DOI:
10.1039/C5RA01835H
(Paper)
RSC Adv., 2015,
5, 35317-35324
Facile synthesis of CuS mesostructures with high photothermal conversion efficiency
Received
30th January 2015
, Accepted 13th April 2015
First published on 13th April 2015
Abstract
Photothermal ablation (PTA) therapy has received much attention during the past few years as a minimally invasive alternative to conventional therapeutic approaches. Among others, the semiconductor copper sulfide (CuS) is a developing and promising photothermal agent for potential cancer therapy. In the present work, CuS mesostructures with high photothermal conversion efficiency have been synthesized by assembling CuS nanoparticles with opposite charges in the aqueous phase via electrostatic interaction and then calcination at elevated temperature. Specifically, 3-mercaptopropionic acid (MPA)- and 3-mercaptopropylamine (MPa)-capping CuS nanoparticles were synthesized respectively as oppositely charged precursors, which were then assembled and calcinated to produce CuS mesostructures. The resultant CuS mesostructures have well-ordered honeycomb-like structures with high specific surface area, and can be readily heated by a 980 nm laser and are capable of killing cancer cells efficiently by the photothermal effect.
1 Introduction
In recent years, copper sulfide (CuS) nanostructured materials have been developed as a promising platform for imaging and sensing,1,2 drug delivery,3 photothermal therapy,4–7 etc. Among these functions, CuS photothermal agents are attracting increasing interest as they are a good alternative for photothermal ablation in cancer therapy. Owing to relative abundance of the component elements, intrinsic NIR-band absorption and low cytotoxicity, CuS nanomaterials in a variety of forms have been fabricated for the photothermal ablation purpose, including nanoparticles,8 flower-like superstructures,9 nanorods,10 nanocomposites,11,12 etc. These nanomaterials will be heated, subjected to near-infrared (NIR) irradiation, which derives from the d–d energy band transition of Cu2+ ions.10
Three-dimensional mesoporous materials have been extensively studied due to their high specific surface area, uniform pore structure and tailorable surface functionalities for potential applications including adsorption, storage, transportation, drug delivery, catalysis, etc.13–17 Usually, template-assisted methods are used to build mesoscale structures, in which soft templates (e.g., surfactants, polymers)18,19 and hard templates (mesoporous carbon or silica)20,21 are adopted and play a critical role in directing the formation of mesostructures. In recent years, template-free strategies have been reported for synthesizing mesoporous materials,22,23 providing new techniques for fabrication of mesostructures. Nevertheless, organic solvents are needed in these synthetic strategies, which are less favorable for biomedical applications.
To date, studies of mesoporous CuS are rarely reported, likely due to the difficulty in CuS processing. If mesoporous CuS with photothermal properties is fabricated, it is expected to have high photothermal conversion efficiency, as the honeycomb-like mesostructures favor the absorption of NIR energy. Herein we report a facile synthesis of CuS mesostructures that was achieved by assembly of oppositely-charged CuS nanoparticles (NPs) in aqueous phase and subsequent calcination at an appropriate temperature. Specifically, aqueous synthesis of 3-mercaptopropionic acid (MPA)-capping CuS NPs and 3-mercaptopropylamine (MPa)-capping CuS NPs were first carried out, respectively. The two types of CuS NPs are oppositely charged precursors, prone to assemble in aqueous phase via electrostatic interaction between the ionized carboxyls and the protonated aminos. The resultant CuS aggregates were then calcinated to produce mesoporous CuS structures (Scheme 1). The CuS mesostructures composed of densely packed CuS NPs exhibit excellent photothermal performance and can kill cancer cells in vitro efficiently under irradiation of 980 nm NIR laser.
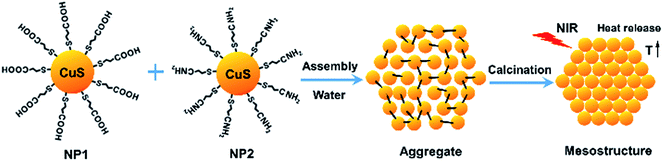 |
| Scheme 1 Schematic illustration of the synthetic procedure of CuS mesostructures. | |
2 Experimental
2.1 Materials
Copper chloride (CuCl2·2H2O, 99%), sodium sulfide (Na2S, 98%), 3-mercaptopropionic acid (MPA, 99%), 3-mercaptopropylamine (MPa, 99%), RPMI 1640 cell culture medium and 3-(4,5-dimethylthiazole-2-yl)-2,5-diphenyltetrazolium chloride (MTT) were all purchased from Sigma Aldrich. Sodium hydroxide (NaOH, 97%) and nitric acid (HNO3, 97%) were provided by Aladin. Fetal bovine serum (FBS) was purchased from Institute of Biochemistry and Cell Biology, Chinese Academy of Science. Phosphate buffered saline buffer (PBS, pH = 7.4) was prepared in our own lab. All chemicals were analytical grade unless otherwise stated. Millipore deionized water was used.
