DOI:
10.1039/C5RA02588E
(Paper)
RSC Adv., 2015,
5, 40820-40830
One-step cross-linked injectable hydrogels with tunable properties for space-filling scaffolds in tissue engineering
Received
10th February 2015
, Accepted 29th April 2015
First published on 30th April 2015
Abstract
Developments in tissue engineering have led to the fabrication and refinement of a wide variety of injectable space-filling agents, as they can be injected to the tissue defect sites in a minimally invasive manner. Hydrogels are promising since the native tissue composed of cells and extracellular matrix can be well simulated by hydrogels encapsulating therapeutic cells and drugs. In this study, aldehyde dextran (ald-dex) and amino gelatin (ami-gel) were prepared through reaction with sodium periodate and ethane diamine to fabricate in situ gelable ald-dex/ami-gel hydrogel. The gelation process was monitored rheologically and the microstructure was revealed under scanning electron microscopy (SEM). The tunable porous structures were obtained through simply altering the ratio of aldehyde dextran and amino gelatin. Gelation time and swelling ratio of the ald-dex/ami-gel hydrogels were shown to be related to the crosslinking density of the hydrogels, which can be modulated through simply changing the proportion of ald-dex/ami-gel. The degradation rate could also be modulated by altering the content of aldehyde dextran. Then biocompatibility evaluation was performed on both two-dimensional (2D) and three-dimensional (3D) environments by using mouse pre-osteoblast cells (MC3T3-E1). According to the results, beneficial cell responses such as the attachment, spreading and proliferation of cells were exhibited with the increase of the gelatin content. Furthermore, MC3T3-E1 cells encapsulated inside the hydrogel assumed a round shape initially and gradually adapted to the microenvironment to spread out. Therefore, the ability to support cell adhesion and spreading on the surface and long-term viability of encapsulated cells enabled the tunable ald-dex/ami-gel hydrogel to be an effective space-filling scaffold for tissue engineering.
Introduction
Since the latter years of the twentieth century, when tissue engineering emerged to promote tissue regeneration, there has been a steady improvement in strategies used to engineer tissues. These approaches depend on employing a material scaffold composed of synthetic polymer or natural material, which serves as a biomimetic extracellular matrix (ECM) to organize cells into a three-dimensional architecture. Space-filling scaffolds are applied as delivery vehicles for bioactive molecules and cells, providing three-dimensional structures for tissue formation. The use of space-filling scaffolds in a wide variety of applications needs to meet a combination of functional properties to match the host tissue without deleterious response. Moreover, the promising scaffolds should be modulated simply and present stimuli directing the growth and formation of a desired tissue, equally important as their injectability being delivered to the tissue defect sites in a minimally invasive manner.
Hydrogels, similar to natural extracellular microenvironment, are highly cross-linked polymer networks and capable of absorbing large amount of water. Its great injectability and easy shaping into any three-dimensional configuration simplify the minimally invasive injection procedure. Bioactive molecules can be delivered from hydrogel scaffolds to promote angiogenesis, chondrogenesis, osteogenesis and epidermal genesis. Similarly, various cells can be encapsulated to engineer many tissue in the body, including skin, cartilage, bone, and smooth muscle. Because of exceptional promise in biomedical applications they have emerged as powerful and efficient matrices for 3D cell culture.1 Currently in situ gel-forming hydrogels have received significant attention because of their great injectability and easy gelation capacity, which provides a means of minimally invasive delivery directly into voids or tissues.2–4 Since the initial materials are often liquid solutions, the system can fit a cavity or a defect easily. What's more, various potential therapeutic agents could also be incorporated by pre-mixing. Both natural polymers and synthetic polymers have been used to fabricate in situ gelling hydrogels over the past decade. Methods of triggering gelation of precursor solutions include photopolymerization, thermosensitivity, pH-sensitivity, Schiff-based reaction, click reaction and self-assembly.5–7 Although synthetic polymers can provide promising tunable properties, their application in tissue engineering is limited by low degradation ratio and poor biocompatibility. Natural polymers originally harvested from the organs possess better biocompatibility in comparison with synthetic polymers.
Polysaccharides, as natural polymers, can biodegrade relatively fast and have gained increasing attention. Dextran is a colloidal and hydrophilic polysaccharide. It is an analog to the glucosaminoglycans found in the extracellular matrix and would therefore be able to mimic the ECM. It has been extensively investigated as an alternative for biomedical applications.8–10 The basic dextran structure is glucopyranose ring with three vicinal hydroxyl groups, which can be chemically converted into various functional groups. For example, Cohen's group has utilized the acetal-modified dextran microparticles for gene delivery in phagocytic and non-phagocytic cells.11 Equally, gelatin is a hydrolyzed protein deriving from collagen, which is the main component of natural ECM. Compared with collagen, gelatin is economical and low-antigenic. It can be manipulated to various forms such as nanoparticles and employed in drug delivery.12 Gelatin hydrogel also mimics natural extracellular matrix (ECM) and provides appropriate biological signals to cells.13 Although the gelatin has many advantages, its application in tissue engineering is limited by its inherent weakness properties. It usually works as a carrier for drug and cell delivery in tissue engineering.14 It has been modified as a scaffold for BMSCs and growth factors to repair osteochondral defects in a rabbit model and worked as a space-filling agent to repair femoral condyle defects in a rat model.15,16
The aim of this study was to develop a tunable and injectable hydrogel which was a biomimetic substrate. Compared with other injectable hydrogel natural polymers, the base materials of this hydrogel are derived from the main components of ECM. The physicochemical and biological properties can be modulated simply through changing the proportion of base materials. Gelatin bears abundant free amino groups, which can react with aldehyde groups, a convenient cross-linked method known as the “Schiff-based reaction”. And oxidizing dextran with periodate is a classic method to produces aldehyde groups serving as crosslinking points to form hydrogels. Thus, dextran was oxidized with sodium periodate to obtain aldehyde groups. Ethane diamine was used to convert carboxyl groups on gelatin chains into amino groups so as to maintain gelatin fluid at room temperature. Then the different formulated mixture solutions could be injectable and the Schiff-based reaction between aldehyde groups and amino groups cross-linked the ald-dex and ami-gel to form in situ hydrogel. The physicochemical properties of the hydrogel were elucidated by experimental analyses. We further characterized the cytotoxicity and biocompatibility of our hydrogel. Specifically, adhesion and spreading of mouse pre-osteoblast cells (MC3T3-E1) on hydrogel surface as well as the proliferation of MC3T3-E1 cells were studied. The results showed that the ald-dex/ami-gel hydrogel could be a desirable matrix for tissue engineering.
