DOI:
10.1039/C4RA16690F
(Paper)
RSC Adv., 2015,
5, 40849-40855
Anisotropic gold nanoparticles for the highly sensitive colorimetric detection of glucose in human urine†
Received
19th December 2014
, Accepted 16th April 2015
First published on 16th April 2015
Abstract
Because of the difference in the surface energies of various crystal facets that arise from a large fraction of edges, corners and vertices, the morphology of nanoparticles has extreme potential for various applications. In this report, using poly(3,4-ethylenedioxythiophene) polystyrene sulfonate (PEDOT:PSS) functionalized anisotropic gold nanoparticles (GNPs) we have demonstrated a simple but robust method for the naked eye colorimetric detection of glucose in human urine with high sensitivity. Glucose oxidase (GOx) was conjugated to the modified anisotropic GNPs. The controlled degree of PEDOT:PSS attached onto the surface of the GNPs was crucial for the detection of glucose. The GNPs were aggregated and the color of the solution changed from pink to blue upon the addition of only 10 μg mL−1 of glucose. The detection limit for glucose was found to be 9.8 μM. Such highly sensitive naked eye detection using PEDOT:PSS will be very useful for low cost homemade sensors.
Introduction
Optical based colorimetric detection has great importance for its high sensitivity for clinically relevant molecules.1–4 This is mainly because of its simplicity, robustness and easy readout. Especially, visible naked eye colorimetric detection is very effective for the fabrication of homemade and low cost sensors for rural peoples. The easy availability of these sensors could overcome the usage of large, sophisticated and non-cost-effective instruments. Consequently, the requirement of a pathology lab for glucose testing will be reduced. Glucose sensing from the blood or urine of a human is of major importance, as improper maintenance of the glucose level in the body may cause serious health problems or even death.5–7 Generally, a normal glucose range in urine is 0–0.8 mmol L−1. Various highly sensitive electrochemical techniques are available, however, there are a few reports on colorimetric and optical based detection in blood as well as in urine.8–29
During the last few decades, nanomaterial based optical sensors have been developed for the sensing of various types of clinical molecules.30–33 Among them, gold nanomaterials were found to be very effective, because of their extensive optical properties, excellent surface recognition and their very high extinction coefficients over those of organic chromophores.34–41 Moreover, the synthetic method is also easy and simple. Although GNPs have shown their potential for various sensing applications, the reports on colorimetric glucose sensors show that they are not up to the mark.17
Further, anisotropic GNPs, because of the differences in the surface energies of various crystal facets arising from a large fraction of edges, corners and vertices, would have unique properties compared with spherical GNPs.42–45 However, the application of anisotropic GNPs in the various relevant fields is very limited.46–50 Very few reports are available on the colorimetric sensing of glucose in urine and blood using anisotropic GNPs.26,51–53
Here, we have demonstrated the use of anisotropic GNPs for optical based colorimetric sensing of glucose in human urine. The synthesized anisotropic GNPs were surface functionalized by PEDOT:PSS, which is the most commonly used polymer for electrochemical sensors.54,55 Controlled surface functionalization played a major role in the highly sensitive detection of glucose, as it helped in the aggregation of the GNPs by providing the optimum zeta potential value. The detection was based on the colorimetric change from pink to blue with an extensive red-shift of the respective surface plasmon resonance (SPR) band. Our sensor showed a detection limit of 9.8 μM estimated at a signal to noise (S/N) ratio of 3 with a regression coefficient of R = 0.99. Such a highly sensitive assay on glucose sensing in human urine using biocompatible PEDOT:PSS surface functionalized anisotropic GNPs will be very beneficial for the design of low cost glucose sensors for third world countries.
Experimental section
Materials
All glasswares were washed with aqua regia (3 HCl
:
1 HNO3), followed by rinsing several times with double distilled water. Gold(III) chloride hydrate (HAuCl4, 99.99%), sodium citrate tribasic hydrate (>99%), sodium borohydride (NaBH4, 99%), L-ascorbic acid, chloroform, PEDOT:PSS (1.3 wt% dispersion in water, Sigma Aldrich 483095), and GOx were purchased from Sigma Aldrich. CTAB (N-cetyl-N,N,N-trimethylammonium bromide), and sodium hydroxide pellets purified (NaOH, 97%), were purchased from Merck. Sodium iodide (NaI, 99%) was purchased from Fisher Scientific, double distilled 18.3 mΩ deionized water (ElgaPurelab Ultra) was used throughout in the preparation of solutions. All of the experiments were performed at room temperature (25 °C).