2.2 Synthesis of CuS nanoparticles
0.2 mmol of CuCl2·2H2O and 0.4 mmol of MPA or MPa were dissolved in 40 mL of water in a three-neck flask at 40 °C under nitrogen flow. After stirring for 5–10 min, 2 mL of NaOH solution or HNO3 solution (1 M) was added, stirred for 3–5 h. Thereafter, 1 mL of Na2S solution (0.2 M) was quickly injected into the above solution, which was allowed to react under vigorous stirring at 50 °C for 2 h. When the reaction was finished, the resultant colloidal solution was cooled to room temperature, and was dialyzed against water for 24 h to remove unreacted molecules and ions. Aqueous dispersions of MPA-capping and MPa-capping CuS NPs were then obtained, respectively.
2.3 Synthesis of CuS mesostructures
The aqueous solutions of as-synthesized CuS NPs with opposite charges were mixed under vigorous stirring, kept stirring for 30 min at room temperature. Once the stirring was stopped, the resultant colloidal solution was centrifuged, filtered, washed with ethanol three times and vacuum dried at 40 °C, producing CuS aggregates. The CuS aggregates were then heated to 200 °C with a heating rate of 10 °C min−1 in a furnace in argon atmosphere. The samples were kept at 200 °C for 2 h to obtain CuS mesostructures.
2.4 Characterization
Scanning electron micrographs (SEM) were recorded using a JSM-7401F field emission scanning electron microscope (JEOL, Japan). Sample micrographs were recorded on a JEM-2100 transmission electron microscope (TEM, JEOL, Japan) at 200 kV. Samples were suspended in ethanol, fully dispersed by ultrasonic wave, and deposited on an amorphous carbon coated copper grid prior to observation. The JEM-2100 TEM equipped with EDX spectrometry was also used for the energy-dispersive X-ray (EDX) analysis and selected area electron diffraction (SAED). Samples were Pt coated at a sputtering rate of 1.5 kV per minute prior to observation. Powder X-ray diffraction (XRD) patterns were collected using a D/max-2200/PC X-ray diffractometer (Rigaku, Japan) fitted with nickel-filtered Cu Kα radiation. The data were collected at 0.02° intervals with counting for 0.2 s at each step. Adsorption–desorption isotherms of N2 were measured at −196 °C with an ASAP 2010M + C surface area and porosimetry analyzer (Micromeritics Inc., US) after degassing the samples. X-ray photoelectron spectroscopy (XPS) was conducted using an ESCALAB 250 X-ray photoelectron spectrometer (Thermo Scientific, US) with non-monochromatic Al Kα X-ray (1486.6 eV). The analyzer was operated at 20 eV pass energy with an energy step size of 1 eV (full spectra) and 0.1 eV (high-resolution spectra). Binding energy calibration was based on C 1s at 284.6 eV. The size distribution of the samples were determined by a Nano ZS90 particle size and zeta potential analyzer (Malvern, UK) based on dynamic light scattering (DLS) at a scattering angle of 90°. Thermogravimetry (TG) was performed on a Q5000IR thermogravimetric analyzer (TA, US) under N2 flow from room temperature to 400 °C at a heating rate of 10 °C min−1. Absorption spectra were recorded by a Lambda 35 UV-vis spectrophotometer (PerkinElmer, US), background corrected for any water contribution.
2.5 Photothermal heating experiment
Various concentrations of as-synthesized CuS mesostructure samples dispersed in water by ultrasonication were filled in quartz cuvettes (1 mL), irradiated by a 0–1 W adjustable 980 nm semiconductor laser with 5 mm diameter laser module (Xi'an Minghui Optoelectronic Technology, China) for 5 min. The output power was independently calibrated using a hand-held optical power meter (Newport model 1918-C, CA, USA) and the power density was calculated based on the power and the light spot size. The temperature of the aqueous dispersions were measured by a digital thermometer with a thermocouple (±0.1 °C) inserted into the aqueous dispersion every 12 s. Pure water was irradiated by the same NIR laser, the temperature change of which was also recorded as control.