Materials and methods
Materials
Dextran (Mw 100
000), gelatin (type B, Mw 60
000), sodium periodate, ethane diamine (ED), 1-ethyl-3-(3-dimethyl aminopropyl) carbodiimide hydrochloride (EDC) were purchased from Sigma-Aldrich (St Louis, MO, USA). Mouse pre-osteoblast cells (MC3T3-E1) were obtained from the institute of Biochemistry and Cell biology (Chinese Academy of Sciences, China). Fetal bovine serum (FBS), phosphate buffered saline (PBS), Live/Dead Viability Assay Kit and other reagents were purchased from Gibco Life Technologies Corporation (Carlsbad, CA, USA).
Preparation of aldehyde dextran and amino gelatin
6.34 g of sodium periodate was dissolved in 100 ml distilled water to prepare NaIO4 solution, which was added dropwise to dextran solution (100 ml, 10% w/v) and stirred at room temperature for 6 hours. Then ethylene glycol was applied to terminate the reaction. The solution was dialyzed against ultrapure water for 3 days. The aldehyde dextran was obtained by lyophilizing the solution. Fourier Transform Infrared-Attenuated Total Reflectance (FTIR-ATR) was carried out to verify the existence of aldehyde groups. The oxidation degree was determined by quantifying the aldehyde groups reacted with tert-butyl carbazate via carbazone formation.17,18 Firstly, aldehyde dextran solution was prepared in acetate buffer (pH = 5.2) at a concentration of 10 mg ml−1. Then a fivefold excess tert-butyl carbazate in acetate buffer was added and the mixture was allowed to react for 24 h at room temperature, which is followed by addition of a fivefold excess of NaBH3CN to react for another 12 h. Then the product was precipitated thrice with acetone, dialyzed thoroughly against water and obtained by lyophilization. The oxidation degree was assessed by 1H NMR through integrating the peaks at 1.3 ppm (tert-butyl) against 4.9 ppm (dextran) (Varian 400).
Type B gelatin (5 g) was dissolved in sodium dihydrogen phosphate solution (pH = 5, 100 ml, 0.1 mol L−1) and 16 ml (0.24 mol) of ethane diamine was added. Then pH was adjusted back to 5 with hydrochloric acid (HCl) and 2.3 g of EDC was added. The reaction was allowed to proceed at room temperature overnight. Hereafter, the result mixture was dialyzed against ultrapure water to remove the excess ED and EDC. The amino group content in amino gelatin was determined by the TNBS method we described previously.19
Aldehyde dextran and amino gelatin were dissolved in phosphate buffered saline (PBS) at a concentration of 10 wt% and 20 wt%. Aldehyde dextran and amino gelatin were mixed at ratio of 3/7, 4/6, 5/5, 6/4 and 7/3 for gel forming.
Rheological evaluation
To characterize the real-time change in mechanical properties of the cross-linked hydrogel, the shear elastic modulus was measured via dynamic oscillatory shear rheometry with parallel-plate geometry (20 mm diameter) at a frequency of 1 rad s−1 and a shear strain of 2% at a constant temperature of 37 °C using a Haake RS 150L Rheometer (Thermo Haake Co., Germany). Initial gel network formation of different groups were monitored by observing elastic, G′, storage, G′′, moduli, and viscosity (η).
Scanning electron microscope (SEM) analysis
The morphology of freeze-dried hydrogels was observed using a SEM (JSM-5600, Japan) at an accelerated voltage of 10 KV. Hydrogels were flash-frozen using liquid nitrogen and lyophilized. The samples were sputter-coated with gold for 120 seconds before observed. Pore sizes of the lyophilized scaffolds were measured from SEM micrographs using image analysis software (Image-J, National Institutes of Health, USA).
Equilibrium swelling ratio
For the swelling studies, hydrogel samples were immersed in PBS and incubated at 37 °C for 24 h. Then, the samples were removed from the PBS and weighed accurately after wipe-dry. Then the samples were frozen at −80 °C and dried to obtain the dry hydrogel weight. The swelling ratio was calculated as the ratio of the swollen hydrogel mass to the dry hydrogel mass. At least three specimens for each sample were measured.
Hydrolytic degradation
Hydrolytic degradation were assessed at different time points (day 0, day 7, day 14, day 21, day 28). Firstly, the hydrogel samples were completely lyophilized to weigh the initial mass. Then the samples were totally submerged in PBS with 0.02% NaN3 at 37 °C for degradation and the PBS was changed per day. At each point, the samples were removed, freeze dried and then weighed to record the weight of the residuals. The weight loss at specific day point was calculated as the ratio of the remaining dry mass to the initial dry mass. All samples were tested in triplicate.
Cell adhesion and spreading on hydrogel surfaces
Aldehyde dextran and amino gelatin were mixed at ratio of 3/7, 4/6, 5/5, 6/4 and 7/3 and injected into the 24-well tissue culture plates for gel forming. MC3T3-E1 cells suspension in complete medium was prepared at a density of 5 × 104 cells per ml. Then cells were seeded on each sample for 1 ml and cultured at 37 °C in a humidified atmosphere of 5% CO2. The complete medium was replaced daily. After 3 days the cells were stained for viability using a Live/Dead Viability/Cytotoxicity Assay Kit. All groups were observed using a fluorescence microscope. The mean cell spreading areas were estimated using image analysis software (Image-J, National Institutes of Health, USA) and calculated by selecting 100 cells randomly observed on the fluorescence images. The cell morphology was classified into three categories: round, partially spread, or fully spread. Cells with spreading area <10
000 pixels2 were defined as round. Cells with areas between 10
000 and 20
000 pixels2 having few cytoplasmic extensions but were predominately round in appearance were classified as partially spread, and cells with surface areas >20
000 pixels2 were classified as fully spread and had multiple cytoplasmic extensions in different directions.
