Fig. 1) were prepared by first synthesizing Fe3O4@SiO2 NPs using the method described previously.24 Briefly, the Fe3O4@SiO2 NPs were prepared by subjecting Fe3O4 NPs to silica encapsulation by a modified Stöber method.23 First, amphiphilic PVP was used to replace oleic acid as the surface ligand of the Fe3O4 NPs (18 nm in average diameter) to obtain a hydrophilic surface. As shown in Fig. 2a and b, the size and shape of the Fe3O4 NPs remained unchanged during the ligand exchange process. The Fe3O4@SiO2 NPs were formed after reacting with TEOS and ammonium hydroxide, as shown in the TEM image of Fig. 2c. The resulting NPs have a magnetic core and silica shell, exhibiting uniform average particle size (75 ± 2.6 nm) and hydrodynamic size (Fig. S1a†). The average size and standard deviation of the prepared NPs were determined by analyzing fifty randomly selected sample images from the obtained TEM micrographs.
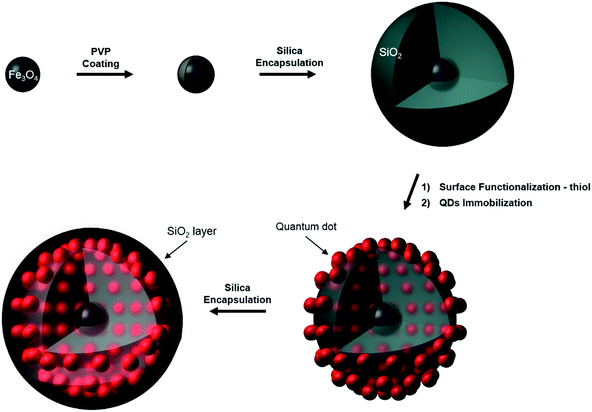 |
| Fig. 1 Illustration of the synthesis of Fe3O4@SiO2@QDs NPs. | |
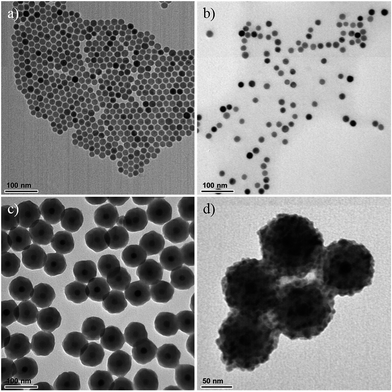 |
| Fig. 2 TEM images of NPs at each step of the synthesis. (a) Oleate-coated Fe3O4 NPs, (b) PVP-coated Fe3O4 NPs, (c) Fe3O4@SiO2 NPs, (d) Fe3O4@SiO2@QDs NPs. | |
The Fe3O4@SiO2 NPs were then functionalized with a thiol silicate monomer, MPTS, to facilitate the immobilization of the CdSe@CdS@ZnS QDs. The QDs immobilization and silica shell addition were performed using the same method described previously.22 First, controlled amounts of an EtOH solution containing the thiol-functionalized Fe3O4@SiO2 NPs and a DCM solution containing the QDs were mixed. The thiol groups of the Fe3O4@SiO2 NPs bound to the Zn atoms on the surface of CdSe@CdS@ZnS QDs, replacing the organic ligands.13 Subsequently, MPTS was added to the QDs-immobilized Fe3O4@SiO2 NPs to replace the remaining organic ligands, and hydrolyzed into silanol groups, forming a thin silica-shell precursor on the surface of the NPs. The NPs were then encapsulated with a silica shell by TEOS. As shown in Fig. 2d, the size of Fe3O4@SiO2@QDs NPs was ∼100 nm, which is slightly larger than that of the bare Fe3O4@SiO2 NPs; this is because of the addition of QDs and a thin silica shell layer on the surface. Significant aggregation of NPs was not detected by dynamic light scattering analysis (Fig. S1b†), and the obtained NPs were uniform in size as determined by TEM analysis (97 ± 4.8 nm).
