DOI:
10.1039/C4RA17229A
(Paper)
RSC Adv., 2015,
5, 15006-15016
Highly efficient copper-imprinted functionalized mesoporous organosilica nanocomposites as a recyclable catalyst for click synthesis of 1,2,3-triazole derivatives under ultrasound irradiation: multivariate study by factorial design of experiments†
Received
29th December 2014
, Accepted 15th January 2015
First published on 15th January 2015
Abstract
We have developed a convenient green method for one-pot synthesis of β-hydroxy-1,2,3-triazoles through three-component coupling of a variety of epoxides and alkynes over copper-imprinted periodic mesoporous organosilica nanocomposites (Cu@PMO NCs) in water under ultrasound irradiation. The surface response obtained from an experimental Box-Behnken design (BBD) was used to model the catalytic synthesis of β-hydroxy-1,2,3-triazole. This method was an economical way of obtaining the optimal reaction conditions based on a restricted number of experiments. Reactions were performed in ultrasound equipment and different variables, such as the amount of catalyst, ultrasound irradiation frequency and ultrasound time, were investigated. Our method is more environmentally friendly than previous methods because it uses water as the solvent, uses ultrasound irradiation, uses a catalyst that is reusable for up to six runs without appreciable loss of activity, and has short reaction times and high yields. Furthermore, the mathematical model of the reaction represents the process well and shows good correlation with the experimental results.
Introduction
According to the principle of green chemistry, synthetic methods should be designed to use substances and reaction conditions that exhibit little or no toxicity to human health and the environment. Thus, recent achievements in chemical efficiency have focused on use of heterogeneous catalysts in organic reactions. The majority of heterogeneous catalysts are based on silica supports, primarily because silica displays some advantageous properties, such as excellent chemical and thermal stability, porosity, and good accessibility. Furthermore, organic groups can be robustly imprinted on periodic mesoporous organosilica (PMO) or anchored to the silica framework to provide catalytic centers.1,2 A wide range of studies have been carried out on the preparation of molecular-imprinted silica hybrids based on inorganic mediums, such as surface immobilization on periodic mesoporous silica materials (SBA-15, MCM-41) and silica particles.3–6 Molecular imprinting on PMO is an important technique for preparing materials with selective recognition properties owing to its cost effectiveness and ease of use compared with complicated biological systems.7–9
An interesting feature of metal-imprinted PMO hybrids is their convenient modification with a relatively high degree of functionality. The ordered mesostructure modification allows a wide range of applications, including heterogeneous catalysis, selective recognition, separation and sensor devices.10,11 1,2,3-Triazole derivatives are usually obtained through the copper-catalyzed Huisgen 1,3-dipolar cycloaddition of azides and alkynes using CuAAC as catalyst.12–14 Recently, this reaction has been developed with different heterogeneous catalysts and a source of copper in water.15–22 One-pot synthesis of β-hydroxy-1,2,3-triazoles from epoxides, linked to dipolar cycloaddition with azides and alkynes has been reported through heterogeneous catalytic systems such as copper nanocomposites on activated carbon,23,24 Cu(I)-modified zeolites,25 Cu(I)@phosphorated SiO2,26 porphyrinato copper nanocomposites,27 Cu[N2,N6-bis(2-hydroxyphenyl) pyridine-2,6-dicarbox-amidate].28 Ultrasound techniques have increasingly been used in organic synthesis during the last few years. Compared with traditional methods, this method can give higher yields in shorter reaction times and under milder conditions.29 Ultrasound irradiation may affect heterogeneous catalytic systems by increasing the active catalyst surface area, promoting cavitation bubble formation and removing impurities deposited on the catalyst.30 Moreover, click synthesis of 1,2,3-triazole derivatives from a variety of alkyl halides or organic azides and alkynes under ultrasound irradiation using green catalysts, such as copper and iron, is attractive in the growing field of green chemistry.31,32
Continuing our work on the preparation and reactivity of hybrid copper-imprinted periodic mesoporous organosilica (Cu@PMO NCs), we found that is an excellent catalyst for the synthesis of β-hydroxy-1,2,3-triazoles under thermal conditions. Herein, we report a convenient green approach to the three-component synthesis of β-hydroxy-1,2,3-triazoles from epoxides, terminal alkynes and sodium azide catalyzed by Cu@PMO NCs under ultrasound irradiation in water at room temperature. To determine the conditions suitable for the reaction, we employed the experimental Box-Behnken design (BBD)33,34 that uses the surface response method. Three variables of catalyst amount, ultrasound irradiation frequency and ultrasound time were selected. The optimized conditions were assessed to obtain the best product yield, reaction time, and stability and recyclability of the catalyst.
Experimental
Experimental design
A BBD composed of three variables over three levels was used to maximize the Cu@PMO NCs activity in terms of β-hydroxy-1,2,3-triazole yields and hence reduce the number of experiments without compromising the results. BBD 15 (2k + 2k + 1, k = 3) was applied with central points in triplicate for the reaction. The three variables and their levels are described below. Variables were chosen to add economic value by reducing the process cost through lower catalyst load and ultrasound time. Ultrasound irradiation was chosen for high β-hydroxy-1,2,3-triazole production at room temperature. The experimental design and results for the reaction over Cu@PMO NCs are listed in Table 1.
Table 1 Experimental design and BBD results for β-hydroxy-1,2,3-triazole (3a)
Entry |
Cu@PMO NCs content (%)a |
Ultrasound irradiation frequency (kHz) |
Ultrasound time |
Yield (%) |
(+ upper, 0 central, − lower). |
1 |
0.005 (0) |
40 (0) |
7 (0) |
98 |
2 |
0.01 (+) |
40 (0) |
10 (+) |
95 |
3 |
0.003 (−) |
40 (0) |
5 (−) |
80 |
4 |
0.005 (0) |
30 (−) |
5 (−) |
84 |
5 |
0.01 (+) |
30 (−) |
7 (0) |
92 |
6 |
0.005 (0) |
40 (0) |
7 (0) |
98 |
7 |
0.003 (−) |
40 (0) |
10 (+) |
80 |
8 |
0.005 (0) |
40 (0) |
7 (0) |
98 |
9 |
0.01 (+) |
40 (0) |
5 (−) |
95 |
10 |
0.005 (0) |
30 (−) |
10 (+) |
93 |
11 |
0.005 (0) |
50 (+) |
5 (−) |
93 |
12 |
0.005 (0) |
50 (+) |
10 (+) |
85 |
13 |
0.01 (+) |
50 (+) |
7 (0) |
90 |
14 |
0.003 (−) |
50 (+) |
7 (0) |
84 |
15 |
0.003 (−) |
30 (−) |
7 (0) |
80 |
General information
All chemicals solvents were purchased from commercial suppliers. FT-IR spectra were obtained by using KBr pellets on a PerkinElmer 781 spectrophotometer and on an impact 400 Nicolet FT-IR spectrophotometer. The XRD patterns were recorded on an X-ray diffractometer (D8 ADVANCE, Bruker, Germany) using Cu-Kα radiation (λ = 0.154056 nm) in the range 2θ = 0.5°–5°. Thermogravimetric analysis (TGA) was carried out on STA 503 WinTA instrument at a heating rate of 10 °C min−1 under a N2 atmosphere. The N2 adsorption/desorption analysis (BET) was performed at −196 °C using an automated gas adsorption analyzer (Tristar 3000, Micromeritics). The surface morphology of the supported catalyst was studied by scanning electron microscopy (SEM). FE-SEM and elemental analysis were carried out using a JEOL SEM instrument (VEGA/TESCAN) combined with an INCA instrument for energy dispersive X-ray spectroscopy scanning electron microscopy (EDS-SEM), with a scanning electron electrode at 15 kV. The diffuse reflectance UV-visible spectra of samples were recorded at room temperature on a PerkinElmer 35 LAMBDA instrument using barium sulfate as a reference. Liquid NMR was obtained on a Bruker DRX-400 MHz Bruker Avance instrument using CDCl3 and DMSO as solvent and TMS as an internal standard. The Bandelin ultrasonic HD 3200 with a KE 76 probe, 6 mm diameter, was used to produce ultrasonic irradiation and to homogenize the reaction mixture.