Cells viability and proliferation inside the hydrogels
After mixing aldehyde dextran and amino gelatin at the ratio of 5
:
5, the MC3T3-E1 cells were suspended at a density of 1 × 106 cells ml−1 and the mixture was injected into the 24-well plates. After gelation complete medium was added and the cell-laden hydrogel was incubated in 37 °C 5% CO2 incubator. The complete medium was replaced daily. MC3T3-E1 cells inside the hydrogel at day 1, day 3, day 7 and day 14 were stained by Live/Dead Viability/Cytotoxicity Assay Kit. Then the samples were observed using a QCapture 5 Imaging System through fluorescent microscope.
The cell proliferation inside the hydrogels in vitro culture for day 1, day 3, day 7, and day 14 was determined by the MTT (3-[4,5-dimethylthiazol-2-yl]-2,5-diphenyltetrazolium bromide) assay. In brief, 0.5 mg ml−1 MTT solution in serum-free medium was added to each well containing the cell-laden hydrogel. Active mitochondria metabolize the tetrazolium salt to form an insoluble formazan salt. After 6 hours of incubation at 37 °C, the solution was removed and DMSO was added to extract the formazan salts. The absorbance was measured at 490 nm with an Enzyme-labeled Instrument (MK3, Thermo, USA).
Results
Characterization of aldehyde dextran and amino gelatin
Dextran was oxidized by sodium periodate through a complicated two-step reaction as illustrated in Fig. 1A. In the first step, the adjacent hydroxyl groups of glucopyranose ring were oxidized generating two aldehyde groups. Since the aldehyde in position C3 has a vicinal hydroxyl group, it was further oxidized to form formic acid and a dialdehyde group. Dextran was oxidized by an excess of sodium periodate and after 6 hours incubation at room temperature, the excess of unreacted periodate was removed by adding ethylene glycol. After 3 days dialysis against ultrapure water to remove the formic acid, the reagent was lyophilized to obtain aldehyde dextran. For confirming the oxidation reaction of dextran, the FTIR spectrum (Fig. 1B) was used. We found that dialdehyde absorption peak (1733 cm−1) which associate with the C
O group appeared in the IR curve of aldehyde dextran. In the 1H NMR spectra of dextran and aldehyde dextran reacted with tert-butyl carbazate (Fig. 1C), the solvent (HOD) proton resonated at 4.67 ppm. The peaks at 3.4–4.0 ppm were assigned to protons at positions C2, C3, C4, C5 and C6. The anomeric proton from the glucopyranose ring of dextran with α-1, 6 linkages was found at δ 4.9 ppm while the peak at δ 5.3 ppm was attributed to the anomeric proton in dextran with α-1, 3 linkages.17,20–22 The signal at δ 1.3 ppm was assigned to the proton of the tert-butyl group. The oxidation degree was calculated as the ratio of integrals of the peak at 1.3 ppm (tert-butyl) to 4.9 ppm (dextran), which was approximately 41%. Generally, Type B gelatin maintains a solid state at room temperature (Fig. 2A). By altering carboxyl groups to amino groups through the reaction with ethane diamine (Fig. 2D), the intermolecular forces between amino and carboxyl groups would be weaken. As a consequence, amino gelatin with different concentrations shows obviously lower sol–gel transition temperature than gelatin (Fig. 2C). Amino gelatin at concentration of 20 wt% stays fluid at room temperature and can be injected to the tissue defect sites. The amino content of amino gelatin was determined as approximately 0.802 mmol g−1 by the TNBS method.
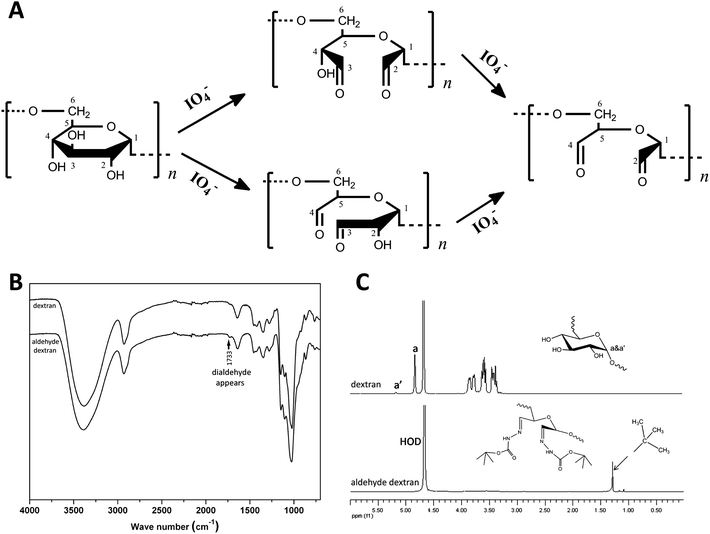 |
| Fig. 1 (A) the corresponding reaction of preparing aldehyde dextran; (B) FTIR spectra of dextran and aldehyde dextran; (C) 1H NMR spectra of dextran and aldehyde dextran treated with tert-butyl carbazate. | |
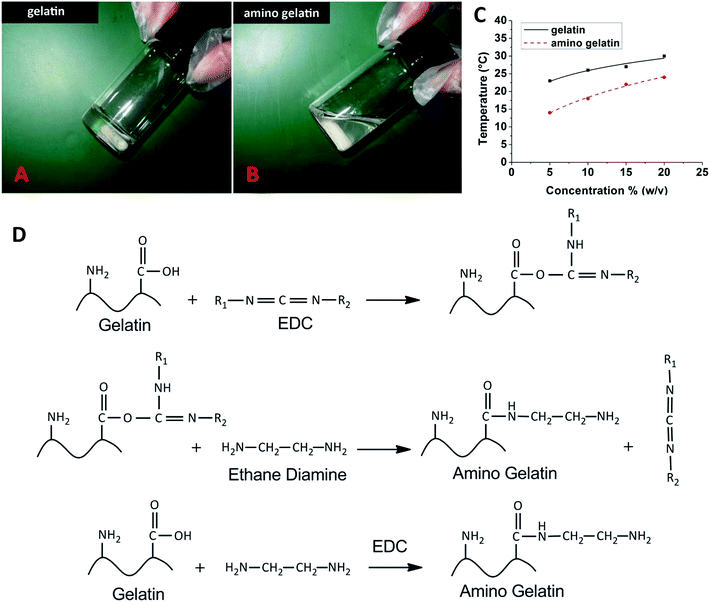 |
| Fig. 2 Illustration of the state of gelatin (A) and amino gelatin (B); (C) sol–gel transition temperature of gelatin and amino gelatin; (D) the corresponding reaction of preparing amino gelatin. | |
Gel formation and gelation time of aldehyde dextran and amino gelatin
Gelation occurred quickly after mixing the two components (Fig. 3A). As shown in Fig. 3B, storage modulus (G′) and loss modulus (G′′) were at a very low level in the beginning. G′ was lower than G′′ and the mixture system exhibited the behavior of a viscous fluid. With the proceeding of Schiff-based reaction between aldehyde groups and amino groups, both moduli increased quickly and the solutions begin to crosslink. Due to the higher growing rate of G′ than that of G′′, there was a crossover point normally considered as “gel point” (tgel). It indicated that the ald-dex/ami-gel system transformed from a viscous behavior dominated liquid-phase to an elastic behavior dominated solid-phase. At last both moduli leveled off, implying the cross-linking process ended and the three-dimensional network was formed. η* of the system also increased rapidly at the beginning and leveled off eventually. From the insert graph we can see that tgel of ald-dex/ami-gel (ratio of 5
:
5) hydrogel was approximately 95 s.