First, the fluorescence properties of the NPs were analyzed. The Fe3O4@SiO2@red-QDs NPs and the Fe3O4@SiO2@green-QDs NPs were both excited at the wavelength of 350 nm using a xenon lamp, and exhibited bright photoluminescence with emission maxima at 620 and 540 nm (Fig. 3a), respectively, which correspond to those observed in the case of the original single QDs (Fig. S2†). The prepared NPs exhibited overlapping UV absorption spectra of the Fe3O4@SiO2 NPs and original single QDs with small knolls at 600 and 510 nm, indicating Stokes shifts of the red and green QDs. The original red QDs in the DCM solution had a QY of 86%. The QY of the Fe3O4@SiO2@red-QDs NPs was determined to be 57%, which is slightly less than that (71%) measured for the bare SiO2@red-QDs NPs. The QY of the original green QDs in the DCM solution was determined to be 70%, and the QYs of the SiO2@green-QDs NPs and Fe3O4@SiO2@green-QDs NPs were 63% and 40%, respectively. To clarify the QY difference between the Fe3O4@SiO2@QDs NPs and SiO2@QDs NPs, TCSPC detection was performed to clarify the QY difference between the Fe3O4@SiO2@QDs NPs and SiO2@QDs NPs. As shown in Fig. S3,† the Fe3O4@SiO2@QDs NPs show a short fluorescence lifetime compared to the SiO2@QDs NPs, indicating that the inserted Fe3O4 NPs could quench the QD fluorescence by electronic coupling and energy transfer.25 The magnetic properties of the Fe3O4@SiO2@QDs NPs were also measured to demonstrate that they can be employed for cell separation. The field-dependent magnetism of the Fe3O4@SiO2@QDs NPs at 300 K exhibited super-paramagnetic characteristics (Fig. 3b), with a saturated magnetization of ∼1.25 emu g−1. Furthermore, the NPs could be collected using a NdFeB magnet (Fig. 3c) and then clearly re-dispersed under gentle agitation. After agitation, the Fe3O4@SiO2@QDs NPs were found to be well-dispersed in various hydrophilic solvents (Fig. 3d) owing to their hydrophilic silica surface.
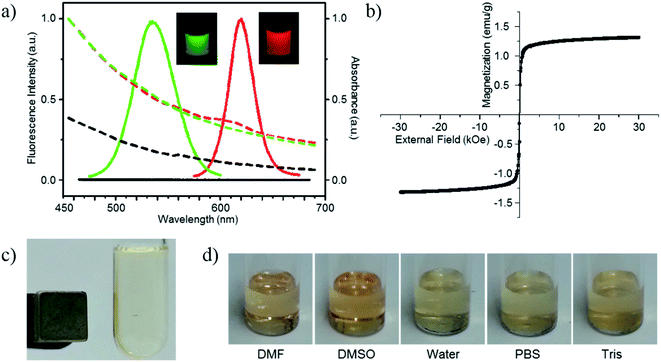 |
| Fig. 3 Various properties of Fe3O4@SiO2@QDs NPs. (a) UV absorption (dashed line) and fluorescence emission (straight line) spectrum of red and green QDs-embedded Fe3O4@SiO2@QDs NPs and Fe3O4@SiO2 NPs (inset: photographs of red and green QDs-embedded Fe3O4@SiO2@QDs NPs), (b) hysteresis loop of Fe3O4@SiO2@QDs NPs, (c) NP separation using NdFeB magnet, (d) dispersed Fe3O4@SiO2@QDs NPs in various solvents. | |
In vitro analysis was subsequently performed using both fluorescence and magnetic field to show that the NPs could be exploited for cell separation. The natural cell uptake of the prepared NPs was confirmed by incubating MDA-MB-231 human breast cancer cells with Fe3O4@SiO2@QDs NPs for 1 h. Confocal microscopy images (Fig. 4a) confirmed that most of the Fe3O4@SiO2@QDs NPs were accumulated in the cytoplasm of the cells by natural endocytosis. In order to verify the applicability of Fe3O4@SiO2@QDs NPs in FACS experiments, cells containing red or green QDs-embedded NPs were then analyzed using flow cytometry. Each cell was excited by a 488 nm laser source. As shown in the scatter plots of Fig. 4b, the cells can be clearly distinguished from the 661/16 nm and 530/30 nm emission filters. These results indicate that cells containing Fe3O4@SiO2@QDs NPs are suitable for use with flow cytometry.
