DOI:
10.1039/C4RA16997B
(Paper)
RSC Adv., 2015,
5, 25348-25356
Effect of the functional diamine structure on the properties of a polyimide liquid crystal alignment film
Received
24th December 2014
, Accepted 2nd March 2015
First published on 2nd March 2015
Abstract
A novel functional diamine containing triphenylamine moiety and biphenyl as well as a long alkyl chain, 4-dodecyloxy-biphenyl-4′,4′′-diaminotriphenylamine (DBDTA), was synthesized and characterized. A series of polyimides (PIs) were copolymerized from DBDTA, 3,3′-dimethyl-4,4′-methylenedianiline (DMMDA) and 4,4′-oxydiphthalic anhydride (ODPA) via a one-step method. The chemical structures of the diamine and PIs were characterized by Fourier transform infrared spectroscopy (FT-IR) and nuclear magnetic resonance spectroscopy (1H NMR). Properties such as the solubility, rubbing resistance, thermal stability and pre-tilt angle of the PIs were investigated. Furthermore, the results were compared with the PIs-BZA derived from 4-dodecyloxy-biphenyl-3′,5′-diaminobenzoate (DBPDA). The PIs-TPA derived from DBDTA exhibited better transparency and thermal stability than the PIs-BZA from DBPDA. When the content of functional diamines was only 10%, the PI1-TPA derived from DBDTA displayed better solubility than the PI1-BZA from DBPDA. In addition, all PIs could induce uniform vertical alignment of the liquid crystals (LCs) before and after the rubbing process, but PI1-BZA only induced parallel alignment of the LCs after rubbing process. It is suggested that the PI1-TPA film is more resistant to the rubbing process than PI1-BZA.
1. Introduction
Aromatic polyimides (PIs) are widely used in many fields, such as membrane materials for separation,1,2 composites,3–5 coatings6 and liquid crystal (LC) alignment layers in liquid crystal displays (LCDs)7,8 because of their excellent mechanical and thermal stability.
One of the key technologies in LCDs is surface alignment of LCs. The pre-tilt angle of LC molecules is a sector angle between the LC molecule and substrate that influences the electro-optic characteristics of LCDs, such as bias voltage, threshold voltage, and response time.9 Due to their rigid chain characteristics, traditional aromatic PIs usually exhibit very low solubility and a high melting point far above their decomposition temperature. Thus, PI processing is generally carried out with polyamic acid (PAA) intermediate and then converted to PI via a rigorous thermal treatment (250–300 °C), which is fatal to the substrate used in the LCD. This disadvantage can be overcome by introducing bulky groups,10 flexible linkages,11 spiroskeleton linkage12 and asymmetric units13 into the backbone or copolymerization.14 Though these attempts could create a more soluble modified PI, main-chain-type PIs are still unable to satisfy the requirements of manufacturing high-performance LCDs due to their small pretilt angles. Recently, it was reported that LCDs with vertical aligned mode had a faster response time and a higher contrast ratio compared to that with parallel aligned mode. PIs containing alkyl side chains exhibited good solubility and high pretilt angle, however, the thermal stability of these PIs was low. To the best of our knowledge, the research on the preparation of soluble, thermally stable and rubbing resistance PI for vertical alignment of LC has rarely been reported. Many works have been carried out on the effect of length of alkyl groups on pretilt angles, but only a few papers have discussed the effect of the skeleton structures of diamine.15
In this study, we have synthesized a functional diamine 4-dodecyloxy-biphenyl-4′,4′′-diaminotriphenylamine (DBDTA). Some PIs were synthesized from 3,3′-dimethyl-4,4′-methyl enedianiline (DMMDA), 4,4′-oxydi(phthalic-anhydride) (ODPA) and DBDTA. Other PIs were synthesized from DMMDA, ODPA and functional diamine 4-dodecyloxy-biphenyl-3,5-diaminobenzoate (DBPDA). Then the solubility, transmittance and thermal stability of the PIs were investigated. Meanwhile, alignment ability and pretilt angles of LCs induced by the PIs were also studied. The structure geometries of two functional diamines were optimized by the density functional theory (DFT) calculations using a large basis-set function which was shown in Fig. 1. As shown in Fig. 1, the lengths of two functional diamines (DBDTA and DBPDA) were 27.0 and 29.2 Å, respectively, that is, their length was almost the same. And an effect of the skeleton structures of the functional diamines on the pretilt angles of the LCs before and after rubbing process has been investigated.
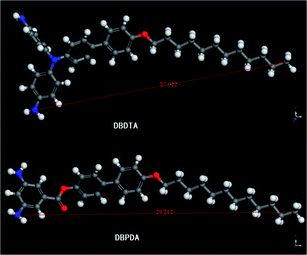 |
| Fig. 1 Spatial diagram of two functional diamines. Gray, white, red and blue of each molecular structure display C, H, O and N, respectively. | |
2. Experimental section
2.1 Materials
4-Hydroxydiphenyl, benzoylchloride, 4-fluoronitrobenzene, fuming nitric acid and cesium fluoride were purchased from Aladdin Chemical Reagent Corp. (shanghai, China). Hydrazine hydrate was purchased from Haihong Experimental Instrument Co., Ltd (Chengdu, China). ODPA (>98%, Shanghai Research Institute of Synthetic Resins) was purified by a recrystallization from acetic anhydride. DMMDA was purchased from Shanghai EMST Corp. (Shanghai, China) and dried before use. DBPDA was synthesized according to our previous work.16 N-Methyl-2-pyrrolodone (NMP), N,N-dimethyl acetamide (DMAc), dimethyl sulfoxide (DMSO), N,N-dimethylformamide (DMF) and chloroform (TCM) were purchased from Bodi Chemical Reagents Corp. (Tianjin, China), and then purified by vacuum-distillation over phosphorus pentoxide. Ethanol, isopropyl alcohol and tetrahydrofuran (THF) were dried with 4 Å molecular sieves before use. 5CB was purchased from Shijiazhuang Crown Chem-Tech. Co. Ltd.