Preparation of organometallic LCu
The 1,1-[2,4,6-triyl-1,3,5-triazinebis(nitrilomethylidine)]bis-(phenol) ligand (1) was synthesized by dissolving salicylaldehyde (0.214 g, 2 mmol) and melamine (0.126 g, 1.0 mmol) in methanol (5 mL) and three drops of acetic acid. The reaction mixture was heated under reflux at 100 °C for 4 h. After completion of the reaction, the ligand product was filtered, washed with water, and dried at ambient temperature, resulting in the formation of an orange solid in 98% yield. IR (KBr): ν = 3468, 3416, 3332, 3129, 1654, 1552, 1439, 1272. 1H NMR (400 MHz, DMSO) δ = 10.71 (s, 1H, O–H), 10.24 (s, 1H, O–H), 7.65–6.63 (m, 8H, aromatic), 6.04 (s, 2H, N–H). 13C NMR (100 MHz, DMSO) δ = 178.34, 174.44, 167.81, 164.94, 130.40, 128.82, 125.61, 121.09, 119.94. Anal. calcd for C, H, N: 61.07 (C), 4.22 (H), 25.14 (N); found: 61.10 (C), 4.18 (H), 25.15 (N).
To prepare organometallic LCu (2), the ligand (0.332 g, 1 mmol) was dissolved in dimethylformamide (15 mL) and treated with copper acetate monohydrate (Cu(OAc)2·H2O), heated under reflux for 3 h. Finally, the metal–ligand complex was filtered, washed with 96% ethanol (100 mL) and dried for 1 day under vacuum to produce the complex as brown crystals in 95% yield. IR (KBr): ν = 3466, 3419, 3343, 3131, 1650, 1548, 1438, 1204.
Preparation of copper-imprinted organopolysilane precursor
Copper-imprinted organopolysilane precursor 3 was obtained as follows. LCu (2) (0.5 g, 1.3 mmol) was dissolved in dry ethanol (60 mL) and 3-chloropropytrimethoxysilane (0.5 mL, 2.6 mmol) was added dropwise under a N2 atmosphere in the presence of NaH as a base. The reaction mixture was stirred at 60 °C for 24 h under a N2 atmosphere. Subsequently, the reaction mixture was filtered, concentrated under vacuum, purified with diethylether (3 × 50 mL) and dried under vacuum. Copper-imprinted organopolysilane precursor 3 was obtained in 90% yield. IR (KBr): ν = 3402, 2922, 2852, 1558, 1460, 1266. Anal. calcd for C, H, N: 48.35 (C), 5.60 (H), 11.67 (N). Found: 47.29 (C), 5.62 (H), 10.82 (N).
Synthesis of copper-imprinted periodic mesoporous organosilica nanocomposite (Cu@PMO NCs)
Copper-imprinted periodic mesoporous organosilica nanocomposite (Cu@PMO NCs) was prepared from three constituents: copper-imprinted organopolysilane precursor, oligomer silicates from tetraethylorthosilicate (TEOS), and a cetyltrimethyl ammonium bromide (CTAB) template. In a typical preparation, CTAB (1.0 g, 2.74 mmol) was dissolved in distilled water (47 mL). The solution was stirred for 10 min, followed by the addition ammonia (11 mL, 25%) and stirred for a further 30 min to obtain a clear solution. A premixed propanol solution of organopolysilane precursor 3 and TEOS were added to the surfactant solution. The molar composition of the final synthetic mixture was TEOS
:
3
:
CTAB
:
NH3
:
H2O
:
propanol = (1 + x)
:
x
:
0.12
:
8.0
:
114
:
7, where x = 0.05 for Cu@PMO NCs, respectively. The reaction mixture was stirred at 35 °C for 24 h. The final mixture was then crystallized at 90 °C for 24 h. The resulting solid was filtered and washed with deionized water, and dried in a vacuum at 60 °C for 12 h. The surfactant was removed by extracting the solid with a 0.05 M ethanolic HCl solution at 60 °C for 6 h (50 mL of 0.05 ethanolic HCl for 1 g of solid material). The obtained material was designated as Cu@PMO NCs.
Typical ultrasound-promoted procedure for synthesis of β-hydroxy-1,2,3-triazoles (3a)
A 25 mL Erlenmeyer flask was charged with the styrene oxide (1 mmol), NaN3 (72 mg, 1.1 mmol), the alkyne (1 mmol), and Cu@PMO NCs (7 mg, 0.33 mol%) in H2O (3 mL). The reaction flask was placed in the ultrasonic probe and irradiated at 40 kHz at room temperature for 7 min. The solid product was collected from the reaction mixture. To separate the catalyst, the product was dissolved in hot methanol and passed through a sintered glass filter funnel. The solvent was removed under vacuum to give product 3a in 99% yield. Other derivatives of β-hydroxy-1,2,3-triazole (3b–l) were synthesized with this procedure.
2-Phenyl-2-(4-phenyl-1H-1,2,3-triazol-1-yl)ethanol (3a). Pale yellow solid: mp. 125–127 °C, lit23 (mp. 125.5–127 °C). IR (KBr): ν = 693, 758, 1045, 1079, 1242, 1436, 1463, 1493, 1595, 2928, 3064, 3087, 3140, 3346. 1H NMR (400 MHz, CDCl3) δ = 7.81–7.79 (m, 3H), 7.70 (s, 1H), 7.43–7.39 (m, 6H) 7.34 (s, 1H), 5.68 (dd, 3J(H,H) = 8 Hz, 3J = 3.9 Hz, 1H), 4.68–4.61 (dd, 3J(H–H) = 12.4 Hz, 3J(H–H) = 7.6 Hz, 1H), 4.26–4.21 (dd, 3J(H–H) = 14.4 Hz, 3J(H–H) = 3.6 Hz, 1H), 3.22 (t, 3J(H,H) = 6.8 Hz; 1H, OH).