2.6 Cell culture and cytotoxicity assay
HeLa cells were cultured in RPMI 1640 medium supplemented with 10 wt% FBS, 100 IU per mL penicillin and 100 μg mL−1 streptomycin in a humidified incubator with 5 vol% carbon dioxide at 37 °C. The medium was refreshed every 2 or 3 days according to cell density. Cytotoxicity of the CuS mesostructures was evaluated by MTT viability assay. The HeLa cells were seeded in 96-well culture plates at a density of 6000 cells per well and incubated at 37 °C for 24 h for cell attachment. The culture medium in each well was then replaced by a fresh medium containing the CuS mesostructures at different concentrations (0.01–1 mg mL−1). One row of the 96-well plates was used as control. After further incubation for 24 h or 48 h, the culture plates were rinsed with HBSS to remove unattached cells and the remaining cells were treated with 5 mg mL−1 MTT stock solution in PBS for 4 h. The medium containing unreacted MTT was then carefully removed. The obtained formazan was dissolved in DMSO, and the absorbance of individual wells was recorded at 570 nm using a Multiskan MK3 Enzyme-labeled Instrument (Thermo Scientific, US). The cell survival rate was determined by the following equation: cell survival rate% = absorbance of test cells/absorbance of control cells × 100%.
2.7 Photothermal ablation of cancer cells in vitro
HeLa cells cultured for 48 h were transferred to a 24-well plate at a density of 40
000 cells per well, in which aqueous dispersions of CuS mesostructures with different concentrations were added. Subsequently, the wells were exposed to irradiation of 980 nm laser (0.5 W cm−2) for 5 min. Then the cells were stained with calcein AM and propidium iodide, which denotes living cells and dead cells, respectively. The cells were observed by a confocal laser scanning microscope (Zeiss LSM 710, Germany).
HeLa cell viability in the presence of CuS mesostructures under the irradiation of 980 nm laser was also conducted. HeLa cells cultured for 48 h were transferred to a 96-well plate, in which aqueous dispersions of CuS mesostructures with different concentrations were added. Then the cells were irradiated by the 980 nm laser (0.5 W cm−2) for 3 and 5 min, respectively, and the cell viability was evaluated using the MTT assay.
3 Results and discussion
3.1 Morphology and dimension
The morphology and size distribution of the precursors CuS NPs were examined. In Fig. 1a, FESEM images show that the as-synthesized CuS NPs possessed spherical profiles with a narrow size distribution. High-resolution transmission electron microscopy (TEM) images in Fig. 1b demonstrate lattice fringes of the CuS NPs with the lattice spacing of 0.304 nm, which corresponds to the (102) facets characteristic of hexagonal structured CuS crystal phase.8 The MPA-capping and MPa-capping CuS NPs had an average diameter of 7.7 and 7.8 nm, respectively, based on DLS measurements as shown in Fig. 1b and c and Table 1. Zeta potential values of the CuS NPs were also measured and listed in Table 1, which confirmed that the two types of CuS NPs had opposite charges.