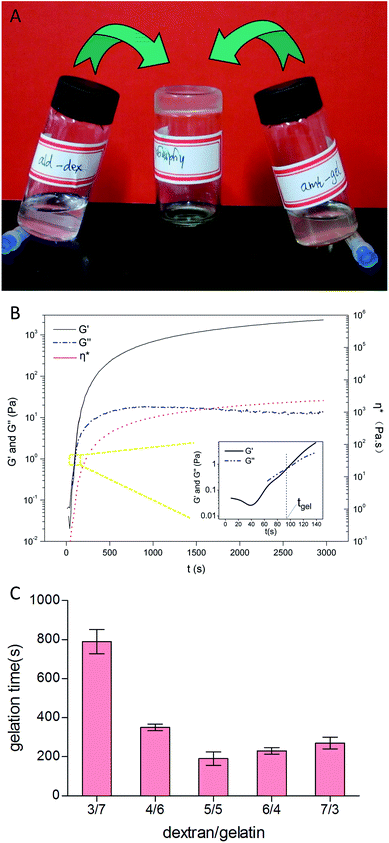 |
| Fig. 3 (A) Gelation occurred quickly after mixing the two components; (B) time sweep of storage modulus (G′) and loss modulus (G′′) for ald-dex/ami-gel (5/5) hydrogels at 37 °C; (C) different gelation time of hydrogels obtained by altering the ratio of dextran and gelatin. | |
In the tube tilting test, conspicuous difference was found in gelation time when changing the blending ratio of aldehyde dextran and amino gelatin (Fig. 3C). With the increase of ald-dex's content, the gelation time decreased dramatically and then increased slightly. The 5/5 hydrogel had the shortest gelation time due to the fact that the highest rate of Schiff-base crosslink formation occurred at the ratio of 5/5. In the ald-dex/ami-gel mixture system, ald-dex served as a macromolecular crosslinker for ami-gel bearing free amino groups to form hydrogels. Due to the increasing content of crosslinker, Schiff-base crosslink formed at an increasing rate and finally reached at a steady rate. So the gelation time decreased dramatically from 3/7 to 5/5 and then there is no decrease among the hydrogels at 5/5, 6/4, and 7/3. Furthermore, there is slight increase in the gelation time among the hydrogels at 5/5, 6/4, and 7/3 on account of the lower content of ami-gel. Thus, the gelation speed could be modulated by adjusting the proportion of ald-dex and ami-gel.
Morphology of hydrogels and swelling analyses
From gross observation, the freeze-dried ald-dex/ami-gel hydrogel was porous and yellowish in color (Fig. 4A). The flash-frozen technique, which can better preserve the morphology, has been used to obtain a good manifestation of microstructural changes among different hydrogel samples. All samples possessed interconnected and homogeneously distributed pores, but with obviously different pore sizes (Fig. 4). Hydrogels with different ratio of ald-dex/ami-gel at 3/7, 4/6, 5/5, 6/4, 7/3 exhibited a pore size of 221.7 ± 26.42 μm, 182.9 ± 19.57 μm, 109.9 ± 20.71 μm, 129.1 ± 22.64 μm, 139.3 ± 21.36 μm. 5/5 hydrogel had the smallest pore size of 109.9 ± 20.71 μm and was more compact than other hydrogels. The high interconnected structure is necessary for scaffolds to retain tissue fluid and possess high oxygen and nutrient permeability. Therefore, the hydrogels might be suitable for cell delivery in tissue engineering.