2.2 Measurements
Fourier transform infrared spectroscopy (FT-IR) was recorded on a Nicolet 560 Fourier transform spectrophotometer. Nuclear magnetic resonance (1H NMR) spectra were obtained from a 400 MHz Unity INVOA 400 spectrophotometer. Thermogravimetric analysis (TGA) was performed under nitrogen on a DuPont TGA2100 thermogravimetric analyzer and the sample was heated at 20 °C min−1. Differential scanning calorimetry (DSC) was conducted under nitrogen with Netzsch 204 DSC and the sample was heated at 10 °C min−1. The rubbing process was operated with a rubbing machine from TianLi Co. Ltd. (Chengdu, China). Spin-coat process was executed using a KW-4A spinner from institute of microelectronics of Chinese Academy of Science (Peking, China). The pretilt angles of LCs were measured by crystal rotation method using a pretilt angle tester from Changchun Institute of Optics, Fine Mechanics and Physics (Changchun, China), and at least five different points on cells were selected for measurement. The optical micrographs were obtained from polarizing optical microscope (Shanghai Millimeter Precision Instrument Co. Ltd., Shanghai, China) under room temperature with the magnification of 40 times. The surface morphology of the PI films before and after rubbing was investigated by atomic force microscopy (AFM) operating in tapping mode using an instrument with a SPI4000 Probe Station controller (SIINT Instruments Co., Japan) at room temperature. Olympus tapping mode cantilevers with spring constants ranging from 51.2 N m−1 to 87.8 N m−1 (as specified by the manufacturer) were used with a scan rate in the range of 0.8–1.2 Hz.
2.3 Synthesis of monomer
The synthesis of DBDTA was shown in Fig. 2.
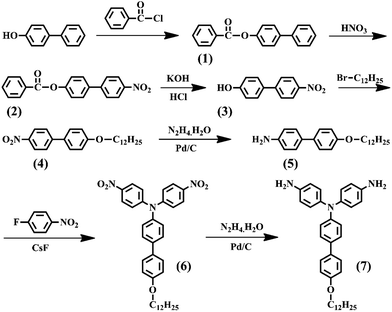 |
| Fig. 2 Synthesis of functional diamine (DBDTA). | |
2.3.1 Synthesis of 4-benzoyloxybiphenyl (1). A 500 ml flask equipped with a magnetic stirrer and a condenser was placed with a mixture of 200 ml of dry THF, 20.40 g (0.08 mol) of 4-hydroxybiphenyl and 16.4 ml (0.05 mol) of dry triethylamine (distilled from KOH). Then 12.2 ml (0.10 mol) of benzoylchloride was added dropwise into the mixed solution at 0 °C and the reaction mixture was stirred for 24 h at room temperature. And then the reaction mixture was filtered. THF and triethylamine were evaporated. The solid was washed with water and hot methanol, and recrystallized from 1-butanol to afford 20.41 g (yield: 93%) of white crystals. 1H NMR (DMSO-d6, δ), ppm: 8.14, 7.51, 7.41 (m, 5H, ArH), 7.55, 7.48, 7.32, 7.25, 7.21 (m, 9H, ArH of biphenyl).
2.3.2 Synthesis of 4-benzoyloxy-4′-nitrobipheny (2). 20.00 g (0.07 mol) of 4-benzoyloxybiphenyl (1) was mixed with 160 ml of glacial acetic acid and heated to 85 °C. Fuming nitric acid (48 ml) was slowly added dropwise into the mixture. The temperature was kept at 90 °C and the reaction mixture was stirred for 30 min. The reaction mixture was filtered when it was hot. The solid was washed with water and methanol, and recrystallized from glacial acetic acid to afford 10.52 g (yield: 45%) of light yellow crystals.17 1H NMR (DMSO-d6, δ), ppm: 8.34, 8.02, 7.92, 7.50 (m, 8H, ArH of biphenyl), 8.18, 7.78, 7.64 (m, 5H, ArH).
2.3.3 Synthesis of 4-hydroxy-4′-nitrobiphenyl (3). 9.98 g (0.03 mol) of 4-benzoyloxy-4′-nitrobipheny (2) was mixed with 60 ml of ethanol and heated to reflux. A solution of 6.01 g of KOH in 20 ml of water was added dropwise at reflux. The reaction mixture was refluxed for 30 min, and then cooled to room temperature. The resulting purple crystals were filtered and washed with THF to yield 8.35 g (yield: 98%) of potassium 4-hydroxy-4′-nitrobiphenyl. And then 4-hydroxy-4′-nitrobiphenyl could be obtained by dissolving the salt in water and adding 50/50 HCl/H2O. The yellow solid was filtered, washed with water, and recrystallized from ethanol. 1H NMR (DMSO-d6, δ), ppm: 8.27–7.03 (m, 8H, ArH of biphenyl), 5.50 (bs, 1H, hydroxy).