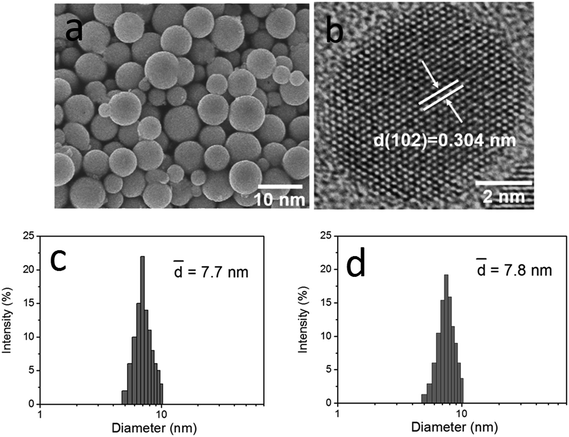 |
| Fig. 1 Representative (a) FESEM image and (b) high-resolution TEM image of as-synthesized CuS NPs. Size distribution of as-synthesized (c) MPA-capping and (d) MPa-capping CuS NPs. | |
Table 1 Parameters obtained from DLS measurements for the two types of CuS NPs
NP sample |
Hydrodynamic diameter (nm) |
Polydispersity index |
Zeta potential (mV) |
MPA-capping |
7.7 ± 1.3 |
0.11 |
−36.4 ± 14 |
MPa-capping |
7.8 ± 1.2 |
0.10 |
34.0 ± 1.6 |
Furthermore, we studied the morphology and dimension of the CuS mesostructures. FESEM images with low and high magnifications in Fig. 2a and b show the surface morphology of the CuS mesostructures. Typical TEM image of CuS mesostructures in Fig. 2c indicates that the CuS mesostructures were nanospheres with dozens of nanometers in diameter. From the high-resolution TEM image in Fig. 2d, we can clearly see well-ordered structures of the nanospheres. An assembly process was conducted for the as-synthesized CuS NPs, during which they were attracted to one another by the opposite charges. The subsequent calcination led to CuS mesostructures comprising compactly packed CuS NPs. Energy dispersion X-ray (EDX) analysis for the mesostructures in Fig. 2e indicates that the atomic ratio of Cu to S was close to the stoichiometry of CuS. The size distribution of the CuS mesostructures determined based on DLS is shown in Fig. 2f. The average size turned out to be 81 nm, in accordance with the TEM observation.
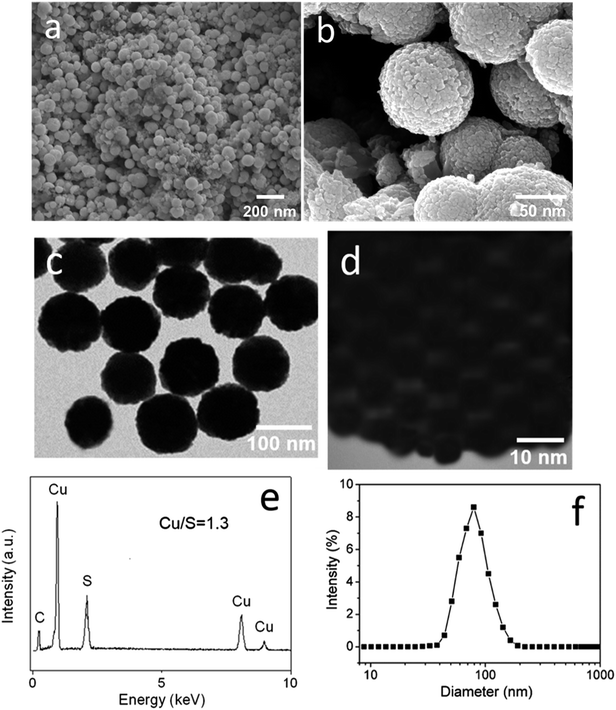 |
| Fig. 2 FESEM images with (a) low magnification and (b) high magnification, showing the surface morphology of CuS mesostructures. (c) TEM image, (d) high-resolution TEM image, (e) EDX spectrum and (f) DLS size distribution of the CuS mesostructures. | |
3.2 Structural characteristics
XPS technique is an effective tool in determining the surface chemical composition and element valence state of a sample. XPS was carried out for the CuS mesostructures, the results of which are shown in Fig. 3. The full spectrum suggests that the oxidation state of Cu (CuII) was univalent in the CuS mesostructures. The absence of N signal and the weak O and C signals indicate few capping ligands existing on the surface of the precursors (CuS NPs) during the calcination process. The high-resolution spectra represent the 2p electrons of S and Cu, respectively, further confirming the chemical composition of the CuS mesostructures.