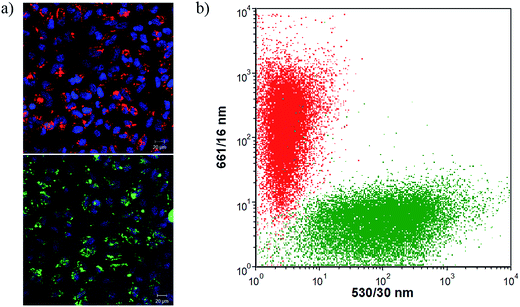 |
| Fig. 4 Cellular internalization assessment of Fe3O4@SiO2@QDs NPs. (a) Fluorescence images of MDA-MB-231 cells that internalized Fe3O4@SiO2@red-QDs NPs (upper panel) and the cells that internalized Fe3O4@SiO2@green-QDs NPs (lower panel). (b) Scatter plot of the fluorescence-activated cell sorting (FACS) analysis of cells that had internalized either Fe3O4@SiO2@red-QDs NPs or Fe3O4@SiO2@green-QDs NPs. | |
Furthermore, the ability of the Fe3O4@SiO2@QDs NPs to undergo magnetic separation was confirmed. Thus, cells that had internalized non-magnetic SiO2@red-QDs NPs or Fe3O4@SiO2@green-QDs NPs were harvested separately, mixed in equal amounts, and then placed on a 6-well plate near a NdFeB magnet for 4 h. The regions of the plate adjacent to and distant from the magnet were simultaneously excited by a 405 nm laser and examined using confocal microscopy. As shown in Fig. 5, the cells that internalized Fe3O4@SiO2@green-QDs NPs were mostly observed in regions near the magnet, while the cells that internalized non-magnetic SiO2@red-QDs NPs were located at the region distant from the magnet; i.e., most of the magnetized cells were accumulated close to the magnet. These results demonstrate the potential use of the Fe3O4@SiO2@QDs NPs in magnetic field-assisted cell separation.
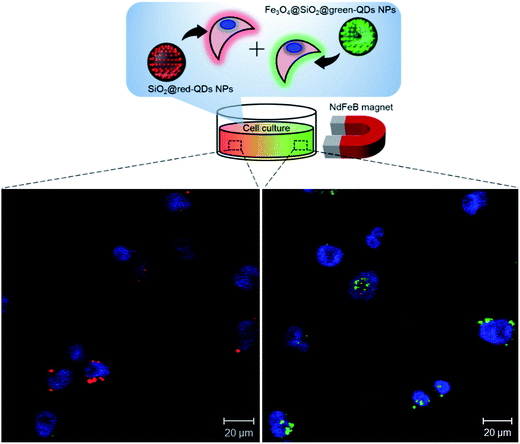 |
| Fig. 5 Illustration of magnetic field-assisted cell separation using Fe3O4@SiO2@QDs NPs and microscopic images of the separated cells containing either magnetic Fe3O4@SiO2@green-QDs NPs or nonmagnetic SiO2@red-QDs NPs. Sample plate regions (a) adjacent to and (b) distant from the magnet. | |
Conclusion
Multifunctional NPs of uniform size that have strong fluorescence and super-paramagnetic properties were fabricated by immobilizing QDs onto the surface of silica-coated iron oxide NPs. The applicability of Fe3O4@SiO2@QDs NPs in flow cytometry and magnetic-assisted cell separation was demonstrated. Our results prove that Fe3O4@SiO2@QDs NPs have a great potential for use in biomedical applications, particularly for multimodal cell separation.
Acknowledgements
The authors are very grateful to Prof. Seong Keun Kim and Jooyoun Kang for help in fluorescence lifetime analysis. This work was supported by the Israel-Korea Scientific Research Cooperation Program (Grant number 2012K1A3A1A31055183) through the National Research Foundation of Korea, funded by the Ministry of Science, ICT & Future Planning, and the Korean Health Technology R&D Project (Grant number HI13C-1299-0100), funded by the Ministry of Health & Welfare, by the Ministry of Science, ICT & Future Planning (2014-A002-0065).