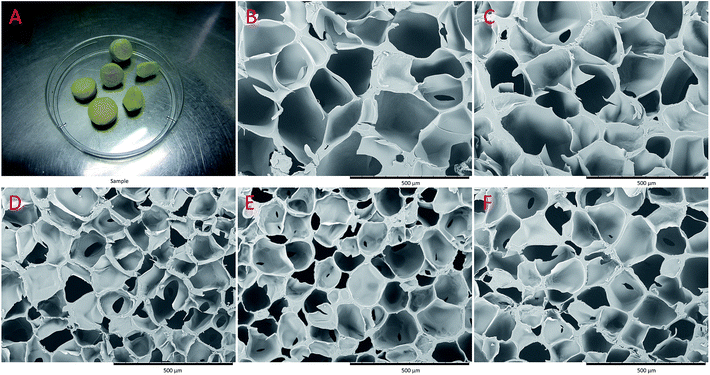 |
| Fig. 4 Morphology of different formulated hydrogels measured by lyophilization. (A) Gross observation of the freeze-dried ald-dex/ami-gel hydrogel; (B)–(F) showed the SEM micrographs of freeze-dried hydrogels at the ratio of 3/7, 4/6, 5/5, 6/4 and 7/3. | |
Fig. 5A indicated the equilibrium swelling ratio of freeze-dried hydrogels and revealed the relationship between the swelling ratio and the dextran's content. First, the swelling ratio decreased rapidly with the increase of dextran's content (3/7 to 5/5). Thereafter, with the dextran's content increased (5/5 to 7/3), the swelling ratio increased slightly. This can be ascribed to the variation of crosslinking degree in the hydrogels and distinct water take-up capacity of dextran and gelatin. When the aldehyde dextran and amino gelatin were mixed at the ratio pf 5/5, the crosslinking density reached its maximum and thus the swelling ratio got its minimum value. A further increase in aldehyde dextran or amino gelatin resulted in an excess of aldehyde groups or amino groups, which led to a lower crosslinking degree and a higher swelling ratio. Due to the stronger capacity of gelatin to hold water compared with dextran, the increase in swelling ratio is slight with more dextran incorporated while that is obvious with more gelatin incorporated. In this regard, the variation of the swelling ratio corresponds well with the results of the gelation time and pore sizes of hydrogels depicted in Fig. 3C and 4.
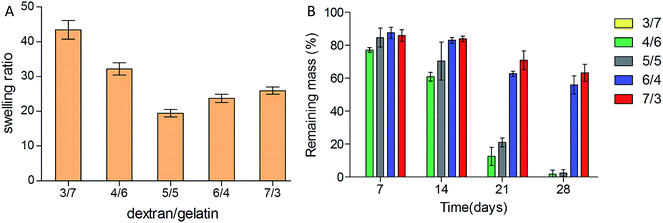 |
| Fig. 5 (A) Swelling ratios of different formulated hydrogels after immersing into PBS for 24 h; (B) degradation property of different formulated hydrogels at different time points. | |
Degradation of hydrogels
Fig. 5B indicated the degradation of different formulated hydrogels and revealed the relationship between degradation and the dextran's content. The hydrogel at 7/3 ratio exhibited the lowest degradation rate compared with other formulated hydrogels. Its remaining mass was approximately 60% of the initial dry weight at 28 days. By simply altering the ratio of constituents various degradation property could obtained. Through decreasing dextran's content, the hydrogel possessed a higher degradation behavior and almost fully degraded after 28 days at the ratio of 5/5 and 4/6. The 3/7 hydrogel exhibited the highest degradation profile (totally degrade at 7 days, which is not shown in Fig. 5B). Not only the crosslinking degree in the hydrogel but also the monomer concentration, influence the erosion profile and the overall time for degradation.23 It seems obvious that the higher crosslinking density due to the higher content of oxidized dextran results in lower degradation rate. During the degradation process more cross-links require more time to be hydrolyzed. On the other hand, it seems that a fraction of unbound molecules was physically entrapped in the matrix at the time of gelation. From 3/7 to 7/3, nonpolymerized monomer in the ald-dex/ami-gel hydrogel transferred mainly from amino gelatin to aldehyde dextran. Due to the easier hydrolyzation of amino gelatin than aldehyde dextran in aqueous environment, the mass losses rate slows down from 3/7 to 7/3. When the crosslinks are hydrolyzed, the hydrogel network becomes looser, imbibing more water and leading to further hydrolysis.24 A severe mass loss occurs over time, culminating in the complete dissolution of the gels. Thus, after 28 days the remaining mass of hydrogels at the 4/6 and 5/5 ratio is much smaller than that at the 6/4 and 7/3 ratio. As a consequence, the degradation rate of the ald-dex/ami-gel hydrogels could be modulated by altering the dextran's content of hydrogel to synchronize the degradation rate with cellular growth and extracellular matrix accumulation.
Cytotoxicity measurement
In this study, MC3T3-E1 cells varied greatly in their ability to adhere, spread and survive with the changing of the ald-dex and ami-gel ratio (Fig. 6). With the ratio of ald-dex/ami-gel change from 3/7 to 7/3, the matrix became adverse to cell responses such as the attachment, spreading and survival of cells. For groups of 6/4 and 7/3, even dead cells emerged. With the decrease of gelatin's content, the mean spreading areas gradually decreased from 29
044.65 ± 8281.80 pixels2 to 13
594.48 ± 6997.20 pixels2 and the percentage of spread cells decreased from 87% to 22%. Poor attachment property was exhibited in the groups (6/4 and 7/3). Meanwhile, 22% and 33% cells remained round and less than 50% cells spread for groups (6/4 and 7/3). Accordingly, when the gelatin increased to 50% or higher than 50%, there were rarely round cells. One possible explanation is that the toxicity caused by the aldehyde groups in aldehyde dextran hampered the adhesion of cells on matrix surface. Most tissue-derived cells require attachment and spreading on a solid surface for viability, growth, migration and differentiation. So the remaining of round cells and poor attachment (6/4 and 7/3) was consistent with the emergence of dead cells.
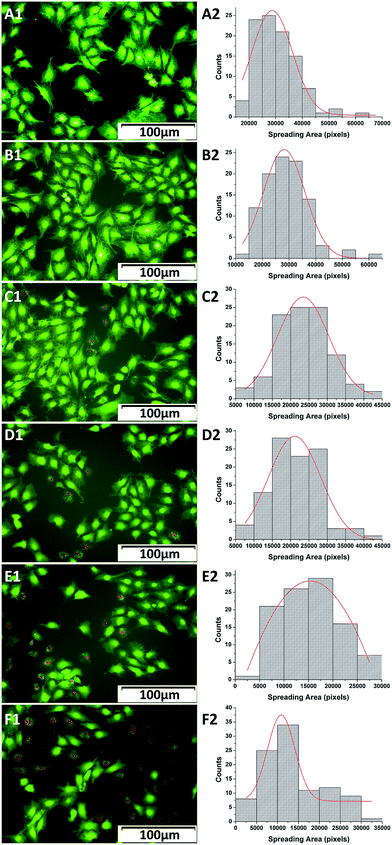 |
| Fig. 6 MC3T3-E1 cells cultured on tissue culture plate (A) and different formulated hydrogels ((B) 3/7; (C) 4/6; (D) 5/5; (E) 6/4; (F) 7/3) at 3 days. Live cells were stained green, while dead cells were red. Scale bar represents 100 μm. | |
Viability of encapsulated cells in hydrogels
When employing ald-dex/ami-gel hydrogels (5/5) as extracellular matrix mimics, it is necessary to assess the growth of cells within the hydrogel. At day 1 there were only round cells in the hydrogels (Fig. 7A2). After 3 days of culture (Fig. 7B2), some cells had spread and the extent of spreading of individual cells was increased over time. At day 7 and day 14, clear evidence could be found that more cells have a spread morphology compared with day 3 (Fig. 7C2 and D2). This may be caused by the formation of extension space to allow cells to spread and proliferate with the degradation of ald-dex/ami-gel hydrogels.