Synthesis of anisotropic GNPs
GNPs were synthesized using the established seed-mediated method with a minor modification by changing the ascorbic acid concentration.56 5 nm spherical seeds were synthesized by mixing 0.5 mL of a 10 mM aqueous HAuCl4·3H2O solution, 1 mL of a 5 mM aqueous solution of sodium citrate and 1 mL of a 50 mM aqueous NaBH4 (ice-cold) solution in 36.5 mL of deionized water with vigorous stirring until the color of the solution turned red. To prepare GNPs, three labeled flasks were used. A mixture of 108 mL of 0.025 M aqueous CTAB solution and 56 μL of 0.1 M aqueous NaI solution was divided into the three containers labeled 1, 2 and 3. 9 mL of the mixture was added into each of the containers 1 and 2. The remaining mixture, 90 mL, was added into container 3. Finally, a mixture of 125 μL of a 10 mM aqueous HAuCl4·3H2O solution, 50 μL of 50 mM NaOH, and 70 μL of 50 mM ascorbic acid was added to each of the containers 1 and 2. A mixture of 1.25 mL of 10 mM HAuCl4·3H2O, 0.4 mL of 50 mM NaOH, and 0.7 mL of 50 mM ascorbic acid were added into container 3. 1 mL of the seed solution was added to container 1 with mild shaking, followed by adding 1 mL of the container 1 solution into container 2. After gentle shaking, the complete solution of container 2 was added into container 3. The solution was kept overnight for complete growth.
Synthesis of CTAB stabilized spherical GNPs
CTAB stabilized spherical GNPs were synthesized using a seed-mediated growth method.50 The container for seed synthesis held 5 mL of 0.50 mM HAuCl4·3H2O and 5 mL of 0.20 M CTAB. The solution was reduced by addition of 600 μL of ice-cold NaBH4 (0.010 M). Next, the container was shaken vigorously for 2 minutes and occasionally opened to vent any evolved hydrogen gas. The seed solution was a brown suspension and was allowed to age for 2 hours. 12 μL of the seed solution was added to a solution already containing 9.50 mL of 0.10 M CTAB, 80 μL of 0.010 M AgNO3, 500 μL of 0.010 M HAuCl4·3H2O and 55 μL of 0.10 M ascorbic acid. The mixture was stirred for 10 minutes. This resulted in a red suspension that was again left undisturbed for 24 hours to increase the yield. The extra CTAB was removed by centrifugation and resuspension of the nanoparticles into an equal amount of water.
Surface functionalization of the GNPs by PEDOT:PSS
After synthesizing the GNPs, the surface modification was done by using PEDOT:PSS.54–57 Here, a different method was used than the reported PEDOT:PSS modification, that is used for either electrochemical or spin coating applications. In the first step, extra CTAB was removed by carrying out centrifugation at 10
000 rpm for 10 minutes followed by discarding the supernatant and the pellet was redispersed in double distilled water. This process was repeated one more time. To ensure the complete removal of CTAB from the above solution, 5 mL of the GNPs was equilibrated with 5 mL of chloroform. The solution was gently mixed and allowed to stand for 20 minutes. In this way, the CTAB was exchanged from the GNPs to the chloroform layer. Finally, the chloroform layer was discarded. In the second step of the surface functionalization, 100 μL of the PEDOT:PSS solution (1.3 wt% dispersion in water) was added to 10 mL of double distilled water (1% PEDOT:PSS of the original solution). 1 mL of the resulting PEDOT:PSS solution was added to 2 mL of the GNP solution and allowed to stand for 10 minutes.
Conjugation of GOx on PEDOT:PSS functionalized GNPs
For GOx binding to the PEDOT:PSS GNPs, 100 μL of 2 mg mL−1 of GOx solution was added to 900 μL of PEDOT:PSS functionalized GNPs and was mixed gently for 5 minutes. Finally the GOx conjugated solution was stored in the refrigerator for further use. The above GOx concentration was found to be optimum as the GNPs became aggregated even in the absence of glucose, when 100 μL of 3 mg mL−1 GOx was added to the same 900 μL of PEDOT:PSS functionalized GNPs.