2.3.4 Synthesis of 4-dodecyloxy-4′-nitrobiphenyl (4). A round flask equipped with a magnetic stirrer and a condenser was placed with a mixture of 6.01 g (0.03 mol) of 4-hydroxy-4′-nitrobiphenyl (3), 7.11 g (0.03 mol) of 1-bromododecane, 3.92 g (0.03 mol) of potassium carbonate and 50.0 ml of dry DMF. Then reaction mixture was stirred for 7 h at 90 °C. The reaction mixture was cooled to room temperature, and then the mixture was poured in distilled water. The precipitate was collected by filtration and recrystallized from acetonitrile to afford 10.15 g (yield: 95%) of pale-yellow crystals. 1H NMR (CDCl3, δ), ppm: 8.27–7.01 (m, 8H, ArH of biphenyl), 4.00 (t, 2H, –O–CH2–), 1.46–1.86 (m, 20H, –(CH2)10–), 0.98 (t, 3H, CH3).
2.3.5 Synthesis of 4-dodecyloxy-4′-aminonitrobiphenyl (5). A flask equipped with a magnetic stirrer and a condenser was charged with a mixture of 7.73 g (0.02 mol) of 4-dodecyloxy-4′-nitrobiphenyl (4), 0.77 g of 5% palladium on activated carbon (Pd/C) and 40 ml of isopropyl alcohol. The mixture was heated to reflux and then 4.8 ml (0.08 mol) of 80% hydrazine hydrate was added dropwise into the mixture. After about 4 h, the reaction was stopped to allow the mixture to room temperature, the catalyst was filtered off, and then the solvent was evaporated under the reduced pressure. The crude product was recrystallized from ethanol to afford 6.53 g of white crystals (yield: 90%). 1H NMR (CDCl3, δ), ppm: 7.45–6.75 (m, 8H, ArH of biphenyl), 4.00 (s, 2H, NH2), 3.96 (t, 2H, –O–CH2–), 1.29–1.83 (m, 20H, –(CH2)10–), 0.98 (t, 3H, CH3).
2.3.6 Synthesis of 4-dodecyloxy-biphenyl-4′,4′′-dinitrotriphenylamine (6). A three-necked flask equipped with a magnetic stirrer, a nitrogen inlet and a distillation head was charged with a mixture of DMSO (40 ml), p-fluoronitrobenzene (4.23 g, 0.03 mol) and cesium fluoride (CsF) (4.93 g, 0.03 mol), heated and stirred at 150 °C. Then a solution of 5.46 g (0.02 mol) 4-dodecyloxy-4′-aminonitrobiphenyl (5) in 40 ml of DMSO was added dropwise. The reaction mixture was heated and stirred at 150 °C for 10 h. The reaction mixture was cooled and poured into a water–methanol (water
:
methanol = 1
:
1 (v/v)). The yellow precipitate was filtered, washed with water and dried. 7.65 g crude product was obtained (yield: 85%). The crude product was purified by recrystallization from CH2Cl2/methanol as yellow crystal.18 1H NMR (DMSO-d6, δ), ppm: 8.21, 7.26 (m, 8H, ArH), 7.75, 7.64, 7.31, 7.02 (m, 8H, ArH of biphenyl), 4.00 (t, 2H, –O–CH2–), 1.21–1.85 (m, 20H, –(CH2)10–), 0.85 (t, 3H, CH3).
2.3.7 Synthesis of 4-dodecyloxy-biphenyl-4′,4′′-diaminotriphenylamine (7). A flask equipped with a magnetic stirrer and a condenser was charged with a mixture of 6.03 g (0.01 mol) of 4-dodecyloxy-biphenyl-4′,4′′-dinitrotriphenylamine (6), 0.61 g of 5% palladium on activated carbon (Pd/C) and 40 ml of isopropyl alcohol. The mixture was heated to reflux and then 4.8 ml (0.08 mol) of 80% hydrazine hydrate was added dropwise into the mixture. After about 4 h, the reaction was stopped to allow the mixture to room temperature, the catalyst was filtered off and the solvent was evaporated under the reduced pressure. The crude product was separated out by the column chromatography (ethyl acetate
:
petroleum ether = 10
:
3 (v/v)). At last, 5.26 g (yield: 95%) of the light gray solid DBDTA was obtained. The FT-IR ν = 3399, 3334 (–NH2), 3037 (C–H), 2919, 2848 (–CH2–), 1603, 1500 (aromatics), 1326 (C–N–C), 1267, 1173 (C–O–C) and the 1H NMR spectrum was given in Fig. 3. 1H NMR (DMSO-d6, δ), ppm: 6.82, 6.54 (m, 8H, ArH), 7.45, 7.31, 6.91, 6.64 (m, 8H, ArH of biphenyl), 4.98 (s, 4H, NH2), 3.90 (t, 2H, –O–CH2–), 1.23–1.83 (m, 20H, –(CH2)10–), 0.85 (t, 3H, CH3).
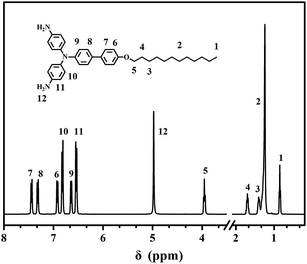 |
| Fig. 3 The 1H NMR spectrum (DMSO-d6) of DBDTA. | |
2.4 Representative procedure for the preparation of PIs
PIs were synthesized via one-step method (as shown in Fig. 4). A typical polymerization procedure was as follows: the diamine DBDTA (0.054 g, 0.1 mmol), ODPA (0.310 g, 1.0 mmol) and o-dichlorobenzene (10.0 ml) were added to a three-necked flask equipped with a stirrer, a nitrogen inlet and a distillation head. Isoquinoline (0.12 g) was added to the flask. After stirring at 80 °C for 1 h, the solution was heated to 180 °C and kept stirring for 2 h. When the mixture was cooled to room temperature, DMMDA (0.203 g, 0.9 mmol) was added. The mixture was reacted at 80 °C for 1 h, and then at 180 °C for 24 h. The resulting PI solution was poured into a large amount of ethanol with vigorous stirring. The precipitated PI was collected by filtration and then dried at 80 °C for 24 h under reduced pressure. The yield was 90–95%. Molar ratios of DBDTA to DMMDA were controlled to 1
:
9 (PI1-TPA), 2
:
8 (PI2-TPA), and 3
:
7 (PI3-TPA). Molar ratios of DBPDA to DMMDA were controlled to 1
:
9 (PI1-BZA), 2
:
8 (PI2-BZA), and 3
:
7 (PI3-BZA).