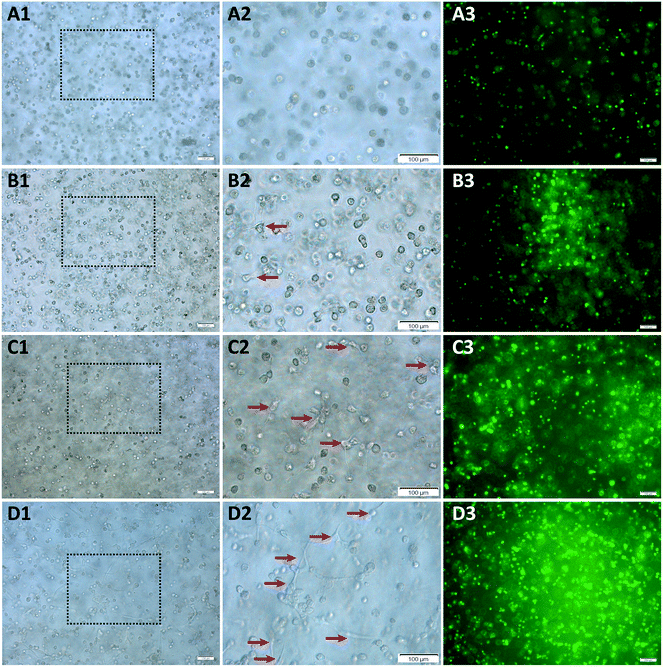 |
| Fig. 7 MC3T3-E1 cells encapsulated in ald-dex/ami-gel (5/5) hydrogels at different time points ((A) day 1; (B) day 3; (C) day 7; (D) day 14). Live cells were stained green, while dead cells were red. Scale bar represents 100 μm. | |
The viability of MC3T3-E1 cells in hydrogels was stained by Live/Dead Viability Assay Kit as shown in Fig. 7. After 1 day and 3 days of culture, the overwhelming majority of encapsulated cells was alive and stained as green. At day 7 and day 14, there were also rarely dead cells stained as red indicating that the ald-dex/ami-gel hydrogels possess a good biocompatibility. The number of viable cells in samples increased with culturing time. Most of the living MC3T3-E1 cells were homogeneously encapsulated within the hydrogels after culture. In addition, MTT tests were performed to quantitatively assess the proliferation of MC3T3-E1 cells encapsulated in ald-dex/ami-gel hydrogels at 1, 3, 7 and 14 days (Fig. 8). During the entire experimental period, cells inside hydrogels displayed a significant increased viability profile. The results of MTT were consistent with the increasing cell number stained by Live/Dead Viability Assay Kit. There was significant difference in optical density for ald-dex/ami-gel hydrogels among 1, 3, 7 and 14 days. The optical density obviously increased with culturing time, further indicating that the ald-dex/ami-gel hydrogels have no toxicity on cell viability.
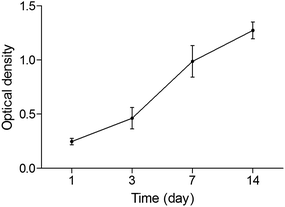 |
| Fig. 8 MTT assay of encapsulated cells at different time points (1, 3, 7, 14 days). | |
Discussion
Developments in tissue engineering have led to a large demand for a variety of injectable space-filling agents, as they can be injected to the tissue defect sites in a minimally invasive manner. In this study, injectable hydrogels were synthesized from aldehyde dextran and amino gelatin through Schiff-based reaction. Firstly, the dextran molecule is a polymer formed by glycosidic bonds linking the carbon atoms (C1 and C6) of consecutive α-D-glucopyranose rings. The dextran monomer has two sites that are cleaved by periodate oxidation (C2–C3 and C3–C4), breaking the structure of the glucopyranose ring. So the oxidation reaction cleaved glucopyranose ring and resulted in the increasing of cross-linking aldehyde groups. Then the amination reaction altered carboxyl groups into amino groups in gelatin, leading to the increasing of cross-linking amino groups. These allowed for the formation of Schiff-based reaction between aldehyde groups in aldehyde dextran and amino groups in amino gelatin. Moreover, carboxyl groups of gelatin were changed into amino groups leading to the decrease in intrinsic viscosity. As a result, the ald-dex and ami-gel are readily available in aqueous solution. They are injectable into irregular-shaped defects and can be easily contoured to the dimensions of the defects.
In the selection of hydrogels to be used in tissue engineering, the first consideration should be fitness for purpose with regard to characteristics and properties of the biomaterials. These include chemical, toxicological, physical and morphological properties. Five formulated hydrogels with different ratios (3/7, 4/6, 5/5, 6/4, 7/3) were prepared. Since rapid gelation is a prerequisite for minimally invasive procedure, we focused on the gelation time of the ald-dex/ami-gel space-filling systems. For the aldehyde dextran and amino gelatin system, mixing 10 w/v% ald-dex and 20 w/v% ami-gel solutions at volume ratio of 5/5 was found to be fastest to form hydrogel. This is ascribed to the higher cross-linking density than other formulated samples, which can be revealed in the SEM morphology of hydrogels.