Nanoparticle characterization
The size of the GNPs was determined using dynamic light scattering (DLS; Malvern Instruments Ltd., Malvern, UK). All of the measurements were performed at a fixed angle of 173°.57,58 The zeta potential and the overall surface charge were determined using the above instrument. The respective SPR band was measured using Shimadzu UV VIS 2450 and UV VIS NIR 3600 spectrophotometers. Finally, the particle size was confirmed using a high resolution transmission electron microscopy (TEM) using a TECNAI 200 kV TEM (FEI, Electron Optics), and SEM (FEI, Electron Optics) and Field Emission Scanning Electron Microscopy (FE SEM) (using FEI, Electron Optics).
Glucose sensing
The changes in the SPR absorption maxima of the GOx functionalized GNPs on adding predetermined quantities of glucose standard were recorded and calibration curves were plotted with the wavelength against the absorbance. For a titration experiment to observe the SPR change, different amounts of glucose (2.5–50 μg mL−1) were spiked into a fixed volume, 100 μL, of double distilled water in each case. Finally, 100 μL of each of the above glucose samples was added to 900 μL of GOx conjugated PEDOT:PSS GNPs. Each solution was allowed to incubate for 30 minutes before measuring the SPR data. To verify the application of this method in real samples, we performed the same experiment with human urine. The urine sample, which did not contain any glucose, was collected from a healthy person, from the recommended pathology lab, Indian Institute of Technology, Mandi Kamand (HP), India. 100 μL of the urine sample was spiked with 10 μg mL−1 of glucose and then the final solution was added to 900 μL of GOx conjugated PEDOT:PSS GNPs and the absorbance was measured continuously for 30 minutes. The selectivity of the method was also investigated by checking the shift in the SPR absorption maxima of the GOx functionalized GNPs upon interacting with the normal urine sample. To confirm the precision and recovery of the probe, each set of experiments was carried out in triplicate. The results were obtained within an error of 2–3%.
Results and discussion
Fig. 1a depicts the characterized SPR band of the as-prepared anisotropic GNPs, PEDOT:PSS surface functionalized and also GOx conjugated PEDOT:PSS GNPs. A 4 nm red-shift in the SPR band was observed for the PEDOT:PSS surface functionalization compared to the as-prepared anisotropic GNPs. The DLS data (Fig. 1b) showed an overall increase in the size of the GNPs. The increase in the size supported the red-shift in the SPR band. GOx conjugated PEDOT:PSS GNPs, showed a further 5 nm red-shift in the SPR band. This was also confirmed from the DLS data. The TEM and SEM images of the as-synthesized GNPs, PEDOT:PSS GNPs and GOx conjugated PEDOT:PSS GNPs showed that anisotropic GNPs are mainly hexagonal and pentagonal in shape with an average size of 45 nm (the size was determined using 80% of the total population from the TEM analysis) (Fig. 1c, d and ESI Fig. S1†). A few triangular shaped GNPs were also observed. However, the characteristic NIR region SPR band for these GNPs was not observed, which suggested that the majority are hexagonal and pentagonal GNPs. The controlled surface modification of GNPs by PEDOT:PSS and the addition of GOx on the modified GNPs was further confirmed by their characteristic zeta potentials (ESI Fig. S2†). The as-prepared GNPs have a zeta potential of about +68 mV, which arises because of the positively charged CTAB on the GNP surface. The zeta potential of the PEDOT:PSS polymer was observed as −70 mV. Hence, complete surface coverage by replacing all of the CTAB would result in a completely negative zeta potential. This was observed when 2% of PEDOT:PSS (200 μL of 1.3 wt% PEDOT:PSS in 10 mL of water) was used. However, for the maximum efficiency of the detection limit, complete coverage was not helpful. To get the maximum efficiency, 1% of PEDOT:PSS (100 μL of 1.3 wt% PEDOT:PSS in 10 mL of water) was added to the GNP system and the corresponding zeta potential was reduced from +68 mV to +15.6 mV. The addition of GOx further reduced the zeta potential to +6.6 mV. Here, 100 μL of 2 mg mL−1 of GOx was used for optimum binding on PEDOT:PSS modified GNPs. In this case, no aggregation was observed in the anisotropic GNPs. However, a slightly higher concentration of GOx (100 μL of 3 mg mL−1) induced aggregation in the anisotropic GNPs, even in the absence of glucose (ESI Fig. S3†). The TEM and SEM images (Fig. 1c and d) and the observed SPR band of GOx conjugated PEDOT:PSS modified GNPs (ESI Fig. S4†) confirm the stability of the GNPs in the desired experimental conditions.