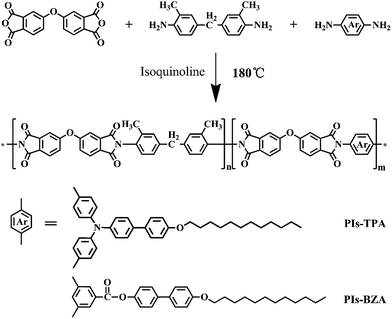 |
| Fig. 4 Synthetic route of the polymers. | |
2.5 Preparation of films and assembling of cells
The glass substrates were cut into 3.0 × 3.0 cm2 pieces. The PI solutions (5 wt% in NMP) were spin-coated onto indium tin oxide (ITO) glass substrates at 600 rpm for 15 s and 2500 rpm for 30 s. The PI films were baked at 120 °C for 2 h. Then the PI film was rubbed by a rubbing machine covered with a cotton velvet cloth. The definition of the rubbing strength, RS was given by
where N is the number of times the substrates were rubbed (N = 1 in this work), M is the depth of the fibers of the fabric deformed due to the pressed contact (0.3 mm), n is the rotational speed of the drum (700 rpm), v is the translational speed of the substrate (17.2 mm s−1) and r is the radius of the roller (22.5 mm).
LC cells were fabricated with two pieces of the rubbed substrates in an anti-parallel rubbing direction using a 40 μm thick spacer. In addition, some LC cells were manufactured with two pieces of the non-rubbed substrates. A nematic LC (5CB) was injected into the cell gap, followed by sealing of the injection hole with epoxy glue. The alignment behaviors of LC cells were examined through the whole cell by polarizing microscope. The pretilt angles of the LC cells were determined using a crystal rotation method.
3. Results and discussion
3.1 Characterization of PI structures
Traditionally, m-cresol was used as the polymerization solvent in the one-step method of PI polymerization. However, early work has speculated that the m-cresol may act as a nucleophile at the elevated temperature and displaced the carboxylate group from the benzoate position.19 Thus, the less polar o-dichlorobenzene was used as the solvent in the synthesis of the PIs. In this case, the PIs remained in solution and no side chain cleavage occurred.
The PI film samples were used for characterization by 1H NMR and FT-IR spectroscopy. 1H NMR spectra of PI2-BZA and PI2-TPA were shown in Fig. 5 and 6, respectively. The multiple peaks at 8.31–7.02 and 8.09–6.85 ppm are the characteristic peaks of the phenyl ring protons of the PI2-BZA and PI2-TPA, respectively. The single peak at 3.96 ppm is the characteristic peaks of methylene protons of the unit of DMMDA and alkyl ether. The single peak at 2.11 ppm is characteristic peaks of methyl protons of the unit of DMMDA. The multiple peaks at 1.78–0.89 and 1.75–0.84 ppm are the characteristic peaks of the alkyl chain protons of the PI2-BZA and PI2-TPA, respectively. Fig. 7 showed the FT-IR spectra of PI2-TPA and PI2-BZA films. Both spectra clearly showed the characteristic imide bands in the vicinity of 1776, 1723 and 1375 cm−1 and C–H stretching vibrations of aliphatic groups in 2924, 2850 cm−1.
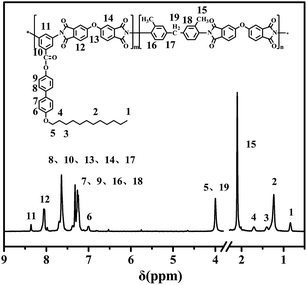 |
| Fig. 5 The 1H NMR spectrum (DMSO-d6) of PI2-BZA. | |
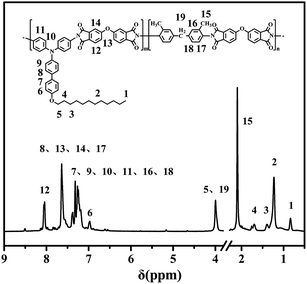 |
| Fig. 6 The 1H NMR spectrum (DMSO-d6) of PI2-TPA. | |
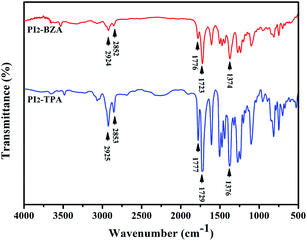 |
| Fig. 7 FT-IR spectra of PI2-TPA and PI2-BZA film. | |
3.2 UV-vis transmission of PIs
The PI resin was dissolved in NMP with stirring to give a homogeneous PI solution with a solid content of 10%. The PI solution was filtered by a 0.22 μm Teflon syringe filter and then spread onto a glass plate which was then thermally baked in an oven at 80 °C for 2 h, 120 °C for 1 h, 150 °C for 1 h and 180 °C for 1 h. The PI film was obtained by immersion the glass plate in water followed by drying in an oven at 100 °C. The UV-vis spectra of the PI films whose thickness is 20 μm were shown in Fig. 8. The PIs-TPA displayed better transparent than PIs-BZA and showed high transmissions (above 80%) in the wavelength range 500–800 nm. The good optical transparency and lighter color of the PI films resulted from the decrease in the intermolecular charge transfer complex (CTC) interaction and electron conjugation due to introduction the side group moieties and the pendant methyl group. Besides, the existence of ether units (–O–) and aliphatic units (–CH2–) in the PI backbone reduces intramolecular CTC interactions and improves optical transmittance. Furthermore, the rigid, twisted and non-coplanar triphenylamine (TPA) structures in the PI main chain can efficiently hinder the main chain dense packing and reduce intermolecular CTC interactions. Consequently, the PIs-TPA derived from DBDTA exhibited more excellent transparency than the PIs-BZA from DBPDA.