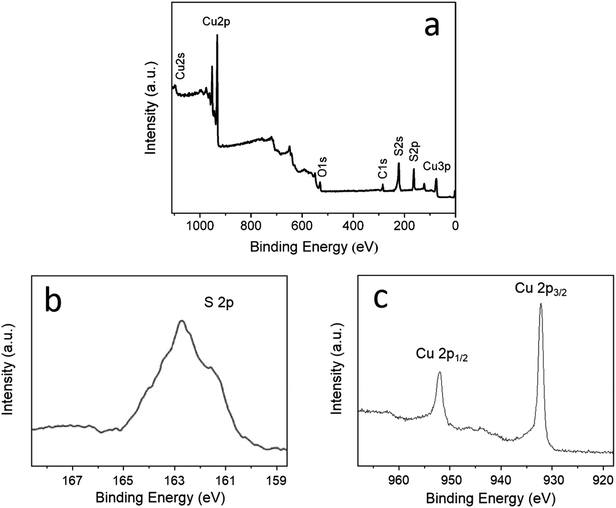 |
| Fig. 3 (a) Full XPS spectrum of the CuS mesostructures. (b) S 2p signal and (c) Cu 2p signals recorded for the CuS mesostructures. | |
The powder XRD pattern of CuS mesostructures in Fig. 3a shows indexed diffraction peaks assigned to hexagonal phase of CuS (JCPDS no. 06-0460). Considering the important role of calcination in the formation of CuS mesostructures, TGA was conducted for the CuS aggregates, i.e., the intermediate products, up to 200 °C under argon protection, as illustrated in Fig. 4b. As CuS becomes unstable at beyond 200 °C, no higher temperature was explored. The TG curve exhibited one weight-loss step from 102.2 to the end temperature, indicative of volatilization of the capping ligands during temperature increase. Judging from the total weight loss at 200 °C in combination with the above-mentioned XPS results, we can conclude that the calcination at 200 °C can remove most of the capping ligands and produce compact mesostructures.
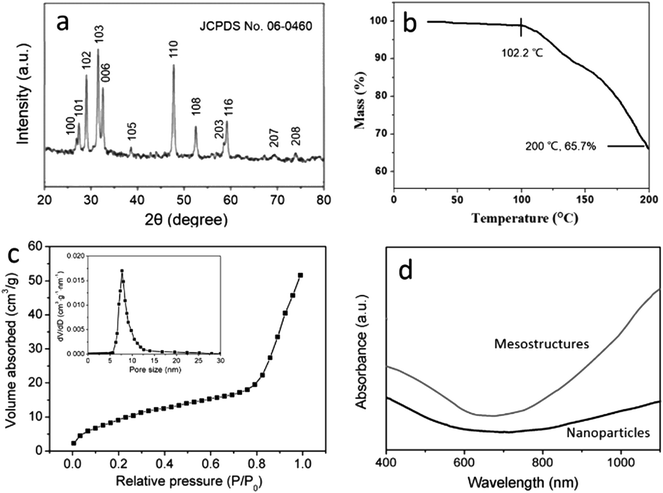 |
| Fig. 4 (a) XRD pattern of the CuS mesostructures. (b) TG curve of the CuS aggregates. (c) N2 adsorption–desorption isotherms and (inset) pore size distribution of the CuS mesostructures. (d) Absorption spectra of the aqueous dispersion of as-synthesized CuS superstructures and comparable CuS NPs. | |
Surface area and porosity analysis is a useful tool for characterizing mesoporous materials. The N2 adsorption/desorption isotherm of the CuS mesostructures in Fig. 4c show a typical type IV curve. The corresponding Brunauer–Emmett–Teller (BET) surface area was calculated to be 48 m2 g−1. The Barrett–Joyner–Halenda (BJH) pore size distribution curve (Fig. 4c, inset) suggest an average pore size of 8.3 nm. The optical property of the CuS mesostructures was investigated. Ultrasonication was utilized to assist dispersion of the as-synthesized CuS mesostructures in water (0.025 g L−1). Fig. 4d shows absorption spectrum of the CuS mesostructures obtained by UV-vis-NIR spectroscopy. The absorption of aqueous dispersion containing MPA-capping CuS NPs with the same concentration was also recorded for comparison. Both spectra showed an absorption edged at ∼600 nm, corresponding to a band gap of 2.07 eV. Moreover, a dramatic increase of absorption in the NIR region was observed, attributed to inter band transitions from valence states to the unoccupied states (indirect band gap).24 It is noted that the absorption intensity of the CuS mesostructures was much larger than that of the CuS NPs in the NIR region, demonstrating the high NIR absorption efficiency of the honeycomb-like mesostructures.