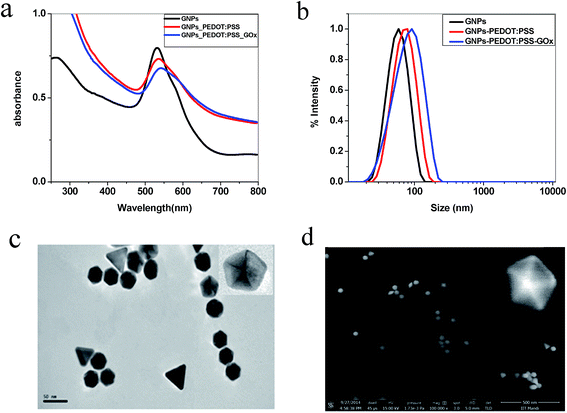 |
| Fig. 1 (a) UV-vis absorption spectra and (b) the hydrodynamic diameter measured using DLS for the as-synthesized anisotropic GNPs, PEDOT:PSS modified GNPs and GOx conjugated PEDOT:PSS surface functionalized GNPs, (c) TEM and (d) SEM images of GOx conjugated PEDOT:PSS surface functionalized anisotropic GNPs; the inset shows the morphology of the anisotropic GNPs. | |
The sensing ability of the anisotropic GNPs is based on the observation of the large SPR band shift due to the changes in the local dielectric constant of the GNPs from the adsorbed biomolecules. The rationale for the colorimetric assay of glucose is essentially based on the aggregation of the anisotropic GNPs induced by glucose through cascade reactions involving glucose, GOx, gluconic acid, H2O2, and H+ (Fig. 2).41 Upon addition of 10 μg mL−1 glucose and incubation for 30 minutes, the SPR band of the PEDOT:PSS–GOx conjugated GNPs showed a 28 nm red-shift. This data confirmed the interaction of glucose with the conjugated GNP system (Fig. 3a). The corresponding hydrodynamic diameter measurement showed that the size of the conjugated GNPs increases to 361 nm, which is due to GNP aggregation. The zeta potential also reduced to +2.6 mV. The aggregation was further confirmed from the TEM and SEM images and the change in color of the GNPs from pink to blue (Fig. 3b–d). To confirm that the degree of surface functionalization was responsible for the maximum detection of glucose, we functionalized the GNPs using different concentrations of the PEDOT:PSS solution. Our data showed that out of 0.5, 1, and 2%, the best aggregation obtained was for the 1% PEDOT:PSS solution (ESI Fig. S5†). Further, to check that the anisotropic nature of the GNPs is mainly responsible for such a highly sensitive detection of glucose, a controlled experiment using PEDOT:PSS functionalized spherical GNPs was also performed. However, no such change was observed in this case (ESI Fig. S6†), which proved the role of the anisotropic nature of the GNPs. Our speculation is based on the fact that the (111) facet of hexagonal and triangular plate GNPs is of low energy and hence the extent of ligand coverage will be more on this facet.59,60 Due to this pre-oriented functionalization, the local concentration of PEDOT:PSS and its conjugation with GOx will be higher. Therefore, the degree of glucose oxidation catalysed by GOx will be more on this facet. On the other hand, because of the isotropic nature the extent of ligand binding is much less in spherical GNPs than in anisotropic GNPs.61,62 As a result, no aggregation was observed in spherical GNPs under similar experimental conditions.