2-(4-Phenyl-1H-1,2,3-triazol-1-yl)-1-p-tolylethanol (3b). Yellow solid: mp. 125–127 °C, lit35 (mp. 124–126 °C). IR (KBr): ν = 696, 724, 755, 1047, 1075, 1008, 1185, 1220, 1380, 1457, 1496, 2927, 3029, 3092, 3418. 1H NMR (400 MHz, CDCl3) δ = 7.69–7.66 (m, 3H), 7.39 (m, 3H), 7.27–7.21 (m, 3H), 5.68–5.66 (dd, 3J(H,H) = 8 Hz, 3J = 3.2 Hz, 1H), 4.66–4.61 (dd, 3J(H–H) = 12.6 Hz, 3J(H–H) = 8.4 Hz, 1H), 4.24–4.20 (dd, 3J(H–H) = 9 Hz, 3J(H–H) = 3.6 Hz, 1H), 2.38 (s, 3H), 2.36 (t, 3J(H, H) = 2.8 Hz; 1H, OH).
2-(4-Phenyl-1H-1,2,3-triazol-1-yl) cyclohexanol (3c). Yellow solid: mp. 168–171 °C, lit23 (mp. 168–171 °C). IR (KBr): ν = 699, 768, 713, 1053, 1083, 1234, 1441, 2858, 2937, 3119, 3307. 1H NMR (400 MHz, CDCl3) δ = 7.79 (s, 1H), 7.74–7.72 (m, 2H), 7.40–7.37 (m, 2H), 7.33–7.30 (s, 1H), 4.19–4.09 (m, 3H), 4.01–1.90 (m, 4H), 2.25–2.22 (m, 2H), 1.51–1.26 (m, 2H).
1-(4-Phenyl-1H-1,2,3-triazol)hexan-2-ol (3d). Cream solid: mp. 92–94 °C, lit35 (mp. 91–93 °C). IR (KBr): ν = 694, 764, 1087, 1138, 1227, 1463, 2862, 2925, 2959, 3141, 3248, 3408. 1H NMR (400 MHz, CDCl3) δ = 7.85 (s, 1H), 7.77–7.75 (m, 2H), 7.42–7.40 (m, 2H), 7.34–7.32 (m, 1H), 4.52–4.49 (m, 2H), 4.29–4.24 (m, 1H), 4.15–4.13 (m, 1H), 1.57–1.51 (m, 3H), 1.44–1.37 (m, 3H), 0.95–0.92 (t, 3H). 13C NMR (100 MHz, CDCl3) δ = 147.27, 130.33, 128.78, 128.06, 125.53, 121.16, 70.44, 56.34, 34.17, 30.77, 28.01, 22.59, 13.99.
1-(4-Phenyl-1H-1,2,3-triazol-1-yl)butan-2-ol (3e). White solid: mp. 110–111 °C, lit26 (mp. 110–111 °C). IR (KBr): ν = 694, 763, 982, 1078, 1136, 1228, 1457, 1617, 2926, 2962, 3139, 3254, 3419. 1H NMR (400 MHz, CDCl3) δ = 7.87 (s, 1H), 7.81–7.79 (m, 2H), 7.44–7.40 (m, 2H), 7.34 (m, 1H), 4.55–4.51 (dd, 3J(H–H) = 14 Hz, 1H), 4.33–4.29 (dd, 3J(H–H) = 13.8 Hz, 2J(H–H) = 7.6 Hz, 1H), 2.66–2.65 (d, 3J(H–H) = 4.4 Hz, 1H), 1.66–1.53 (m, 2H), 1.09–1.05 (t, 3H). 13C NMR (100 MHz, CDCl3) δ = 147.44, 130.40, 128.82, 128.12, 125.61, 121.09, 71.86, 55.84, 27.48, 9.81.
1-Phenoxy-3-(4-phenyl-1H-1,2,3-triazol-1-yl)propan-2-ol (3f). Pale yellow solid: mp. 125–127 °C, lit27 (mp. 125.5–126 °C). IR (KBr): ν = 691, 755, 982, 1043, 1245, 1494, 1595, 2867, 2927, 3087, 3429. 1H NMR (400 MHz, CDCl3) δ = 7.89 (s, 1H), 7.75–7.73 (m, 2H), 7.41–7.38 (m, 2H), 7.32–7.27 (m, 3H), 7.02–6.93 (m, 1H), 6.93–6.91 (m, 2H), 4.75–4.73 (m, 1H), 4.56–4.54 (m, 2H), 4.23–4.04 (m, 2H), 3.95–3.76 (m, 1H).
3-Isoproxy-2-(4-phenyl-1H-1,2,3-triazol-1-yl)propan-1-ol (3g). Yellow solid: mp. 61–63 °C, lit26 (mp. 61–63 °C). R (KBr): ν = 695, 765, 924, 975, 1078, 1127, 1228, 1373, 1468, 2866, 2973, 3062, 3138, 3425. 1H NMR (400 MHz, CDCl3) δ = 7.91 (s, 1H), 7.79–7.77 (m, 2H), 7.42–7.38 (m, 2H), 7.34–7.30 (m, 1H), 4.61–4.57 (dd, 3J(H–H) = 12 Hz, 2J(H–H) = 2.8 Hz, 1H), 4.64–4.41 (dd, 3J(H–H) = 14 Hz, 2J(H–H) = 4 Hz, 1H), 4.22 (m, 1H), 3.64–3.58 (m, 1H), 3.53–3.50 (dd, 3J(H–H) = 8 Hz, 3J(H–H) = 4.4 Hz, 1H), 3.40–3.36 (dd, 3J(H–H) = 10 Hz, 3J(H–H) = 4 Hz, 1H), 1.16–1.17 (t, 6H). 13C NMR (100 MHz, CDCl3) δ = 131.55, 130.36, 129.94, 129.21, 126.72, 122.49, 73.59, 70.45, 70.18, 54.49, 23.14.