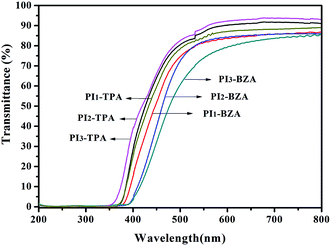 |
| Fig. 8 The UV-vis spectra of the PI films (film thickness: 20 μm). | |
3.3 Solubility of PIs
The solubility of the polymer was listed in Table 1. It could be found that PI1-TPA and PI1-BZA were partly soluble in the polar solvents, however, the PI2-TPA, PI2-BZA, PI3-TPA and PI3-BZA showed good solubility. They could not only easily dissolve in strong polar aprotic solvents, such as NMP, DMAc, DMSO, DMF, m-cresol, but also dissolve in low-boiling-point solvents, such as THF, TCM. It can be attributed to the presence of the long side groups, the pendant methyl group and the flexible ether in the polymer backbone which inhibit close packing and reduce the chain–chain interaction to enhance solubility.20 Comparing PI1-TPA with PI1-BZA, it was found that the solubility of PI1-TPA was better than that of PI1-BZA. The excellent solubility of PI1-TPA could be attributed to the existence of the twisted three-dimensional TPA structure that limited the chain packing, increased the free volume and caused solvent molecules to penetrate into the polymer chains.
Table 1 Solubility of the PIs in different solventsa
PI |
NMP |
DMSO |
DMF |
DMAc |
m-Cresol |
TCM |
THF |
(++) soluble at room temperature, (+) soluble upon heating at 60 °C, (+−) partial soluble upon heating at 60 °C. |
PI1-TPA |
++ |
++ |
++ |
+ |
+ |
++ |
+ |
PI2-TPA |
++ |
++ |
++ |
++ |
++ |
++ |
++ |
PI3-TPA |
++ |
++ |
++ |
++ |
++ |
++ |
++ |
PI1-BZA |
++ |
++ |
+ |
+− |
+− |
+ |
+− |
PI2-BZA |
++ |
++ |
++ |
++ |
++ |
++ |
++ |
PI3-BZA |
++ |
++ |
++ |
++ |
++ |
++ |
++ |
3.4 Thermal properties of PIs
Glass transitions temperature (Tg) of PIs was listed in Table 2. The Tg decreased with the increase in the content of functional diamine. Because the introduction of bulky side groups reduced intermolecular interactions and chain dense packing, the free volume in polymer systems increased and thus Tg decreased.21 Comparing PI with the same content of side chains, it could be found that Tg of PI containing DBDTA was lower than that of the PI containing DBPDA. The reason is that the introduction of non-coplanar TPA structure in the PI main chain would increase the free volume in polymer system.
Table 2 Thermal properties of the PIs
PI |
Tga (°C) |
Tdb (°C) |
T5b (°C) |
T10b (°C) |
R800c (%) |
Tg, glass transition temperature. Td, the onset thermal decomposition temperature of the PIs. T5 and T10 are the temperature at a 5% and 10% weight loss of the PIs, respectively. R800, weight percentage at 800 °C from TGA measurements. |
PI1-TPA |
240 |
384 |
493 |
523 |
60.5 |
PI2-TPA |
236 |
373 |
464 |
496 |
55.9 |
PI3-TPA |
227 |
365 |
423 |
471 |
38.9 |
PI1-BZA |
251 |
372 |
474 |
514 |
60.2 |
PI2-BZA |
242 |
369 |
451 |
488 |
45.8 |
PI3-BZA |
231 |
354 |
418 |
466 |
36.1 |
Thermal resistance was studied by TGA in N2. As shown in Fig. 9, all PIs showed two-step pyrolysis behavior with increasing temperature. This behavior may reasonably be presumed to arise from the fact that side chains are degraded in the lower-temperature range and the main chains are degraded in the higher-temperature range.22 Table 2 showed the thermal properties of the PIs with Td, T5 and T10. With an increase of the side chains content, the gradual decrease in Td, T5 and T10 indicated that thermal stability of PI was greatly affected by the side chain. Comparing PI containing the same content of side chains, it could be found that thermal stability of PIs-TPA with DBDTA was higher than that of PIs-BZA with DBPDA. The reason is that the PI with DBDTA side chain contained more benzene rings, thus, higher values of Td were observed. Furthermore, the PIs-TPA had higher T5 values than the corresponding PIs-BZA, which could be attributed to the fact that less of the overall weight of each PIs than its corresponding PIs-BZA derived from alkoxy units. The introduction of bulky, twisted TPA group into DBDTA increased the rigidity of the backbone of PIs; thus, higher values of T10 were observed.