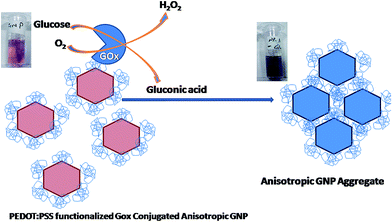 |
| Fig. 2 Schematic of the binding mechanism of GOx with PEDOT:PSS functionalized anisotropic GNPs and the detection of glucose based on the color transition from pink to blue. | |
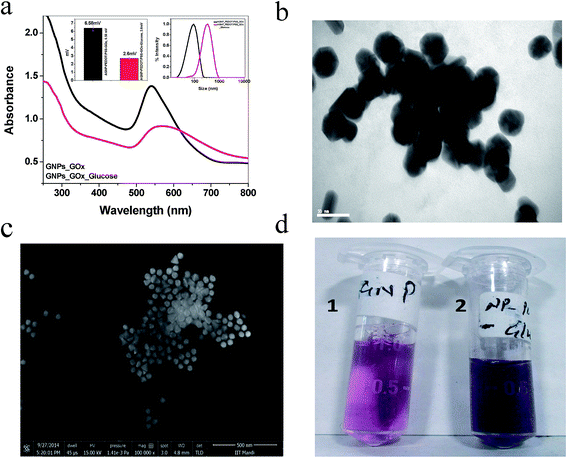 |
| Fig. 3 (a) UV-visible absorption spectra of GOx conjugated PEDOT:PSS functionalized anisotropic GNPs show a red-shift on reacting with 10 μg mL−1 of glucose standard (the corresponding zeta potential is shown in the left hand side inset and the right hand side inset shows the hydrodynamic diameter). (b) TEM image of aggregated anisotropic GNP–PEDOT:PSS–GOx with the addition of 10 μg mL−1 glucose. (c) SEM image of aggregated anisotropic GNP–PEDOT:PSS–GOx with the addition of 10 μg mL−1 glucose. (d) Color changes of (1) anisotropic GNPs and (2) GOx-anisotropic GNPs upon reacting with 10 μg mL−1 glucose after 10 minutes of incubation. | |
To get quantitative analytical information on the sensitivity, the detection limit of the sensor was calculated from a titrimetric experiment, where the concentration of glucose was varied. Fig. 4a shows a gradual change in the SPR band from 542 nm to 565 nm upon the addition of glucose from 2.5–50 μg mL−1. A good linear correlation between the absorbance change and glucose concentration could be established in the range from 2.5 to 20 μg mL−1. No further change in the SPR band was observed upon the addition of more glucose to the solution. The detection limit was found to be 9.8 μM, when the change in absorbance (ΔA) was plotted against the glucose concentration (Fig. 4b).
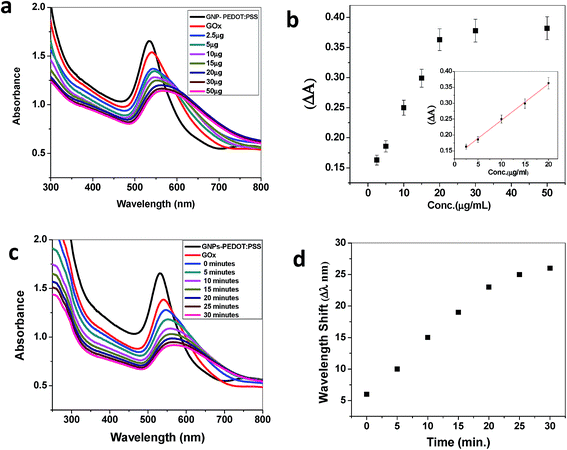 |
| Fig. 4 (a) UV-visible absorption spectra of GOx conjugated anisotropic GNPs show a red-shift upon reacting with different quantities of glucose standard. (b) Plot of the change in absorbance (ΔA) at 542 nm against the glucose concentration for the quantitative detection of glucose. The inset shows the calibration curve of the absorbance change as a function of glucose concentration. (c) Time dependent kinetics of glucose with GOx conjugated anisotropic GNPs. (d) Graphical relationship of the wavelength shift of the SPR band with the progression of the reaction time. | |
To evaluate the optimum time for the catalytic oxidation of glucose by the GOx–GNP conjugate, kinetic studies were also performed. Fig. 4c and d depict the shift in the SPR band with the progression of the reaction time when 10 μg mL−1 of glucose was added and incubated progressively up to 30 minutes. The color change happened within 10 minutes, however, the complete shift in the SPR band did not occur until the saturation end point at around 30 minutes. No further change in the SPR band was observed, rather there was a precipitation of the solution when it was incubated beyond 30 minutes.
The application of the newly developed sensor was evaluated by carrying out the detection of glucose in a real urine sample. A fresh urine sample from a healthy person was collected and spiked with 10 μg mL−1 of glucose followed by addition to the GOx GNP conjugated system. An extensive red-shift in the SPR band was observed (Fig. 5). The color change from pink to blue was also clearly noticeable as was observed for the normal assay (Fig. 3d). It is important to note that our present assay is able to oxidize glucose selectively even in the complex matrix of a urine sample.
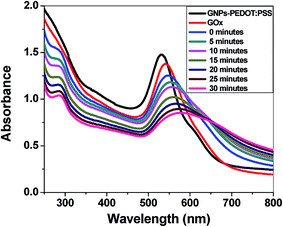 |
| Fig. 5 The SPR band shift of the GOx conjugate GNPs, when a urine sample was spiked with 10 μg mL−1 of glucose. | |
To check that only the added glucose in the urine is responsible for the above sensing, a similar experiment was also performed in the absence of glucose. The pH dependency of our assay was also verified, as the pH of human urine varies from person to person. No noticeable SPR shift was observed in either case (ESI Fig. S7 and S8†). Finally, we also tested the effect of cysteine, which is majorly present in urine samples. No effect was also observed in this case (ESI Fig. S9†).17 All of the above experiments confirmed that the developed GOx GNP system has high selectivity for glucose even in the presence of interference from other agents.