2-Hydroxy-3-(4-phenyl-1H-1,2,3-triazol-1-yl)propyl acrylate (3h). Gray solid: mp. 101–103 °C, lit26 (mp. 101–103 °C). IR (KBr): ν = 692, 756, 1044, 1246, 1494, 1596, 2925, 3087, 3425. 1H NMR (400 MHz, CDCl3) δ = 7.91 (s, 1H), 7.79–7.77 (m, 1H), 7.43–7.40 (m, 2H), 7.35–7.31 (m, 2H), 7.02–6.99 (t, 1H), 6.93–6.91 (m, 2H), 4.22 (m, 1H), 3.64–3.58 (m, 1H), 4.07–3.98 (m, 1H), 3.38–3.37 (m, 1H). 13C NMR (100 MHz, CDCl3) δ = 158.25, 147.29, 130.10, 129.63, 128.86, 128.19, 125.56, 121.66, 121.40, 121.14, 114.57, 68.98, 68.70, 53.52.
1,3-Bis(5-phenyl-1H-1,2,3-triazol-1-yl) propan-2-ol (3i). Green solid: mp. 233–236 °C, lit36 (mp. 233–236 °C). IR (KBr): ν = 688, 724, 763, 1074, 1125, 1278, 1464, 1729, 2860, 2928, 2959. 1H NMR (400 MHz, DMSO) δ = 8.54 (s, 1H), 7.84 (m, 2H), 7.44–7.43 (m, 2H), 7.32–7.31 (m, 1H), 5.81 (m, 1H), 4.62–4.56 (d, 1H), 4.40 (m, 2H). 13C NMR (100 MHz, DMSO) δ = 147.71, 132.42, 130.51, 129.68, 129.41, 127.11, 126.72, 124.12, 69.91, 54.84.
Results and discussion
Preparation and characterization of catalyst
Periodic mesoporous organosilicas (PMOs) represent an exciting new class of organic–inorganic nanocomposites for catalysis. Fabricated from an organosilane precursor with a metal template imprinted ligand centre, an interphase catalyst can simulate homogeneous reaction conditions and has the advantages of easy separation and recovery of the heterogeneous catalysts. In the present study, we use the interphase strategy to prepare a copper-imprinted periodic mesoporous organosilica nanocomposites (Cu@PMO NCs) for use as a heterogeneous catalyst for three-component synthesis of β-hydroxy-1,2,3-triazoles from epoxides, sodium azide and non-activated terminal alkynes. The first step in the accomplishment of this goal was the synthesis of (Cu@PMO NCs) (Scheme 1). To prepare the catalyst, initially ligand 1 was synthesized from the reaction of salicylaldehyde and melamine via a thermal method. Then, LCu (2) and copper-imprinted organopolysilane precursor 3 were prepared using Cu(OAc)2·H2O and a trimethoxysilyl moiety, respectively. Finally, the catalyst was obtained by using a sol–gel process from a chemically synthesized organopolysilane precursor with a metal template (Cu(II)) imprinted ligand centre with tetraethylorthosilicate (TEOS) and cetyltrimethylammoniumbromide (CTAB) as a structural directing surfactant under basic conditions. The preparation procedure to obtain the catalyst (Cu@PMO NCs) is shown in Scheme 1.
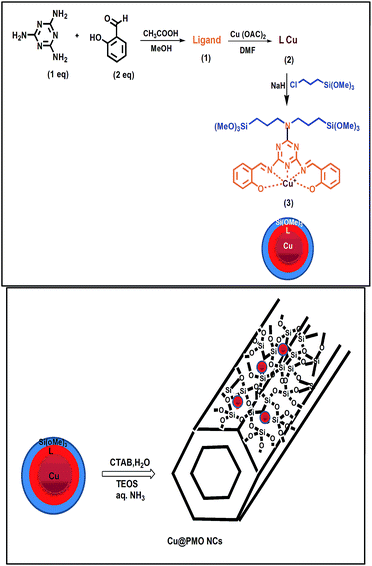 |
| Scheme 1 General route for the synthesis of LCu (2), copper-imprinted organopolysilane precursor 3 and Cu@PMO NC catalysts. | |
Characterization of catalyst
The X-ray diffraction (XRD) pattern of the Cu@PMO NCs showed a sharp peak at 2θ = 0.9° and some weak peaks in 2θ = 1.7–2.45°. The XRD spectrum (Fig. 1) and FE-SEM image (Fig. 2a) confirm the formation of the well-ordered two-dimensional (2D) hexagonal PMOs with a high content of the copper template imprinted ligand centre groups. As can be clearly seen in Fig. 2, the Cu@PMO NCs nanocomposite catalyst has a spherical structure with a size distribution of around 86 nm (Fig. 2b).
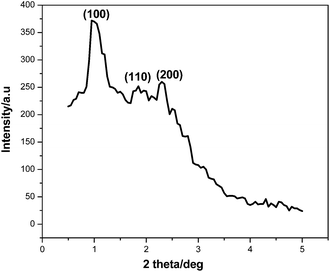 |
| Fig. 1 XRD spectrum of Cu@PMO NCs. | |
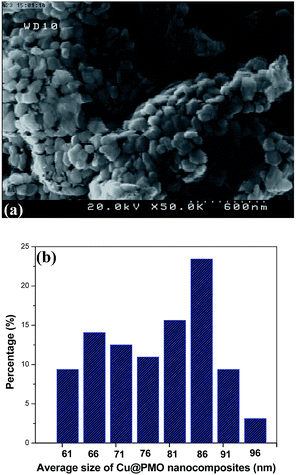 |
| Fig. 2 (a) FE-SEM images of Cu@PMO NCs. (b) Size distribution of Cu@PMO NCs framework according to FE-SEM data. | |
FT-IR confirms the presence of LCu (2) on PMO (Fig. 3). The C
N stretching vibration frequency of Cu@PMO NCs was observed at 1561 cm−1. The vibration bands at 2853 and 2923 cm−1 were assigned to the C–H stretching vibrations of aromatic and aliphatic groups, respectively. The vibration peak at 1474 cm−1 was characteristic of N–C vibrations of the aromatic functional groups present in the Cu@PMO NCs. The band in the range 1000–1100 cm−1 was assigned to Si–O–Si groups and the band at 961 cm−1 was attributed to Si–OH groups. A broad band at 3367 cm−1 was assigned to the O–H stretching vibrations of surface silanols on Cu@PMO NCs.
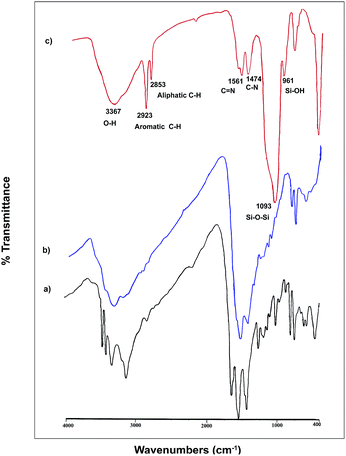 |
| Fig. 3 FT-IR spectra of (a) ligand, (b) LCu and (c) Cu@PMO NCs. | |
For Cu@PMO NCs, the EDS results were as follows (%): C, 18.42; N, 7.09; Cu, 3.43; Si, 24.73; O, 49.34 (Fig. 4). The EDS analysis confirmed the presence of LCu on PMO. Based on EDS, the amount of copper loading on Cu@PMO NCs was 0.52 mmol Cu g−1. In addition, AAS analysis indicated the presence of Cu in the catalyst and the content of Cu was estimated to be 0.47 mmol g−1. The presence of copper on the PMO framework was confirmed by two methods of analysis.