3.3 Photothermal properties
When the photothermal performance of the CuS mesostructures is examined, sample concentration and irradiation dose are essential factors that should be considered. To this end, the aqueous dispersions of CuS mesostructures with different concentrations were prepared by sonication and exposed to continuous irradiation of a 980 nm laser at the power density of 0.5 W cm−2. The resultant temperature changes were recorded over a period of 5 min, as depicted in Fig. 5a. With the increase of CuS mesostructures concentration, the temperature elevation was increasingly significant, revealing the collective heating effect of the mesostructures. However, the temperature increment was not linear with the concentration (Fig. 5a, inset). In addition, the temperature changes of the sample with the concentration of 0.3 g L−1 were traced under the irradiation of varied power densities, as shown in Fig. 5b. The temperature elevation was larger at a higher power density, as expected. When the power density exceeded 0.75 W cm−2, the temperature change vs. power density curve levelled off (Fig. 5b, inset). The photothermal stability of the CuS mesostructures was evaluated by applying repeated on and off laser irradiation to the sample. It can be seen from Fig. 5c that the photothermal behaviour of the CuS mesostructures did not show any sign of fatigue, indicating the excellent photothermal stability that is advantageous for therapeutical applications. In a previously published work,9 flower-like CuS superstructures (0.5 g L−1) heated the water from room temperature to ∼45 °C within 5 min under irradiation of 980 nm laser (0.51 W cm−2). Under similar irradiation conditions, the water temperature increased by nearly the same value at the CuS mesostructures concentration of merely 0.3 g L−1 in this work. The photothermal conversion efficiency of the CuS mesostructures turned out to be 24.4%, much higher than that of their precursors (7.5% for CuS NPs). Obviously, the photothermal performance strongly depends on the morphology of CuS. The CuS mesostructures can absorb irradiation energy efficiently attributable to the unique honeycomb-like structure.
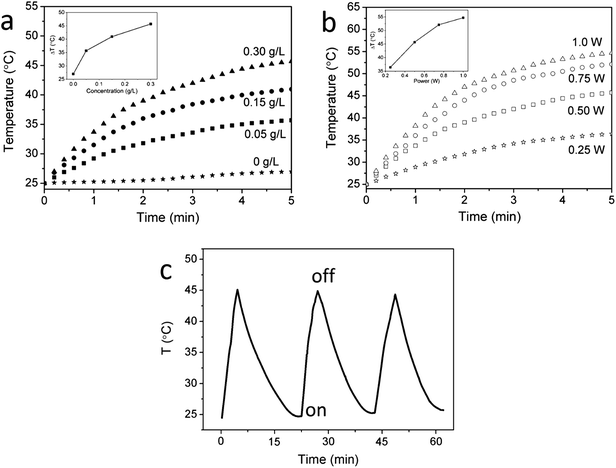 |
| Fig. 5 (a) Temperature elevation of the aqueous dispersion of CuS mesostructures with different concentration as a function of 980 nm irradiation time at the irradiation power density of 0.5 W cm−2; (inset) temperature change (ΔT) vs. CuS mesostructure concentration over a period of 5 min. (b) Temperature elevation of the aqueous dispersion of CuS mesostructures at the concentration of 0.30 g L−1 as a function of 980 nm irradiation time at varied irradiation power density; (inset) temperature change (ΔT) vs. irradiation power density over a period of 5 min. (c) Temperature changes of the aqueous dispersion of CuS mesostructures (0.3 g L−1) under repeated on–off irradiation of 980 nm laser at the irradiation power density of 0.5 W cm−2. | |
The NIR light-induced thermal effect makes the CuS mesostructures potential agents for cancer therapy. In general, heating to 40–45 °C is sufficient to kill cancer cells.25,26 In vitro photothermal heating experiment for HeLa cells was designed to confirm the cancer cell killing ability of the CuS mesostructures. To eliminate the toxicity of CuS to the cells, cytotoxicity test was conducted first. The results of MTT assay in Fig. 6a suggested low cytotoxicity of the CuS mesostructures even at a high concentration of 1 mg mL−1. We subjected the HeLa cells to irradiation of 980 nm laser for 3 min and 5 min, respectively, in the presence of CuS mesostructures, and performed MTT assay to determine the cell viability. As a control, the cells were incubated with the CuS mesostructures for 5 min without irradiation. It was found from Fig. 6b that the cell survival rate decreased with increasing concentration under the same irradiation condition. At the same concentration, longer irradiation time led to lower cell viability. 79% of the cells were killed by the heat released from the 0.3 g L−1 CuS mesostructures under the irradiation for 5 min.