The glucose-induced GNP aggregation was considered to be a consequence of the suppressive effect of the glucose against the coulombic repulsion between the PEDOT:PSS stabilized GNPs.63 This was proposed from the decrease in zeta potential when the glucose was added to the system. If all of the GNPs in the suspension have either large negative or positive zeta potentials, the high electrostatic repulsion is protected from any aggregation.64 However, the low zeta potential for the controlled PEDOT:PSS functionalization helped the GNPs to aggregate. The zeta potential of the conjugated GOx PEDOT:PSS GNPs was found to be 6.6 mV. On addition of 10 μg mL−1 glucose it was further reduced to +2.6 mV. The significant decrement in the zeta potential upon the addition of glucose could be explained by the GOx catalyzed oxidation of glucose to gluconic acid via gluconate.41 Gluconic acid could reduce the cationic character of the anisotropic GNPs, which can help in bringing down the zeta potential of the assay. This observation is also supported by dynamic light scattering (DLS) measurements. The size of the GOx conjugate system is increased from 56 nm to 361 nm upon the addition of glucose. This data clearly suggested that the aggregation of the anisotropic GNPs is taking place due to the oxidative degradation of the glucose to gluconic acid. For further verification of the mechanism for the produced gluconic acid that is responsible for the aggregation by reducing the zeta potential, the fresh urine sample spiked only with 51 μM of gluconic acid added to the PEDOT:PSS GOx conjugated GNPs. After 10 minutes of equilibration, a 14 nm shift in the SPR band of the assay was observed confirming the role of gluconic acid (ESI Fig. S10†).
Conclusion
PEDOT:PSS surface functionalized anisotropic GNPs have been designed for a colorimetric naked eye glucose sensor. The corners and edges of anisotropic GNPs were found to play a major role in the glucose sensing. Further, an optimum surface coverage of PEDOT:PSS was also very useful for the maximum detection limit. The results will be very effective for the fabrication of homemade, low cost sensors for rural people, especially in third world countries.
Acknowledgements
The authors acknowledge the home institute (IIT Mandi) and Department of Science and Technology (DST) India for their financial support (Project no. IITM/SG/CKN/003 and SR/FT/CS-152/2011) for running the experiments. AC thanks Charu Dwivedi for helpful discussion.
References
- J. Homola, S. S. Yee and G. Gauglitz, Sens. Actuators, B, 1999, 54, 3 CrossRef CAS.
- A. J. Haes, L. Chang, W. Klein and V. R. P. Duyne, J. Am. Chem. Soc., 2005, 127, 2264 CrossRef CAS PubMed.
- R. L. Rich and D. G. Myszka, J. Mol. Recognit., 2005, 18, 431 CrossRef CAS PubMed.
- D. R. Shankaran, K. V. Gobi and N. Miura, Sens. Actuators, B, 2007, 121, 158 CrossRef CAS PubMed.
- A. J. Haes and R. P. Duyne, J. Am. Chem. Soc., 2002, 124, 10596 CrossRef CAS PubMed.
- P. K. Jain and M. A. El-Sayed, J. Phys. Chem. C, 2007, 111, 17451 CAS.
- M. D. Malinsk, K. L. Kelly, G. C. Schatz and V. R. P. Duyne, J. Am. Chem. Soc., 2001, 123, 1471 CrossRef.
- X. Kang, Z. Mai, X. Zou, P. Cai and J. Mo, Anal. Biochem., 2007, 369, 71 CrossRef CAS PubMed.
- S. H. Lim, J. Wei, J. Lin, Q. Li and J. K. You, Biosens. Bioelectron., 2005, 20, 2341 CrossRef CAS PubMed.
- J. Liu, M. Agarwal and K. Varahramyan, Sens. Actuators, B, 2008, 135, 195 CrossRef CAS PubMed.
- D. J. Macaya, M. Nikolou, S. Takamatsu, J. T. Mabeck, R. M. Owens and G. G. Malliaras, Sens. Actuators, B, 2007, 123, 374 CrossRef CAS PubMed.
- J. Park, H. K. Kim and Y. Son, Sens. Actuators, B, 2008, 133, 244 CrossRef CAS PubMed.