Due to large water content, hydrogels have tissue-like properties, allowing them to encapsulate cells and give appropriate structural growth cues to cells. High water-absorbency may facilitate maintaining significant amounts of tissue fluid and nutrients for tissue regeneration. In this study, a decrease in swelling with an increasing ald-dex/ami-gel ratio from 3/7 to 5/5 is due to increased crosslinking density. The 5/5 hydrogel has the highest crosslinking density and lowest swelling ratio, which is implied by gelation time. This is in agreement with a reported dependency of swelling ratio on the aldehyde dextran's content of a hydrogel containing N-carboxyethyl chitosan.18 On the other hand, the correlation between the swelling ratio of lyophilized hydrogels and crosslinking density is also in agreement with the literature.25 A high degree of swelling implies limited crosslinking density and is consistent with the looser structure. Obviously, ald-dex/ami-gel (3/7) hydrogel had a porous structure with larger pores than others. The looser network might result in a higher swelling ratio compared with others.
The surface properties of the hydrogels also play an important role because the unfavorable interaction between hydrogel and the surrounding tissue firstly occurs at the interface. The scaffold surface should be designed to enhance beneficial cell responses such as the attachment, spreading and proliferation. In this study, the increase of gelatin elevated the percentage of spread cells and contributed to cell viability. Gelatin is a partially hydrolyzed form of collagen, which is the most abundant protein found in the ECM. It contains Asp-Gly-Arg-like sequences that have been shown to improve cell attachment. Modulation of the biologic activity of gelatin has been shown in other studies in which the gelatin can mediate attachment and spreading of cells on many surfaces and enhance cell growth.26–28 The addition of gelatin could greatly promote cell spreading and proliferation in 3-D cell culture, which gave us the thought that by using gelatin as the major section of the scaffold, a distinct matrix for tissue engineering could be obtained. So the cell responses on the surface of ald-dex/ami-gel hydrogels can be varied by gelatin content. Good surface biocompatibility can be achieved and cells can migrate more easily from the surrounding tissue into the hydrogels.
Hydrogels are promising since the native tissue composed of cells and extracellular matrix can be well simulated by hydrogels encapsulating therapeutic cells and drugs.29 Various biomolecules could be incorporated to functionalize hydrogels and control cell adhesion, proliferation and extension. The key property of a useful hydrogel is the growth of cells within the hydrogel. After 14 days of culture, there were rarely dead cells within the hydrogels (5/5) indicating that the ald-dex/ami-gel hydrogels are suitable as carriers for cell delivery in tissue engineering. It is promising that cells can migrate from the surrounding tissue into the ald-dex/ami-gel hydrogels and proliferate within the hydrogels, which produce new tissue with appropriate structure and properties, thus provide a natural repair that can later become integrated with the patient's tissues.
Conclusion
Aldehyde dextran and amino gelatin were prepared to fabricate a tunable injectable hydrogel. The physical properties including rheological, swelling ratio, gelling time, degradation rate, and morphology of the lyophilized hydrogels could be modulated through simply changing the proportion of ald-dex and ami-gel. Surface adhesion and spreading of cells have also been testified by using MC3T3-E1 cells. Beneficial cell responses such as the attachment, spreading and proliferation of cells are exhibited with the increase of amino gelatin's content. Furthermore, MC3T3-E1 cells encapsulation demonstrated that the ald-dex/ami-gel hydrogel can sustain cell spreading and promoted cell proliferation in vitro.
Acknowledgements
This work was financially supported by the National Natural Science Foundation of China (Contract Grant no. 81171671, no. 81371966, no. 2014ZYJB0302).
References
- M. W. Tibbitt and K. S. Anseth, Hydrogels as Extracellular Matrix Mimics for 3D Cell Culture, Biotechnol. Bioeng., 2009, 103, 655–663 CrossRef CAS PubMed
. - L. Yu and J. D. Ding, Injectable hydrogels as unique biomedical materials, Chem. Soc. Rev., 2008, 37, 1473–1481 RSC
. - H. Park, J. S. Temenoff, Y. Tabata, A. I. Caplan and A. G. Mikos, Injectable biodegradable hydrogel composites for rabbit marrow mesenchymal stem cell and growth factor delivery for cartilage tissue engineering, Biomaterials, 2007, 28, 3217–3227 CrossRef CAS PubMed
. - R. Mateen and T. Hoare, Injectable, in situ gelling, cyclodextrin-dextran hydrogels for the partitioning-driven release of hydrophobic drugs, J. Mater. Chem. B, 2014, 2, 5157–5167 RSC
. - W. E. Hennink and C. F. van Nostrum, Novel crosslinking methods to design hydrogels, Adv. Drug Delivery Rev., 2012, 64, 223–236 CrossRef PubMed
. - J. J. Pillai, A. K. T. Thulasidasan, R. J. Anto, D. N. Chithralekha, A. Narayanan and G. S. V. Kumar, Folic acid conjugated cross-linked acrylic polymer (FA-CLAP) hydrogel for site specific delivery of hydrophobic drugs to cancer cells, J. Nanobiotechnol., 2014, 12, 25 CrossRef PubMed
. - A. Prasannan, H. C. Tsai, Y. S. Chen and G. H. Hsiue, A thermally triggered in situ hydrogel from poly(acrylic acid-co-N-isopropylacrylamide) for controlled release of anti-glaucoma drugs, J. Mater. Chem. B, 2014, 2, 1988–1997 RSC