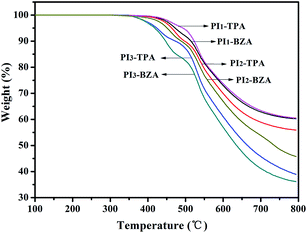 |
| Fig. 9 The TGA curves of the PIs. | |
3.5 Alignment ability and pretilt angle of LCs
3.5.1 Pretilt angle. As shown in Table 3, all PIs induced pretilt angle of 90° before rubbing process and uniform alignment of LCs. But after rubbing process, PIs with different composition of functional diamine could induce different pretilt angles of LCs. The pretilt angles increased with the content of functional diamine. With an increase in the content of the functional diamine, the density of side chains increased and the interaction of the side chains enhanced at the same time. The enhancement in interaction of the side chains increased the rigidity of the side chains and restricted its incline trend along the rubbing direction. After rubbing process, PI1-TPA could still induce LC vertical alignment, but PI1-BZA only induced LC parallel alignment. It is suggested that PI1-TPA film is more resistance to rubbing process than PI1-BZA.
Table 3 Pretilt angle of the PIs
PI |
Pretilt angle (°) |
Before rubbing |
After rubbing |
PI1-TPA |
90 |
89.5 |
PI1-BZA |
90 |
1.8 |
PI2-TPA |
90 |
89.7 |
PI2-BZA |
90 |
89.6 |
PI3-TPA |
90 |
89.8 |
PI3-BZA |
90 |
89.8 |
3.5.2 Alignment behavior. The alignment behaviors of the LC cells between crossed polarizers were examined with a polarizing optical microscope. As shown in Fig. 10a, an alternate and periodical change of transmittance with the rotation of the substrate stage could be observed, meaning that the LC molecules in cells using the rubbed PI1-BZA were aligned planar to the PI surface. Fig. 10b showed that the transmittance of the LC cells using the rubbed PI1-TPA with a rotation of the sample stage gave no rise to an alternate and periodic appearance of darkness and brightness, meaning that the LC molecules are aligned vertical to the PI surface. Conoscope observation of the cells was used with polarized optical microscope. As shown in Fig. 10b, dark crossed brush was seen clearly and not moved with the LC cell rotating before and after rubbing. The result further proved the vertical alignment was induced by PI1-TPA. The same result could be obtained by PI2-TPA, PI3-TPA, PI2-BZA and PI3-BZA.
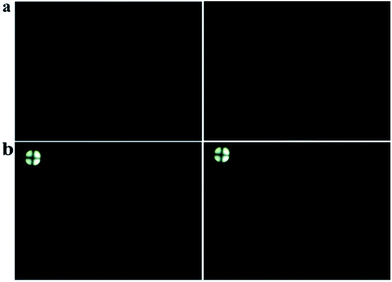 |
| Fig. 10 The optical micrographs on the left hand side were taken for LC cells using the rubbed PI1-BZA (a) PI1-TPA (b), the corresponding optical micrographs on the right hand side were taken for them after rotating the stage by 45°. | |
3.5.3 Surface morphology. Surface morphology of PI1-TPA and PI1-BZA films was measured by AFM before and after rubbing treatment. Parts A and B of Fig. 11 were shown AFM images of pristine PI1-TPA and PI1-BZA films, respectively. The PI1-TPA and PI1-BZA films were found to have a root-mean-square (RMS) roughness of 0.35 and 0.33 nm over an area of 2.5 × 2.5 μm2, respectively. The surface morphology and roughness of the PI film derive mainly from the characteristics of the polymer chains that govern the aggregation and molecular ordering that occur during the drying and thermal imidization processes after spin-casting.9 In contrast, the rubbed PI films displayed completely different morphology. As can be seen in Fig. 11C and D, both of the rubbed PI films exhibited groove-like structures and covered with sub-micrometer-scaled spikes along the rubbing direction. The microgroove lines that were generated might be due to the shear-induced deformation of the polymer film surface induced by the contact of fibers during the rubbing process. The spikes might be due to some degree of abrasion accompany the shear-induced PI deformation of the rubbing process. The developed microgrooves of the rubbed PI1-TPA and PI1-BZA films were around 35 nm or larger in size. The RMS surface roughness values of the rubbed PI1-TPA and PI1-BZA films were 0.94 and 1.37 nm over an area of 2.5 × 2.5 μm2, respectively.