References
- D. K. Yi, et al., Silica-coated nanocomposites of magnetic nanoparticles and quantum dots, J. Am. Chem. Soc., 2005, 127(14), 4990–4991 CrossRef CAS PubMed
. - J. Bao, et al., Bifunctional Au-Fe3O4 nanoparticles for protein separation, ACS Nano, 2007, 1(4), 293–298 CrossRef CAS PubMed
. - N. Insin, et al., Incorporation of iron oxide nanoparticles and quantum dots into silica microspheres, ACS Nano, 2008, 2(2), 197–202 CrossRef CAS PubMed
. - M. S. Noh, et al., Magnetic surface-enhanced Raman spectroscopic (M-SERS) dots for the identification of bronchioalveolar stem cells in normal and lung cancer mice, Biomaterials, 2009, 30(23–24), 3915–3925 CrossRef CAS PubMed
. - R. Di Corato, et al., Multifunctional Nanobeads Based on Quantum Dots and Magnetic Nanoparticles: Synthesis and Cancer Cell Targeting and Sorting, ACS Nano, 2011, 5(2), 1109–1121 CrossRef CAS PubMed
. - J. H. Gao, H. W. Gu and B. Xu, Multifunctional Magnetic Nanoparticles: Design, Synthesis, and Biomedical Applications, Acc. Chem. Res., 2009, 42(8), 1097–1107 CrossRef CAS PubMed
. - M. Liong, et al., Multifunctional inorganic nanoparticles for imaging, targeting, and drug delivery, ACS Nano, 2008, 2(5), 889–896 CrossRef CAS PubMed
. - J. Kim, Y. Piao and T. Hyeon, Multifunctional nanostructured materials for multimodal imaging, and simultaneous imaging and therapy, Chem. Soc. Rev., 2009, 38(2), 372–390 RSC
. - T. Neuberger, et al., Superparamagnetic nanoparticles for biomedical applications: Possibilities and limitations of a new drug delivery system, J. Magn. Magn. Mater., 2005, 293(1), 483–496 CrossRef CAS PubMed
. - M. Bruchez, et al., Semiconductor nanocrystals as fluorescent biological labels, Science, 1998, 281(5385), 2013–2016 CrossRef CAS
. - M. Y. Han, et al., Quantum-dot-tagged microbeads for multiplexed optical coding of biomolecules, Nat. Biotechnol., 2001, 19(7), 631–635 CrossRef CAS PubMed
. - X. H. Gao, et al., In vivo cancer targeting and imaging with semiconductor quantum dots, Nat. Biotechnol., 2004, 22(8), 969–976 CrossRef CAS PubMed
. - W. C. W. Chan and S. M. Nie, Quantum dot bioconjugates for ultrasensitive nonisotopic detection, Science, 1998, 281(5385), 2016–2018 CrossRef CAS
. - D. V. Talapin, et al., Synthesis and surface modification of amino-stabilized CdSe, CdTe and InP nanocrystals, Colloids Surf., A, 2002, 202(2–3), 145–154 CrossRef CAS
. - Z. D. Lu, et al., Direct Assembly of Hydrophobic Nanoparticles to Multifunctional Structures, Nano Lett., 2011, 11(8), 3404–3412 CrossRef CAS PubMed
. - D. Gerion, et al., Synthesis and properties of biocompatible water-soluble silica-coated CdSe/ZnS semiconductor quantum dots, J. Phys. Chem. B, 2001, 105(37), 8861–8871 CrossRef CAS
. - J. Kim, et al., Magnetic fluorescent delivery vehicle using uniform mesoporous silica spheres embedded with monodisperse magnetic and semiconductor nanocrystals, J. Am. Chem. Soc., 2006, 128(3), 688–689 CrossRef CAS PubMed
. - N. Ma, et al., Facile Synthesis, Silanization, and Biodistribution of Biocompatible Quantum Dots, Small, 2010, 6(14), 1520–1528 CrossRef CAS PubMed
. - P. Yang, M. Ando and N. Murase, Preparation of SiO2 beads with highly luminescent and magnetic nanocrystals via a modified reverse micelle process, New J. Chem., 2009, 33(7), 1457–1461 RSC
. - W. Park, et al., Highly photoluminescent superparamagnetic silica composites for on-site biosensors, J. Mater. Chem. B, 2014, 2(14), 1938–1944 RSC
. - H. H. Wang, et al., Synthesis and characterization of multifunctional CdTe/Fe2O3@SiO2 core/shell nanosensors for Hg2+ ions detection, New J. Chem., 2010, 34(12), 2996–3003 RSC
. - B. H. Jun, et al., Ultrasensitive, Biocompatible, Quantum-Dot-Embedded Silica Nanoparticles for Bioimaging, Adv. Funct. Mater., 2012, 22(9), 1843–1849 CrossRef CAS
. - W. Stober, A. Fink and E. Bohn, Controlled Growth of Monodisperse Silica Spheres in Micron Size Range, J. Colloid Interface Sci., 1968, 26, 62–69 CrossRef
. - W. Y. Rho, et al., Facile synthesis of monodispersed silica-coated magnetic nanoparticles, J. Ind. Eng. Chem., 2014, 20(5), 2646–2649 CrossRef CAS PubMed
. - T. R. Sathe, A. Agrawal and S. M. Nie, Mesoporous silica beads embedded with semiconductor quantum dots and iron oxide nanocrystals: Dual-function microcarriers for optical encoding and magnetic separation, Anal. Chem., 2006, 78(16), 5627–5632 CrossRef CAS PubMed
.
Footnote |
† Electronic supplementary information (ESI) available. See DOI: 10.1039/c5ra03139g |
|
This journal is © The Royal Society of Chemistry 2015 |