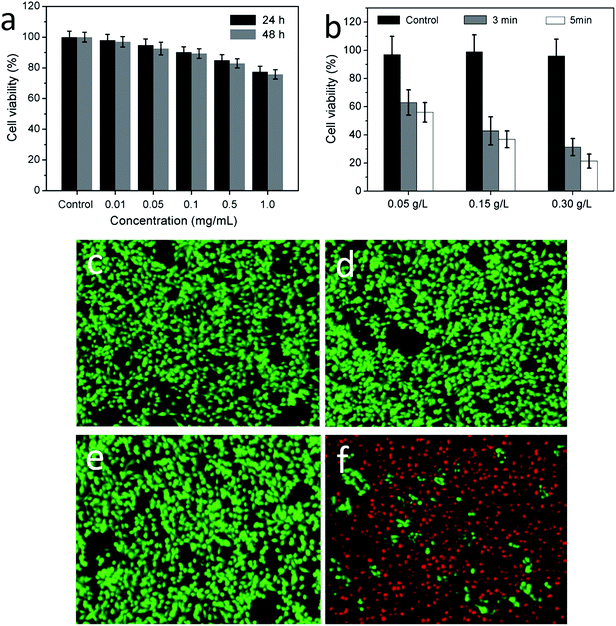 |
| Fig. 6 (a) Cell viability after incubation with various concentrations of CuS mesostructures for 24 and 48 h. (b) HeLa cell viability after treatment with various concentrations of CuS mesostructures under irradiation of 980 nm laser at the irradiation power density of 0.5 W cm−2 for 3 min and 5 min. The control: incubation for 5 min. Fluorescence images of HeLa cells before and after irradiation by 980 nm laser with the power density of 0.5 W cm−2 over a period of 5 min in the absence (c and d) and presence (e and f) of the CuS mesostructures with the concentration of 0.3 g L−1. The living cells were labeled by calcein AM (green emission), and the dead cells were labeled by propidium iodide (red emission). The scale bar represents 100 μm. | |
Fluorescence microscopy observation is a direct approach to judge whether the cells are killed. The HeLa cells were incubated at 37 °C with or without the presence of CuS mesostructures, meanwhile irradiated by the 980 nm laser for 5 min. The cells were then stained with calcein AM (denoting living cells) and propidium iodide (denoting dead cells), and were observed on a confocal laser scanning microscope. In Fig. 5c and d, there was no evidence of cell death in the absence of CuS mesostructures, as the cells in the field of view emitted strong green fluorescence characteristic of calcein. In Fig. 5e and f, on the contrary, most of the cells died under the same irradiation condition in the presence of the CuS mesostructures, as indicated by the red emission from the dead cells. These results confirm that the CuS mesostructures are capable of killing cancer cells efficiently when exposed to 980 nm laser. In consideration of biomedical purposes, surface modification of the CuS mesostructures to prevent aggregation may be a research focus in our future work.
4 Conclusions
In summary, photothermal CuS mesostructures have been successfully synthesized by assembly of oppositely charged CuS NPs and then calcination at elevated temperature. The CuS mesostructures are honeycomb-like mesoporous nanospheres with high specific surface area, and more importantly, have high photothermal conversion efficiency. Under 980 nm laser irradiation, the CuS mesostructures can be heated in a short time and are able to kill cancer cells in vitro. This work provides new insights for scale-up production of photothermal mesostructured materials for cancer therapy.
Acknowledgements
This work was financially supported by the Project of Shandong Province Higher Educational Science and Technology Program (J14LM02), the Science and Technology Research Program of Shangdong Academy of Medical Sciences (2014-40), the National Natural Science Foundation (51403125) and the Specialized Research Fund for the Doctoral Program of Higher Education (20130073120087).
References
- L. Qian, J. Mao, X. Tian, H. Yuan and D. Xiao, Sens. Actuators, B, 2013, 176, 952 CrossRef CAS PubMed.
- G. Ku, M. Zhou, S. Song, Q. Huang, J. Hazle and C. Li, ACS Nano, 2012, 6, 7489 CrossRef CAS PubMed.
- S. Ramadan, L. Guo, Y. Li, B. Yan and W. Lu, Small, 2012, 8, 3143 CrossRef CAS PubMed.