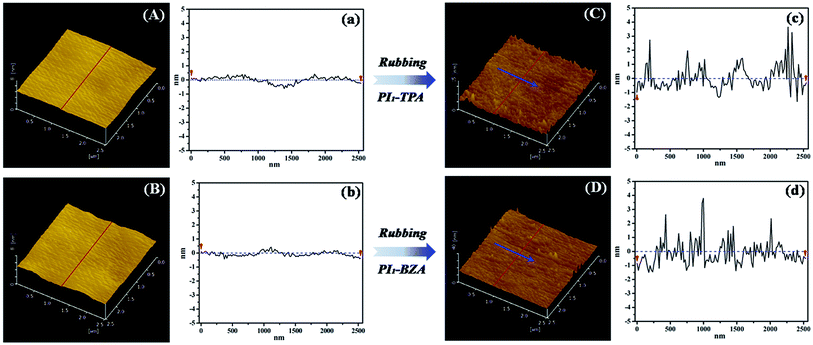 |
| Fig. 11 AFM images and surface profiles of PI films before and after rubbing: (A) and (a) pristine PI1-TPA PI; (B) and (b) pristine PI1-BZA PI; (C) and (c) rubbed PI1-TPA PI; (D) and (d) rubbed PI1-BZA PI. The arrows in the images (C) and (D) denote the rubbing direction, respectively. | |
First, the LC molecules on the PI1-TPA and PI1-BZA films with side chains containing rigid biphenyl groups could achieve vertical alignment without rubbing process. Second, although the PI1-TPA film had different morphologies before and after rubbing process, they could induce LC molecules vertical alignment. Third, both of the rubbed PI1-TPA and PI1-BZA films had the similar microgrooves, but they induced different alignment behaviors of LC molecules. Finally, the microgrooves developed along the rubbing direction are much larger in size than the LC molecules, which have dimensions of ca. 1.8 nm (length) and 0.25 nm (diameter). The dimensions of these surface structures seem to be large to effectively interact with and align LC molecules. These facts and the observed LC alignment suggested that the LC molecules are more likely to be aligned by interactions with the polymer chain segments, rather than by the microgrooves. Further, some reports had pointed out that there were some intermolecular interactions existing between LC molecules and the side chains of PIs, such as the dipole–dipole and π–π interaction between the biphenyl groups of LC molecules and the biphenyl groups of side chains, and the van der Waals forces between the alkoxy end-group of PI and the flexible alkoxy tail in an LC molecules. Due to the strong mutual interactions, LC molecules tend to be aligned in the direction parallel to the biphenyl groups of the side chains in the PI films.23,24 Therefore, it is believed that the molecular chain reorientation, especially biphenyl groups and alkoxy tails, had a greater influence on the LC aligning ability of the PI film surfaces than do the microgrooves. After rubbing process, PI1-TPA could still induce LC vertical alignment, but PI1-BZA only induced LC parallel alignment. The reason was that the rigid biphenyl groups in the side chain of PI1-TPA were directly linked to the backbone, which could restrain the lodging of the biphenyl group of the side chain in the rubbing process. However, the side chain of PI1-BZA containing flexible ester bond was easy to lodge along the rubbing direction when the density of side chain was low.
3.6 Molecular structures and molecular orbital energies
It is well known that the materials properties of PI alignment films are strongly depended on their molecular structure. In order to get a comprehensive understanding of the relationship between optical and rubbing resistance properties and polymer structure, the three-dimensional molecular structures of the model compounds for PIs were investigated. The structure geometries were optimized by the density functional theory [B3LYP/6-311G (d,p)] calculations using a large basis-set function.25 The molecular orbital energies of these model compounds were determined by molecular orbital (MO) calculation.
Fig. 12 depicted the optimized molecular structures of the model compounds for PI1-TPA and PI1-BZA. Meanwhile, the calculated molecular orbital energies were listed in Table 4, it is found that the PI1-TPA exhibited the much higher εLUMO values and smaller Δε values than PI1-BZA. In general, the large energy gap is favorable for preventing the CTC formation. However, the optical transparency of PI1-TPA is higher than PI1-BZA. It is presumed that the incorporation of the non-coplanar TPA structure in PI1-TPA backbone no longer allows to dense chain stacking, which decreases inter-molecular CTC interactions. In addition, as shown in Fig. 12, the dihedral angle between benzene ring and imide ring of PI1-TPA and PI1-BZA are 46.9 and 35.3°, respectively. It is suggested that the backbone of PI1-TPA is more twisted, which is capable of efficiently hindering the chain packing and decreasing electron conjugation and intra-molecular CTC interactions. It is further supported that PI1-TPA has better solubility and optical transparency than PI1-BZA.
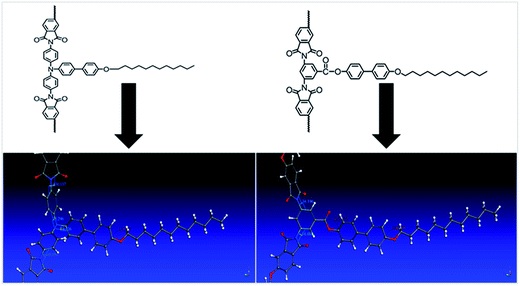 |
| Fig. 12 Molecular structure of the model compounds for PI1-BZA and PI1-TPA. Gray, white, red and blue color unit display C, H, O and N atom, respectively. | |
Table 4 Molecular orbital energies of HOMO and LUMO as well as energy gaps of aromatic PIs
PIs |
εLUMOa (eV) |
εHOMOb (eV) |
Δεc (eV) |
εLUMO: the lowest unoccupied molecular orbital energy. εHOMO: the highest occupied molecular orbital energy. Δε: energy gap, Δε = εLUMO − εHOMO. |
PI1-TPA |
−3.812 |
−4.710 |
0.898 |
PI1-BZA |
−3.844 |
−5.097 |
1.253 |
As shown in Fig. 12, the torsion angle of C–N bond in TPA structure of the model compound for PI1-TPA is about 13.6, 68.7 and 14.5°. The torsion angle of C–N bond in TPA structure limits the movement of the biphenyl group, so that biphenyl group always aligned perpendicular to the plane of alignment film, thereby inducing the vertical alignment of the LC molecules after rubbing. However, biphenyl group of the side chain in PI1-BZA can be rotated around the ester and the bond angle is 119.3°. Thus, biphenyl group of the side chain in PI1-BZA can be easily reoriented along the rubbing direction, which resulted in change of the conformation of biphenyl group of the PI thin films causing the LC molecules parallel alignment.24,26 The alkyl side chains of the PI1-BZA film surface, which are absence of biphenyl rigid support, are prone to lodge and re-orientate along the rubbing direction inducing LC parallel alignment.15 In short, after rubbing, the vertical alignment of PI1-TPA is attributed to the distorted backbone and the fixed torsion angle of C–N bond in TPA structure, which restrains the movement of the biphenyl group of the side chain in the rubbing process. It is suggested that PI1-TPA film is more resistance to rubbing process than PI1-BZA.