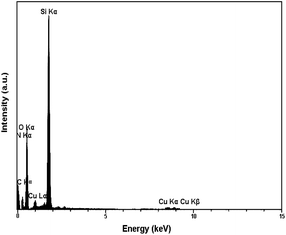 |
| Fig. 4 EDS pattern of Cu@PMO NCs. | |
The oxidation state of Cu on the PMO was determined by the UV-vis spectrum of LCu (2) and Cu@PMO CPs, as shown in Fig. 5. This spectrum showed a clear shift from 267 to 348 nm in accordance with the formation of Cu(II) during the addition of the ligand. Comparing the UV-vis spectra for LCu (2) and Cu@PMO CPs (diffuse reflective spectrum) confirmed the presence of the anchored LCu (2) on the PMO (Fig. 5a). To verify the oxidation state of copper in the complex on the PMO framework, the electrochemical properties of Cu(OAc)2·H2O and Cu@PMO CPs were investigated. In this experiment, the cyclic voltammograms of Cu(OAc)2·H2O and Cu@PMO CPs in 0.1 M KCl as the supporting electrolyte was recorded with a scan rate of 100 mV s−1 using a glassy carbon working electrode (GCE; Fig. 5b). The complex (1 mg) was dispersed in water (100 μL) to provide a suspension. Next, the suspension (5 μL) was dropped on a clean GCE and allowed to dry at room temperature. The cyclic voltammograms of 0.1 μM Cu(OAc)2·H2O was carried out in a supporting electrolyte. The curve for Cu(OAc)2·H2O exhibits one peak (Ec = −0.37 V vs. Ag/AgCl) corresponding to the one-electron reduction of Cu(II) and the formation of Cu(I). Moreover, in accordance with the Cu@PMO CPs curve, the reduction of Cu(II) on the PMO was negatively shifted (Ec = −0.41 V vs. Ag/AgCl) compared with the curve for Cu(OAc)2·H2O. Unexpectedly, these results reveal that Cu(OAc)2·H2O and Cu@PMO CPs show a partial cathodic shift.
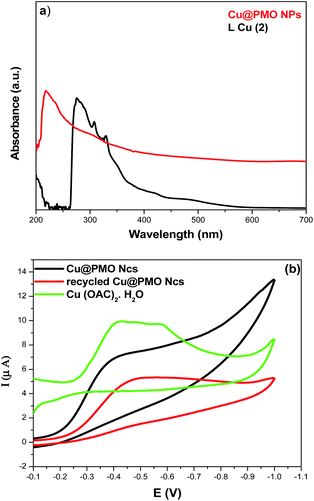 |
| Fig. 5 (a) UV-vis spectra of Cu@PMO NCs and LCu (2). (b) Cyclic voltammograms of Cu@PMO NCs, recycled Cu@PMO NCs and Cu(OAc)2·H2O. | |
However, several bonds were formed between Cu(II) and the oxygen and nitrogen of the ligand, which was probably the main reason for the negative shift for the complex compared with that of Cu(OAc)2·H2O. Furthermore, the oxidation state of Cu(II) remained after the reaction; therefore, a cyclic voltammetry study of the recycled catalyst was performed (Fig. 5). The cyclic voltammogram of recycled Cu@PMO CPs was similar to that of as synthesised Cu@PMO CPs, although its reduction was negatively shifted (E = −0.49 V vs. Ag/AgCl) compared with the voltammogram for Cu(OAc)2·H2O.
Typical N2 adsorption/desorption type IV patterns with H3 hysteresis loops,37 which is characteristic of highly ordered mesoporous materials, were obtained for Cu@PMO NCs. The BET specific surface area and a total pore volume were 462.40 m2 g −1 and 0.5 cm3 g−1, respectively (Fig. 6a). The pore diameter was calculated according to the BJH model to be 3.98 nm (Fig. 6b).
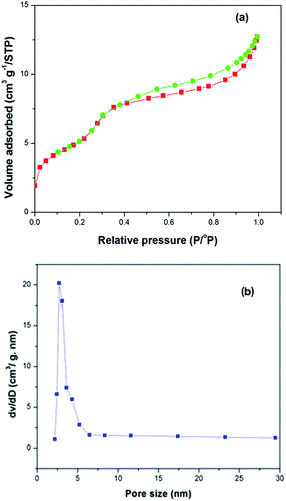 |
| Fig. 6 N2 adsorption–desorption isotherm (a) and pore size distribution curve (b) of Cu@PMO NCs. | |
TGA of Cu@PMO NCs shows two major weight losses at 25–800 °C in a N2 atmosphere (Fig. 7). A weight loss of less than 3% between 100–250 °C was observed, probably arising from physically adsorbed water. The sharp weight loss from 300–800 °C results from the decomposition of LCu (2)-bridging groups, suggesting a stable organic–inorganic composite framework (22%). In addition, TGA analysis indicated that the prepared Cu@PMO NCs catalyst has high thermal stability and negligible LCu (2) leaching up to about 300 °C. Based on TGA analysis, the loading of LCu (2) on the Cu@PMO NCs nanocomposites catalyst was 52.62 mmol LCu g−1.
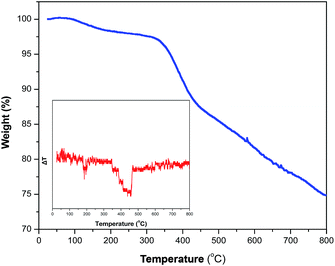 |
| Fig. 7 TGA-DTA thermograms of the Cu@PMO NCs catalyst. | |
Experimental design for β-hydroxy-1,2,3-triazole (3a)
To optimize conditions for producing β-hydroxy-1,2,3-triazole (3a), a series of experiments were performed with the standard reaction of styrene oxide, phenylacetylene and sodium azide as a model reaction (Scheme. 2). To evaluate the effect of ultrasound irradiation on β-hydroxy-1,2,3-triazole (3a) production using the Cu@PMO NCs as the catalyst, the effect of different variables such as ultrasound irradiation frequency, Cu@PMO NCs amount (%) and ultrasound time (min) in the three-component reaction were investigated. The influence of these variables was observed through an experimental BBD using the surface response.