- P. Santhosh, K. M. Manesh, S. Uthayakumar, S. Komathi, A. I. Gopalan and K. P. Lee, Bioelectrochemistry, 2009, 75, 61 CrossRef CAS PubMed.
- X. D. Hoa, A. G. Kirkb and M. Tabrizian, Biosens. Bioelectron., 2007, 23, 151 CrossRef CAS PubMed.
- S. Nambiar and J. T. W. Yeow, Biosens. Bioelectron., 2011, 26, 1825 CrossRef CAS PubMed.
- U. Lange, N. V. Roznyatovskaya and V. M. Mirsky, Anal. Chim. Acta, 2008, 614, 1 CrossRef CAS PubMed.
- C. Radhakumary and K. Sreenivasan, Anal. Chem., 2011, 83, 2829 CrossRef CAS PubMed.
- Y. Jiang, H. Zhao, Y. Lin, N. Zhu, Y. Ma and L. Mao, Angew. Chem., 2010, 49, 4800 CrossRef CAS PubMed.
- G. Palazzo, L. Facchini and A. Mallardi, Sens. Actuators, B, 2012, 161, 366 CrossRef CAS PubMed.
- R. Narayanan, R. J. Lipert and M. D. Porter, Anal. Chem., 2008, 80, 2265 CrossRef CAS PubMed.
- T. Lin, L. Zhong, Z. Song, L. Guo, H. Wu, Q. Guo, Y. Chen, F. Fu and G. Che, Biosens. Bioelectron., 2014, 62, 302 CrossRef CAS PubMed.
- Q. Liu, H. Li, Q. R. Zhao, Y. Zhu, Q. Jia, B. Bian and L. Zhuo, Mater. Sci. Eng., C, 2014, 41, 142 CrossRef CAS PubMed.
- L. Su, W. Qin, H. Zhang, Z. U. Rahman, C. Ren, S. Ma and X. Chen, Biosens. Bioelectron., 2015, 63, 384 CrossRef CAS PubMed.
- L. Su, J. Feng, X. Zhou, C. Ren, H. Li and X. Chen, Anal. Chem., 2012, 84, 5753 CrossRef CAS PubMed.
- M. Miyashita, N. Ito, S. Ikeda, T. Murayama, K. Oguma and J. Kimura, Biosens. Bioelectron., 2009, 24, 1336 CrossRef CAS PubMed.
- H. Gao, F. Xiao, C. B. Ching and H. Duan, ACS Appl. Mater. Interfaces, 2011, 3, 3049 CAS.
- M. Veerapandian, Y. T. Seo, H. Shin, K. Yun and M. H. Lee, Int. J. Nanomed., 2012, 7, 6123 CrossRef CAS PubMed.
- K. V. Kong, Z. Lam, W. K. O. Lau, W. K. Leong and M. Olivo, J. Am. Chem. Soc., 2013, 135, 18028 CrossRef CAS PubMed.
- A. T. E. Vilian, S. M. Chen, M. A. Alib and F. M. A. Al-Hemaidb, RSC Adv., 2014, 4, 30358 RSC.
- D. Liu, J. Yang, H. F. Wang, Z. Wang, X. Huang, Z. Wang, G. Niu, A. R. H. Walker and X. Chen, Anal. Chem., 2014, 86, 5800 CrossRef CAS PubMed.
- R. A. Reynolds, C. A. Mirkin and R. L. Letsinger, J. Am. Chem. Soc., 2000, 122, 3795 CrossRef CAS.
- M. Rex, F. E. Hernandez and A. D. Campiglia, Anal. Chem., 2006, 78, 445 CrossRef CAS PubMed.
- C. C. Huang and H. T. Chang, Anal. Chem., 2006, 78, 8332 CrossRef CAS PubMed.
- P. K. Jain, X. Huang, I. H. E. Sayed and M. E. Sayed, Acc. Chem. Res., 2008, 41, 1578 CrossRef CAS PubMed.
- E. Ozbay, Science, 2006, 311, 189 CrossRef CAS PubMed.
- P. K. Jain, W. Huang and M. A. E. Sayed, Nano Lett., 2007, 7, 2080 CrossRef CAS.
- S. Underwood and P. Mulvaney, Langmuir, 1994, 10, 3427 CrossRef CAS.
- E. Hao, G. C. Schatz and J. T. Hupp, J. Fluoresc., 2004, 14, 331 CrossRef CAS.
- M. E. Sayed, Acc. Chem. Res., 2001, 34, 257 CrossRef PubMed.