4. Conclusions
A novel functional diamine DBDTA containing TPA, biphenyl and long alkyl chain was synthesized and characterized. A series of PIs were prepared based on ODPA, DMMDA and different contents of DBDTA or DBPDA. The PIs with DBDTA exhibited high thermal stability with Tg of 227–240 °C and T5 of 423–493 °C, respectively. The PIs-TPA with DBDTA displayed better solubility and transparency than that with DBPDA.
The PIs showed high pretilt angle and good uniform vertical alignment without rubbing. When the rubbing was performed, PI1-BZA showed parallel alignment and the rest of PIs showed vertical alignment. The different alignment of LC induced by rubbed PI1-TPA and PI1-BZA with the same content of side chains was attributed to the distorted backbone and the fixed torsion angle of C–N bond in TPA, which restrained the movement of the biphenyl group in PI1-TPA film. It provides a method of designing molecular structure for LC vertical alignment film with good solubility, high thermal stability and rubbing resistance.
Acknowledgements
This work was supported by National Natural Science Foundation of China (Grant no. 51173115), the Ministry of Education (the Foundation for Ph.D. training, Grant no. 20110181110030) of China.
References
- X. Y. Chen, V. T. Hoang, D. Rodrigue and S. Kaliaguine, RSC Adv., 2013, 3, 24266 RSC.
- X. Y. Chen, V. T. Hoang, D. Rodrigue and S. Kaliaguine, RSC Adv., 2014, 4, 12235 RSC.
- H. Shima, M. M. Hossain and J. R. Hahn, RSC Adv., 2014, 4, 41204 RSC.
- S. Ramakrishnan, M. Dhakshnamoorthy, E. J. Jelmy, R. Vasanthakumari and N. K. Kothurkar, RSC Adv., 2014, 4, 9743 RSC.
- Y. Niu, X. Zhang, J. Zhao, Y. Tian, Y. Li and X. Yan, RSC Adv., 2014, 4, 28456 RSC.
- H. J. Yen, C. L. Tsai, P. H. Wang, J. J. Lin and G. S. Liou, RSC Adv., 2013, 3, 17048 RSC.
- Y. Q. Fang, J. Wang, Q. Zhang, Y. Zeng and Y. H. Wang, Eur. Polym. J., 2010, 46, 1163 CrossRef CAS PubMed.
- X. Wang, H. Wang, L. Luo, J. Huang, J. Gao and X. Liu, RSC Adv., 2012, 2, 9463 RSC.
- B. Chae, S. B. Kim, S. W. Lee, S. I. Kim, W. Choi, B. Lee, M. Ree, K. H. Lee and J. C. Jung, Macromolecules, 2002, 35, 10119 CrossRef CAS.
- Z. Sun, M. Liu, L. Yi and Y. Wang, RSC Adv., 2013, 3, 7271 RSC.
- P. M. Heregenrother and S. J. Havens, Macromolecules, 1994, 27, 4659 CrossRef.
- C. P. Yang, Y. Y. Su, S. J. Wen and S. H. Hsiao, Polymer, 2006, 47, 7021 CrossRef CAS PubMed.
- Y. T. Chenrn, J. Y. Tsai and J. J. Wang, J. Polym. Sci., Part A: Polym. Chem., 2009, 47, 2443 CrossRef.
- S. Xia, Z. Sun, L. Yi and Y. Wang, RSC Adv., 2013, 3, 14661 RSC.
- Y. J. Lee, J. G. Choi, I. Song, J. M. Oh and M. H. Yi, Polymer, 2006, 47, 1555 CrossRef CAS PubMed.
- Z. J. Liu, F. F. Yu, Q. Zhang, Y. Zeng and Y. Wang, Eur. Polym. J., 2008, 44, 2718 CrossRef CAS PubMed.
- S. H. Ou, V. Percec, J. A. Mann and J. B. Lando, Langmuir, 1994, 10, 905 CrossRef CAS.
- S. H. Hsiao, G. S. Liou, Y. C. Kung, H. Y. Pan and C. H. Kuo, Eur. Polym. J., 2009, 45, 2234 CrossRef CAS PubMed.
- D. H. Wang, Z. Shen, M. Guo, S. Z. D. Cheng and F. W. Harris, Macromolecules, 2000, 40, 889 CrossRef.
- C. F. Chen, W. M. Qin and X. A. Huang, Polym. Eng. Sci., 2008, 10, 1151 Search PubMed.
- L. Wang, P. Chang and C. L. Cheng, J. Appl. Polym. Sci., 2006, 100, 4672 CrossRef CAS.
- J. Y. Hwang, K. J. Lee, D. S. Seo and T. H. Kim, Jpn. J. Appl. Phys., 2003, 42, L6725 Search PubMed.
- X. Wang, P. Zhang, Y. Chen, L. Luo, Y. Pang and X. Liu, Macromolecules, 2011, 44, 9731 CrossRef CAS.
- S. B. Lee, G. J. Shin, J. H. Chi, W. C. Zin, J. C. Jung, S. G. Hahm, M. Ree and T. Chang, Polymer, 2006, 47, 66061 Search PubMed.
- K. Takizawa, J. Wakita, M. Kakiage, H. Masunaga and S. Ando, Macromolecules, 2010, 43, 2115 CrossRef CAS.
- S. W. Lee, S. J. Lee, S. G. Hahm, T. J. Lee, B. Lee, B. Chae, S. B. Kim, J. C. Jung, W. C. Zin, B. H. Sohn and M. Ree, Macromolecules, 2005, 38, 4331 CrossRef CAS.
|
This journal is © The Royal Society of Chemistry 2015 |
Click here to see how this site uses Cookies. View our privacy policy here.