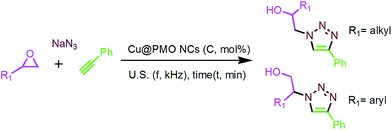 |
| Scheme 2 Three-component synthesis of β-hydroxy-1,2,3-triazoles. | |
Effects of major variables
The effect of the main variables on the reaction were evaluated based on the p values (Table 2). As shown in Table 2, the amount of Cu@PMO NCs had a positive effect on β-hydroxy-1,2,3-triazole (3a) yield (6.00). Ultrasound irradiation frequency had a small effect (0.375) on the reaction catalyzed by Cu@PMO NCs. The duration of the reaction also had a small effect (0.125).
Table 2 Effect of the parameter estimates for the β-hydroxy-1,2,3-triazole (3a) production
Variables |
Effect |
Standard error |
p-Value |
Mean |
98.000 |
1.103 |
<0.0001 |
Cu@PMO NCs content (C) |
6.000 |
0.675 |
<0.0001 |
Ultrasound irradiation frequency (F) |
0.375 |
0.675 |
0.603 |
Ultrasound time (t) |
0.125 |
0.675 |
0.860 |
C × C |
−6.37 |
0.994 |
0.001 |
F × F |
−5.12 |
0.994 |
0.004 |
t × t |
−4.12 |
0.994 |
0.009 |
C × F |
−1.500 |
0.955 |
0.177 |
C × t |
−0.000 |
0.955 |
1.000 |
F × t |
−4.250 |
0.955 |
0.007 |
The effect of variables on the β-hydroxy-1,2,3-triazole (3a) production under ultrasound irradiation catalyzed by Cu@PMO NCs can be observed clearly in the surface response graphs that are shown in Fig. 8. Fig. 8a shows the variation of yield of β-hydroxy-1,2,3-triazole (3a) caused by varying the amount of catalyst and ultrasound irradiation frequency. The yield increased rapidly to an optimum value with an increase in catalyst amount and thereafter declined slightly as the microwave power increased. The amount of catalyst had a greater effect than the ultrasound irradiation frequency, and the interaction between these two variables was significant. Fig. 8b shows the correlation between the amount of catalyst and the ultrasound time. As the ultrasound time increased, the response improved dramatically to the highest value and decreased thereafter. The decrease might be caused by degradation of β-hydroxy-1,2,3-triazole (3a) in the later reaction stage. Based on the response surface, the yield of β-hydroxy-1,2,3-triazole (3a) was more sensitive to the amount of catalyst than to the ultrasound time. The yield of β-hydroxy-1,2,3-triazole (3a) as a function of ultrasound irradiation frequency and ultrasound time is presented in Fig. 8c. The features of the graph and the results in Table 2 indicate that ultrasound irradiation frequency was more important than ultrasound time.
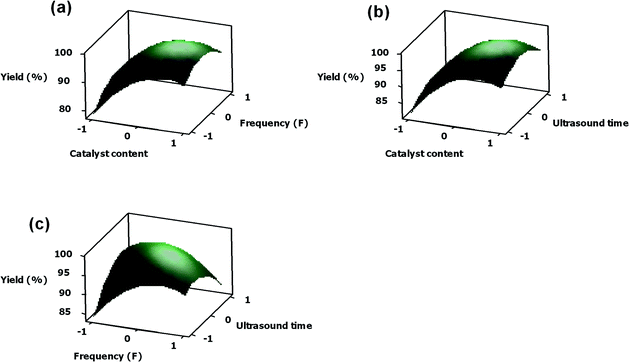 |
| Fig. 8 Fitted response surface for the reaction: (a) amount of catalyst and frequency irradiation, (b) amount of catalyst and ultrasound time and (c) frequency irradiation and ultrasound time. | |
Based on the quadratic model, the optimum yield of β-hydroxy-1,2,3-triazole (3a) catalyzed by Cu@PMO NCs under ultrasound irradiation frequency was predicted to be 99% under the following conditions: amount of catalyst of 0.007 g (0.33 mol%), ultrasound irradiation frequency of 40 kHz and ultrasound time of 7 min (Table 1, entry 1).
The quadratic model that predicts the relationship between the yield of β-hydroxy-1,2,3-triazole (3a) (y) with independent variables is expressed by eqn (1)
|
y = 89.00 + 6.00C + 0.37F + 0.12t − 6.37C2 − 5.12F2 − 4.12t2 − 1.50C × F − 4.25F × t
| (1) |
where
y is the percentage of yield conversion, and
C,
F, and
t are the coded values of Cu@PMO NCs content, irradiation frequency and ultrasound time, respectively.
Heterogeneity test
Any leaching of the active species from the support would make the catalyst unattractive; thus, it was necessary to study the stability and leaching of LCu (2) from the support. For the rigorous proof of heterogeneity, the catalyst was filtered from the reaction mixture under ultrasound irradiation after 3.5 min and the filtrate was allowed to react up to 15 min. After 3.5 min, the solid product and catalyst were separated from the reaction mixture by dissolving in hot methanol to give the corresponding product 3a in 47% yield. After 15 min, no solid product was observed in the reaction mixture. Based on these results, it can be concluded that there is no leaching of LCu (2) species from the support PMO.
In addition, leaching of Cu from Cu@PMO NCs was checked by AAS analysis of the filtrate, which showed there was no deactivation of the catalyst. Hence, this analysis strongly suggests there was no leaching of Cu@PMO NCs, and the present catalyst is truly heterogeneous in nature.
Recycling the catalyst
One of the main aims of this work was to develop a heterogeneous catalyst that was easy to recover and recycle. We further explored the reusability of the catalyst in the model reaction with treatment of the styrene oxide, phenylacetylene, and NaN3 in the presence of 0.33 mol% Cu@PMO NCs catalyst under ultrasound irradiation (40 kHz) in H2O at room temperature for 7 min. The catalyst was separated easily and completely from the reaction mixture by simple filtration, washing with double distilled water and drying at 100 °C in an oven for 3 h. The recovered catalyst was then used for a further run. The corresponding results indicated that this simple separation method could be repeated for six consecutive runs and the recovered catalyst showed remarkably constant catalytic activity in all six cycles (Fig. 9).
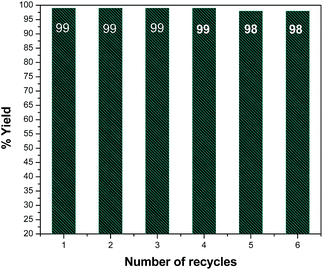 |
| Fig. 9 Recycling of the Cu@PMO NCs catalyst. | |
Comparison with reported catalysts
We compared the reactivity of the Cu@PMO NCs with a variety of copper catalysts under standard conditions for 1,3-dipolar cycloaddition of styrene oxide, sodium azide, and phenylacetylene. Table 3 shows that much higher yields were obtained for β-hydroxy-1,2,3-triazoles using our catalyst, and the catalyst amount and copper loading (0.33 mol%, 0.007 g) were low compared with the other catalysts (Table 3, entries 2 and 3) and commercial copper (Table 3, entry 1).