- C. J. Gannon, C. R. Patra, R. Bhattacharya, P. Mukherjee and S. A. Curley, J. Nanobiotechnol., 2008, 6, 1 CrossRef PubMed.
- F. Wang, X. Liu, C. H. Lu and I. Willner, ACS Nano, 2013, 7, 7278 CrossRef CAS PubMed.
- E. C. Cho, L. Au, Q. Zhang and Y. Xia, Small, 2010, 6, 517 CrossRef CAS PubMed.
- W. Jiang, B. Y. Kim, J. T. Rutka and W. C. Chan, Nat. Nanotechnol., 2008, 3, 145 CrossRef CAS PubMed.
- P. Nativo, I. A. Prior and M. Brust, ACS Nano, 2008, 2, 1639 CrossRef CAS PubMed.
- J. E. Gagner, M. D. Lopez, J. S. Dordick and R. W. Siegel, Biomaterials, 2011, 32, 7241 CrossRef CAS PubMed.
- G. Yang, F. Zhao and B. Zeng, Talanta, 2014, 127, 116 CrossRef CAS PubMed.
- X. Huang, I. H. E. Sayed, W. Qian and M. A. E. Sayed, J. Am. Chem. Soc., 2006, 128, 2115 CrossRef CAS PubMed.
- A. Wijaya, S. B. Schaffer, I. G. Pallares and K. H. Schifferli, ACS Nano, 2009, 3, 80 CrossRef CAS PubMed.
- C. Bao, N. Beziere, P. D. Pino, B. Pelaz, G. Estrada, F. Tian, V. Ntziachristos, V. Fuente and D. Cui, Small, 2013, 9, 68 CrossRef CAS PubMed.
- A. Chaudhary, A. Gupta, S. Khan and C. K. Nandi, Phys. Chem. Chem. Phys., 2014, 16, 20471 RSC.
- Y. Xianyu, J. Sun, Y. Li, Y. Tian, Z. Wang and X. Jiang, Nanoscale, 2013, 5, 6303 RSC.
- Y. Xia, J. Ye, K. Tan, J. Wang and G. Yang, Anal. Chem., 2013, 85, 6241 CrossRef CAS PubMed.
- A. C. Fdez, T. Lopez-Luke, A. T. Castro, D. A. Wheeler, J. Z. Zhangd and E. D. Rosa, RSC Adv., 2014, 4, 59233 Search PubMed.
- K. M. Manesha, P. Santhosh, A. Gopalan and K. P. Lee, Talanta, 2008, 75, 1307 CrossRef PubMed.
- G. Istamboulie, T. Sikora, E. Jubete, E. Ochoteco, J. L. Marty and T. Noguer, Talanta, 2010, 82, 957 CrossRef CAS PubMed.
- S. Hong, Y. Choi and S. Park, Chem. Mater., 2011, 23, 5375 CrossRef CAS.
- S. Khan, A. Gupta and C. K. Nandi, J. Phys. Chem. Lett., 2013, 4, 3747 CrossRef CAS.
- S. Khan, A. Gupta, A. Chaudhary and C. K. Nandi, J. Chem. Phys., 2014, 141, 084707 CrossRef PubMed.
- C. H. Kuo, T. F. Chiang, L. J. Chen and M. H. Huang, Langmuir, 2004, 20, 7820 CrossRef CAS PubMed.
- J. E. Millstone, S. Park, K. L. Shuford, L. Qin, G. C. Schatz and C. A. Mirkin, J. Am. Chem. Soc., 2005, 127, 5312 CrossRef CAS PubMed.
- C. Dwivedi, A. Chaudhary, A. Gupta and C. K. Nandi, ACS Appl. Mater. Interfaces, 2015, 7, 5039–5044 CAS.
- M. R. Jones, R. J. Macfarlane, A. E. Prigodich, P. C. Patel and C. A. Mirkin, J. Am. Chem. Soc., 2011, 133, 18865–18869 CrossRef CAS PubMed.
- N. Wangoo, J. Kaushal, K. K. Bhasin, S. K. Mehta and C. R. Suri, Chem. Commun., 2010, 46, 5755 RSC.
- R. P. Bagwe, L. R. Hilliard and W. Tan, Langmuir, 2006, 22, 4357 CrossRef CAS PubMed.
Footnote |
† Electronic supplementary information (ESI) available. See DOI: 10.1039/c4ra16690f |
|
This journal is © The Royal Society of Chemistry 2015 |
Click here to see how this site uses Cookies. View our privacy policy here.