. - G. M. Sun, X. J. Zhang, Y. I. Shen, R. Sebastian, L. E. Dickinson, K. Fox-Talbot, M. Reinblatt, C. Steenbergen, J. W. Harmon and S. Gerecht, Dextran hydrogel scaffolds enhance angiogenic responses and promote complete skin regeneration during burn wound healing, Proc. Natl. Acad. Sci. U. S. A., 2011, 108, 20976–20981 CrossRef CAS PubMed
. - L. Pescosolido, W. Schuurman, J. Malda, P. Matricardi, F. Alhaique, T. Coviello, P. R. van Weeren, W. J. A. Dhert, W. E. Hennink and T. Vermonden, Hyaluronic Acid and Dextran-Based Semi-IPN Hydrogels as Biomaterials for Bioprinting, Biomacromolecules, 2011, 12, 1831–1838 CrossRef CAS PubMed
. - L. S. M. Teixeira, S. Bijl, V. V. Pully, C. Otto, J. Rong, J. Feijen, C. A. van Blitterswijk, P. J. Dijkstra and M. Karperien, Self-attaching and cell-attracting in-situ forming dextran-tyramine conjugates hydrogels for arthroscopic cartilage repair, Biomaterials, 2012, 33, 3164–3174 CrossRef PubMed
. - J. A. Cohen, T. T. Beaudette, J. L. Cohen, K. E. Brooders, E. M. Bachelder and J. M. J. Frechet, Acetal-Modified Dextran Microparticles with Controlled Degradation Kinetics and Surface Functionality for Gene Delivery in Phagocytic and Non-Phagocytic Cells, Adv. Mater., 2010, 22, 3593–3597 CrossRef CAS PubMed
. - J. T. Long, T. Y. Cheang, S. Y. Zhuo, R. F. Zeng, Q. S. Dai, H. P. Li and S. Fang, Anticancer drug-loaded multifunctional nanoparticles to enhance the chemotherapeutic efficacy in lung cancer metastasis, J. Nanobiotechnol., 2014, 12, 37 CrossRef PubMed
. - M. Azami, M. J. Moosavifar, N. Baheiraei, F. Moztarzadeh and J. Ai, Preparation of a biomimetic nanocomposite scaffold for bone tissue engineering via mineralization of gelatin hydrogel and study of mineral transformation in simulated body fluid, J. Biomed. Mater. Res., Part A, 2012, 100, 1347–1355 CrossRef PubMed
. - M. Santoro, A. M. Tatara and A. G. Mikos, Gelatin carriers for drug and cell delivery in tissue engineering, J. Controlled Release, 2014, 190, 210–218 CrossRef CAS PubMed
. - T. Mazaki, Y. Shiozaki, K. Yamane, A. Yoshida, M. Nakamura, Y. Yoshida, D. Zhou, T. Kitajima, M. Tanaka and Y. Ito, et al., A novel, visible light-induced, rapidly cross-linkable gelatin scaffold for osteochondral tissue engineering, Sci. Rep., 2014, 4, 4457 Search PubMed
. - H. N. Wang, Q. Zou, O. C. Boerman, A. W. G. Nijhuis, J. A. Jansen, Y. B. Li and S. C. G. Leeuwenburgh, Combined delivery of BMP-2 and bFGF from nanostructured colloidal gelatin gels and its effect on bone regeneration in vivo, J. Controlled Release, 2013, 166, 172–181 CrossRef CAS PubMed
. - J. Maia, L. Ferreira, R. Carvalho, M. A. Ramos and M. H. Gil, Synthesis and characterization of new injectable and degradable dextran-based hydrogels, Polymer, 2005, 46, 9604–9614 CrossRef CAS PubMed
. - L. Weng, A. Romanov, J. Rooney and W. Chen, Non-cytotoxic, in situ gelable hydrogels composed of N-carboxyethyl chitosan and oxidized dextran, Biomaterials, 2008, 29, 3905–3913 CrossRef CAS PubMed
. - X. H. Geng, X. M. Mo, L. P. Fan, A. L. Yin and J. Fang, Hierarchically designed injectable hydrogel from oxidized dextran, amino gelatin and 4-arm poly(ethylene glycol)-acrylate for tissue engineering application, J. Mater. Chem., 2012, 22, 25130–25139 RSC
. - R. J. H. Stenekes, H. Talsma and W. E. Hennink, Formation of dextran hydrogels by crystallization, Biomaterials, 2001, 22, 1891–1898 CrossRef CAS
. - S. G. Levesque, R. M. Lim and M. S. Shoichet, Macroporous interconnected dextran scaffolds of controlled porosity for tissue-engineering applications, Biomaterials, 2005, 26, 7436–7446 CrossRef CAS PubMed
. - J. Maia, M. P. Ribeiro, C. Ventura, R. A. Carvalho, I. J. Correia and M. H. Gil, Ocular injectable formulation assessment for oxidized dextran-based hydrogels, Acta Biomater., 2009, 5, 1948–1955 CrossRef CAS PubMed
. - J. Carvalho, S. Moreira, J. Maia and F. M. Gama, Characterization of dextrin-based hydrogels: rheology, biocompatibility, and degradation, J. Biomed. Mater. Res., Part A, 2010, 93, 389–399 Search PubMed
. - M. Molinos, V. Carvalho, D. M. Silva and F. M. Gama, Development of a Hybrid Dextrin Hydrogel Encapsulating Dextrin Nanogel as Protein Delivery System, Biomacromolecules, 2012, 13, 517–527 CrossRef CAS PubMed
. - S. M. Vickers, T. Gotterbarm and M. Spector, Cross-Linking Affects Cellular Condensation and Chondrogenesis in Type II Collagen-GAG Scaffolds Seeded with Bone Marrow-Derived Mesenchymal Stem Cells, J. Orthop. Res., 2010, 28, 1184–1192 CrossRef CAS PubMed
. - Z. W. Ma, W. He, T. Yong and S. Ramakrishna, Grafting of gelatin on electrospun poly(caprolactone) nanofibers to improve endothelial cell spreading and proliferation and to control cell orientation, Tissue Eng., 2005, 11, 1149–1158 CrossRef CAS PubMed
. - H. W. Kim, H. S. Yu and H. H. Lee, Nanofibrous matrices of poly(lactic acid) and gelatin polymeric blends for the improvement of cellular responses, J. Biomed. Mater. Res., Part A, 2008, 87, 25–32 CrossRef PubMed
. - Y. X. Liu and M. B. Chan-Park, Hydrogel based on interpenetrating polymer networks of dextran and gelatin for vascular tissue engineering, Biomaterials, 2009, 30, 196–207 CrossRef PubMed
. - D. Seliktar, Designing Cell-compatible Hydrogels for Biomedical Applications, Science, 2012, 336, 1124–1128 CrossRef CAS PubMed
.
Footnote |
† Jian-feng Pan, Heng-feng Yuan and Chang-an Guo contributed equally to this work. |
|
This journal is © The Royal Society of Chemistry 2015 |
Click here to see how this site uses Cookies. View our privacy policy here.