Table 3 Three-component synthesis of β-hydroxy-triazoles catalyzed by copper on different supports
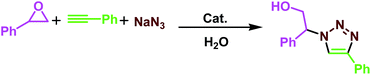
|
Entry |
Catalyst |
Catalyst amount (mol%) |
t (°C) |
T (h) |
Yield (%) |
Zeolite loading of 20 mg (ca. 0.08 mmol Cu(I)). Ultrasound irradiation (40 kHz), ultrasound time, 7 min. |
1 |
Cu(OAc)2·H2O |
10 |
70 |
24 |
0 |
2 |
CuI-Zeolitea |
8 |
25 |
20 |
77 |
3 |
Cu@phosphorated SiO2 |
0.64 |
60 |
1 |
94 |
4 |
Cu@PMO NCsb |
0.33 |
25 |
0.12 |
99 |
High efficiency and generality of the synthesis of β-hydroxy-1,2,3-triazoles under optimized conditions
After identifying the most efficient catalyst amount (0.33 mol%), ultrasound time (7 min) and ultrasound irradiation frequency (40 kHz) to determine the role of ultrasound, we also developed an experimentally convenient one-pot regioselective synthesis of β-hydroxy-1,2,3-triazoles from epoxides, sodium azide, and phenylacetylene in water at room temperature. Ultrasonic-assisted chemistry was used because of the efficiency of the interaction of ultrasound irradiation with the nanocatalyst. When the reaction was carried out under optimized conditions (frequency, 40 kHz); Cu@PMO NCs, 0.33 mol%; and ultrasound time, 7 min), it gave excellent yields in a short ultrasound time. The results are summarized in Table 4. For aryl-substituted epoxides, the yield was high and the reaction was complete within 7 min (Table 4, entries 1 and 2). The reaction of the alkyl epoxides in this procedure showed lower yields (Table 4, entries 3–10), and cyclooctene oxide (1f) and cyclododecene oxide (1g) did not react and remained unchanged even after extended ultrasound irradiation and ultrasound time until 8 h (Table 4, entries 11 and 12). Furthermore, the results show that Cu@PMO NCs is a highly efficient catalytic system for the cycloaddition of alkyl and aryl epoxides with phenylacetylene.
Table 4 Three-component synthesis of β-hydroxy-1,2,3-triazoles from epoxides 1a–m, phenylacetylene, and sodium azide, catalyzed by Cu@PMO NCs in watera
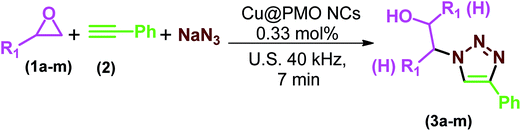
|
Entry |
Epoxide |
Triazole |
Yieldb (%) |
TONc/TOFd |
Reaction conditions: epoxide (1 mmol), phenylacetylene (1 mmol), NaN3 (1.1 mmol), Cu@PMO NCs (0.007 g, 0.33 mol% Cu), H2O, ultrasound irradiation (40 kHz), room temperature, ultrasound time of 7 min. Isolated yields. TON: mole of β-hydroxy-1,2,3-triazole formed per mole of catalyst. TOF : TON per time (min). The reaction was done with a 1 : 2 : 2 molar ratio of epoxide : alkyne : NaN3. |
1 |
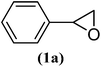 |
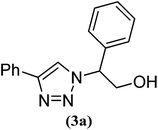 |
99 |
341.37/48.76 |
2 |
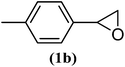 |
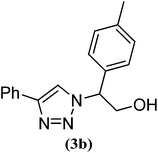 |
99 |
341/48.76 |
3 |
 |
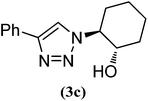 |
86 |
296.55/42.36 |
4 |
 |
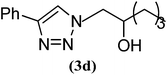 |
80 |
275.86/39.40 |
5 |
 |
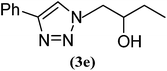 |
86 |
296.55/42.36 |
6 |
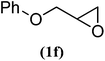 |
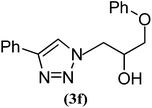 |
89 |
306.89/43.84 |
7 |
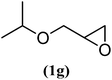 |
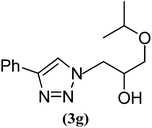 |
79 |
272.41/38.91 |
8 |
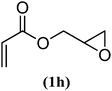 |
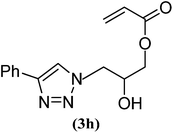 |
76 |
262.06/37.43 |
9 |
 |
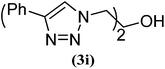 |
74 |
255.17/36.45 |
10 |
 |
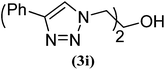 |
78 |
268.95/38.42 |
11e |
 |
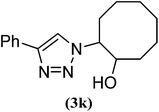 |
— |
— |
12 |
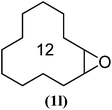 |
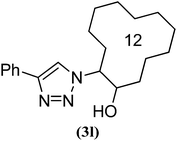 |
— |
— |
The proposed mechanism for the formation of β-hydroxy-1,2,3-triazoles involves two pathways, in which Cu@PMO NCs has a twofold catalytic role as a bifunctional catalyst, which combines one-pot ring opening and 1,3-dipolar cycloaddition. First, the participation of a metal azide as the catalytically active species suggests that the mechanism of epoxide ring opening involves azide delivery from the catalyst in addition to epoxide activation by the Cu@PMO NCs catalyst and formation of the organoazide. However, the π-complex formation between the phenylacetylene and Cu@PMO NCs to afford the Cu(II) acetylide, followed by the 1,3-dipolar cycloaddition between the Cu(II) acetylide and the organoazide, and final protonolysis of the intermediate would produce the β-hydroxy-1,2,3-triazole product. Moreover, the cyclic voltammetry studies for the Cu@PMO NCs catalyst (Fig. 5b) before and after the reaction confirmed the 2 + oxidation state for Cu. However, other studies reported that Cu(II) functioned as a catalyst for the click reaction with no reducing agent.16,38
Conclusions
We have synthesized and characterized Cu@PMO NCs, and tested its catalytic activity. Furthermore, we used a BBD to provide data to model the effects of the amount of catalyst, ultrasound irradiation frequency and ultrasound time on β-hydroxy-1,2,3-triazole yields. The preparation and characterization of a copper catalyst based on ligand-functionalized PMO were described. The Cu@PMO NCs are a highly efficient, reusable catalyst for the synthesis of β-hydroxy-1,2,3-triazoles. The mathematical model presented a satisfactory representation of the process and a good correlation between theoretical values provided by the model equation and experimental results. Thus, BBD was used to evaluate the optimized reaction conditions, and can be used to obtain the maximum yield with the minimum number of experiments and to save time. We developed a quick, highly efficient method for copper-catalyzed cycloaddition of aryl/alkyl epoxides and alkynes in water at room temperature. The reaction is a promising candidate for green chemistry because it is simple, uses water, occurs at ambient temperature under ultrasound irradiation, produces excellent yields, does not need chromatographic purification, has short reaction times, and the catalyst has a high capacity and recyclability.