- Q. Tian, F. Jiang, R. Zou, Q. Liu, Z. Chen, M. Zhou, S. Yang, J. Wang, J. Wang and J. Hu, ACS Nano, 2011, 5, 9761 CrossRef CAS PubMed.
- C. M. Hessel, V. P. Pattani, M. Rasch, M. G. Panthani, B. Koo, J. W. Tunnell and B. A. Korgel, Nano Lett., 2011, 11, 2560 CrossRef CAS PubMed.
- Q. Xiao, X. Zheng, W. Bu, W. Ge, S. Zhang, F. Chen, H. Xing, Q. Ren, W. Fan, K. Zhao, Y. Hua and J. Shi, J. Am. Chem. Soc., 2013, 135, 13041 CrossRef CAS PubMed.
- Z. Zha, S. Wang, S. Zhang, E. Qu, H. Ke, J. Wang and Z. Dai, Nanoscale, 2013, 5, 3216 RSC.
- M. Zhou, R. Zhang, M. A. Huang, W. Lu, S. L. Song, M. P. Melancon, M. Tian, D. Liang and C. Li, J. Am. Chem. Soc., 2010, 132, 15351 CrossRef CAS PubMed.
- Q. Tian, M. Tang, Y. Sun, R. Zou, Z. Chen, M. Zhu, S. Yang, J. Wang, J. Wang and J. Hu, Adv. Mater., 2011, 23, 3542 CrossRef CAS PubMed.
- X. Bu, D. Zhou, J. Li, X. Zhang, K. Zhang, H. Zhang and B. Yang, Langmuir, 2014, 30, 1416 CrossRef CAS PubMed.
- J. Bai, Y. Liu and X. Jiang, Biomaterials, 2014, 35, 5805 CrossRef CAS PubMed.
- K. Dong, Z. Liu, Z. Li, J. Ren and X. Qu, Adv. Mater., 2013, 25, 4452 CrossRef CAS PubMed.
- P. Yang, T. Deng, D. Zhao, P. Feng, D. Pine, B. F. Chmelka, G. M. Whitesides and G. D. Stucky, Science, 1998, 282, 2244 CrossRef CAS.
- H. Wu, D. Kong, Z. Ruan, P.-C. Hsu, S. Wang, Z. Yu, T. J. Carney, L. Hu, S. Fan and Y. Cui, Nat. Nanotechnol., 2013, 8, 421 CrossRef CAS PubMed.
- A. Corma, P. Atienzar, H. García and J.-Y. Chane-Ching, Nat. Mater., 2004, 3, 394 CrossRef CAS PubMed.
- H. Zheng, Z. Huang and S. Che, Dalton Trans., 2012, 5038 RSC.
- I. Zlotnikov, P. Werner, H. Blumtritt, A. Graff, Y. Dauphin, E. Zolotoyabko and P. Fratzl, Adv. Mater., 2014, 26, 1681 CrossRef PubMed.
- M. J. Maclachlan, N. Coombs and G. A. Ozin, Nature, 1999, 397, 681 CrossRef CAS PubMed.
- B. Tian, X. Liu, C. Yu, J. Fan, L. Wang, S. Xie, G. D. Stucky and D. Zhao, Nat. Mater., 2003, 2, 159 CrossRef CAS PubMed.
- F. Jiao, A. Harrison, A. H. Hill and P. G. Bruce, Adv. Mater., 2007, 19, 4063 CrossRef CAS PubMed.
- F. Jiao, A. H. Hill, A. Harrison, A. Berko, A. V. Chadwick and P. G. Bruce, J. Am. Chem. Soc., 2008, 130, 5262 CrossRef CAS PubMed.
- D. Wang, T. Xie, Q. Peng and Y. Li, J. Am. Chem. Soc., 2008, 130, 4016 CrossRef CAS PubMed.
- J. Qian, M. Zhou, Y. Cao, X. Ai and H. Yang, J. Phys. Chem. C, 2010, 114, 3477 CAS.
- L. F. Chen, W. Yu and Y. Li, Powder Technol., 2009, 191, 52 CrossRef CAS PubMed.
- G. M. Hahn, J. Braun and I. Har-Kedar, Proc. Natl. Acad. Sci. U. S. A., 1975, 72, 937 CrossRef CAS.
- J. van der Zee, Ann. Oncol., 2002, 13, 1173 CrossRef CAS PubMed.
|
This journal is © The Royal Society of Chemistry 2015 |