Acknowledgements
The authors are grateful to University of Kashan for supporting this work by Grant no. 159148/39.
References
- V. Rebbin, R. Schmidt and M. Fröba, Angew. Chem., Int. Ed., 2006, 45, 5210–5214 CrossRef CAS PubMed.
- C. V. Polshettiwar, C. Len and A. Fihri, Chem. Rev., 2009, 253, 2599 Search PubMed.
- H. H. Yang, S. Q. Zhang, W. Yang, X. L. Chen, Z. X. Zhuang, J. G. Xu and R. X. Wang, J. Am. Chem. Soc., 2004, 126, 4054–4055 CrossRef CAS PubMed.
- G.-Z. Fang, J. Tan and X.-P. Yan, Anal. Chem., 2005, 77, 1734–1739 CrossRef CAS PubMed.
- Y.-K. Lu and X.-P. Yan, Anal. Chem., 2004, 76, 453–457 CrossRef CAS PubMed.
- M. C. Bruzzoniti, A. Prelle, C. Sarzanini, B. Onida, S. Fiorilli and E. Garrone, J. Sep. Sci., 2007, 30, 2414–2420 CrossRef CAS PubMed.
- R. Ouyang, J. Lei and H. Ju, Chem. Commun., 2008, 44, 5761–5763 RSC.
- K. Haupt, Anal. Chem., 2003, 75, 376–383 CrossRef.
- G. Wuff, Chem. Rev., 2002, 102, 1–27 Search PubMed.
- F. L. D. A. Mujahid and P. A. Lieberzeit, Materials, 2010, 3, 2196–2217 CrossRef PubMed.
- M. S. Moorthy, P. K. Tapaswi, S. S. Park, A. Mathew, H. Cho and C. Ha, Microporous Mesoporous Mater., 2013, 180, 162–171 CrossRef CAS PubMed.
- R. Huisgen, Pure Appl. Chem., 1989, 61, 613–628 CrossRef CAS.
- R. Huisgen, G. Szeimies and L. Moebius, Chem. Ber., 1965, 98, 4014–4021 CrossRef CAS.
- V. V. Rostovtsev, L. G. Green, V. V. Fokin and K. B. Sharpless, Angew. Chem., Int. Ed., 2002, 41, 2596–2599 CrossRef CAS.
- K. R. Reddy, C. U. Maheswari, M. L. Kantam and P. C. Division, Synth. Commun., 2008, 38, 2158–2167 CrossRef CAS.
- N. Mukherjee, A. Sabir, S. Bhadra and B. C. Ranu, Green Chem., 2013, 15, 389–397 RSC.
- F. Wang, H. Fu, Y. Jiang and Y. Zhao, Green Chem., 2008, 10, 452 RSC.
- L. S. Campbell-Verduyn, W. Szymański, C. P. Postema, R. A. Dierckx, P. H. Elsinga, D. B. Janssen and B. L. Feringa, Chem. Commun., 2010, 46, 898–900 RSC.
- R. B. Nasir Baig and R. S. Varma, Green Chem., 2012, 14, 625 RSC.
- P. Appukkuttan, W. Dehaen, V. V. Fokin and E. Van Der Eycken, Org. Lett., 2004, 6, 4223–4225 CrossRef CAS PubMed.
- J. García-Álvarez, J. Díez and J. Gimeno, Green Chem., 2010, 12, 2127 RSC.
- T. Miao and L. Wang, Synthesis, 2008, 3, 363–368 Search PubMed.
- F. Alonso, Y. Moglie, G. Radivoy and M. Yus, J. Org. Chem., 2011, 76, 8394–8405 CrossRef CAS PubMed.
- F. Alonso, Y. Moglie, G. Radivoy and M. Yus, Adv. Synth. Catal., 2010, 352, 3208–3214 CrossRef CAS.
- T. Boningari, A. Olmos, B. M. Reddy, J. Sommer and P. Pale, Eur. J. Org. Chem., 2010, 33, 6338–6347 CrossRef.
- H. Naeimi and V. Nejadshafiee, New J. Chem., 2014, 38, 5429–5435 RSC.
- H. Sharghi, M. H. Beyzavi, A. Safavi, M. M. Doroodmand and R. Khalifeh, Adv. Synth. Catal., 2009, 351, 2391–2410 CrossRef CAS.
- H. Sarvari, M. Moeini, F. Khalifeh, R. Beni and A. Salimi, Helv. Chim. Acta, 2010, 93, 435–449 CrossRef.
- M. Ashokkumar and T. J. Mason, Encyclopedia of Chemical Technology, 2007 Search PubMed.
- J. K. Kim, F. Martinez and I. S. Metcalfe, Catal. Today, 2007, 124, 224–231 CrossRef CAS PubMed.
- M. Driowya, A. Puissant, G. Robert, P. Auberger, R. Benhida and K. Bougrin, Ultrason. Sonochem., 2012, 19, 1132–1138 CrossRef CAS PubMed.
- B. A. Dar, A. Bhowmik, A. Sharma, P. R. Sharma, A. Lazar, A. P. Singh, M. Sharma and B. Singh, Appl. Clay Sci., 2013, 81, 351–357 CrossRef PubMed.
- K. M. Goncalves, F. K. Sutili, S. G. F. Leite, R. O. M. A. Souza and R. C. I. Leal, Ultrason. Sonochem., 2012, 19, 232–236 CrossRef CAS PubMed.
- A. Tsirk, S. Gronowitz and A. B. Hiirnfeldt, Tetrahedron, 1998, 54, 1817–1834 CrossRef CAS.
- J. S. Yadav, B. V. S. Reddy, G. M. Reddy and D. N. Chary, Tetrahedron Lett., 2007, 48, 8773–8776 CrossRef CAS PubMed.
- T. Boningari, A. Olmos, B. M. Reddy, J. Sommer and P. Pale, Eur. J. Org. Chem., 2010, 33, 6338–6347 CrossRef.
- G. Leofanti, M. Padovan, G. Tozzola and B. Venturelli, Catal. Today, 1998, 41, 207–219 CrossRef CAS.
- K. Namitharan, M. Kumarraja and K. Pitchumani, Chem.–Eur. J., 2009, 15, 2755–2758 CrossRef CAS PubMed.
Footnote |
† Electronic supplementary information (ESI) available. See DOI: 10.1039/c4ra17229a |
|
This journal is © The Royal Society of Chemistry 2015 |