DOI:
10.1039/C4RA16387G
(Paper)
RSC Adv., 2015,
5, 22289-22293
Cathodic electrochemiluminescence behavior of an ammonolysis product of 3,4,9,10-perylenetetracarboxylic dianhydride in aqueous solution and its application for detecting dopamine†
Received
15th December 2014
, Accepted 12th February 2015
First published on 12th February 2015
Abstract
The cathodic electrochemiluminescence (ECL) behavior of the ammonolysis product of 3,4,9,10-perylenetetracarboxylic dianhydride (PTC–NH2) in aqueous solution with K2S2O8 as the co-reactant has been, for the first time reported, in this paper. The possible mechanisms have been investigated. Based on the fact that dopamine (DA) can efficiently quench the ECL intensity of PTC–NH2, DA was chosen as a template to investigate the application of PTC–NH2–S2O82− system in the sensor field. Under the optimal conditions, we achieved the detection of DA with the detection limit of 1.6 × 10−9 M. Due to the excellent ECL behavior, PTC–NH2 could be a new class of promising material for the construction of ECL sensors.
1. Introduction
During the past several years, perylene has attracted considerable attention due to its outstanding optical properties.1 As early as 1970, Werner et al. investigated the ECL of perylene using an image intensifier spectrograph in benzonitrile and acetonitrile as the solvent.2 Based on this work of Werner et al., Lee and co-workers in 1990 further investigated the ECL and electrochemical properties of a homologous series of perylene diimides in an aprotic solution.3 However, the ECL behaviors of the abovementioned components in an aqueous solution have not been attainable owing to the poor solubility and radical ion stability problems.4 Furthermore, the application of poisonous organic solvent not only limits the applications of perylene, but it is also harmful to the human body. Thus, it is still important and urgent to find a gentle and nontoxic aqueous solution system, in which the analytical interest is the highest, to further study the ECL behaviors of perylene, which would expanding the application of these ECL luminophores. One of the effective methods for solving this problem is to introduce multi-polar groups, such as carboxyl and amido in perylene to synthesize water-soluble derivatives. Our group found that 3,4,9,10-perylenetetracarboxylic acid (denoted as PTCA) has good water-solubility and could enhance the ECL intensity of the O2–S2O82− system and Ru(II) system.5 Based on the abovementioned facts, we constructed PTCA based biosensors, which exhibited high sensitivity owing to the outstanding features of PTCA.6 However, to date, the ECL behavior of PTCA cannot be achieved in neutral aqueous solution. In addition, in our previous study, another derivative of perylene, the ammonolysis product of 3,4,9,10-perylenetetracarboxylic dianhydride (denoted as PTC–NH2), has also been used to enhance the ECL intensity of the Ru(II) system.7 Fortunately, in this work, we found the ECL of PTC–NH2 in neutral aqueous solution, and we investigated the cathodic ECL behavior of PTC–NH2 in aqueous solutions with potassium peroxydisulfate (K2S2O8) as the co-reactant and discussed the application of PTC–NH2 in ECL sensing.
In previous ECL systems, graphite-like carbon nitride,8 Au clusters,9 Ru complex,10 and some semiconductor quantum dots (i.e., CdS,11 CdTe,12 AgSe13), carbon nanodot14 and 5,5′-bis(methylthio)-2,2′-bithiophene (BMTbT)15 have been widely used as ECL luminophores to fabricate sensors for the detection of DA, phenolic compounds, Cu2+, DNA, Hg2+, and ascorbic acid. Compared to the abovementioned luminophores, the PTC–NH2 not only overcomes the toxicity, poor water-solubility or high-cost problem, but also exhibits some attractive advantages such as the desirable optical properties, outstanding film-forming ability and organic electronic properties.1 Thus, it is of great significance to investigate the cathodic ECL behavior of PTC–NH2 in water to expand its applications in the field of analytical chemistry.
In this work, the cathodic ECL of PTC–NH2 was tested in 0.10 M phosphate-buffered saline (PBS) containing K2S2O8 as the co-reactant using cyclic voltammetry and ECL measurements. The ECL mechanism of PTC–NH2 is shown in Scheme 1. The major contribution of this work is to study the cathodic ECL mechanism of PTC–NH2 with K2S2O8 as a co-reactant in gentle, neutral aqueous solution, which would open a new avenue to fabricate promising ECL sensing platforms. Based on the ECL behavior of luminophore PTC–NH2, DA was chosen as a template to investigate the application of PTC–NH2–S2O82− system in the sensor field. It is found that DA could quench the ECL signal of PTC–NH2; thus, the determination of DA at the PTC–NH2 modified electrode can be achieved with high sensitivity and selectivity. This work revealed the fact that the water-soluble luminophore PTC–NH2 would open a new avenue to further develop ECL sensors.
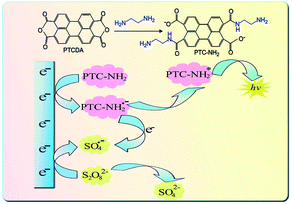 |
| Scheme 1 Diagram of the preparation process and ECL mechanism of PTC–NH2. | |
2. Experimental
2.1 Reagents and chemical
3,4,9,10-Perylenetetracarboxylic dianhydride (PTCDA) was obtained from Lian Gang Dyestuff Chemical Industry Co. Ltd (Liaoning, China). Dopamine (DA) was purchased from Sigma Chemical Co. (St. Louis, MO, USA). Uric acid and ascorbic acid were purchased from Aladdin Ltd. (Shanghai, China). Potassium persulfate (K2S2O8) was purchased from Chengdu Kelong Chemical Reagent Co. (Chengdu, China). Phosphate-buffered saline (PBS) solutions with various pH were prepared using 0.10 M KH2PO4 and 0.10 M Na2HPO4. 0.10 M KCl was used as supporting electrolyte. All the other materials used were of analytical grade. Double distilled water was used throughout the work.
2.2 Apparatus
Electrochemical measurements were performed using a CHI660D electrochemical workstation (CH Instruments Co., China). ECL measurements were carried out on a MPI-A electrochemiluminescence analyzer (Xi'an Remax Electronic Science & Technology Co. Ltd., Xi'an, China). The prepared GCE was used as the working electrode, a platinum wire was used as the auxiliary electrode and an Ag/AgCl (sat.) electrode was used as the reference electrode. For the detection, the voltage of the photomultiplier tube (PMT) was set at 800 V and potential scan from 0 to −0.4 V was used. The FT-IR spectra were recorded on a Nexus 670 FT-IR spectrophotometer (Nicolet Instruments) and scanning electron micrographs were recorded on a scanning electron microscope (SEM, Hitachi, Japan).
2.3 Synthesis of PTC–NH2
First, 0.50 g PTCDA was dissolved in 15 mL anhydrous ethanol with vigorous stirring, and then 0.5 mL ethylenediamine was slowly dripped into the abovementioned PTCDA solution at 4 °C to synthesise PTC–NH2. Subsequently, the prepared PTC–NH2 was centrifuged and washed with ethanol and acetone to remove the residual ethylenediamine. Finally, the PTC–NH2 was dispersed in water for further use. The preparation process of PTC–NH2 is shown in Scheme 1. The toxicity of PTC–NH2 was tested on cells using the MTT assay and the result is presented in ESI.†
2.4 Preparation of the sensor
First, a glassy carbon electrode (GCE, Φ = 4.0 mm) was polished with 0.3 μm and 0.05 μm alumina slurry, and then ultrasonically cleaned in water and ethanol. Finally, 10 μL suspension of PTC–NH2 was dripped onto the surface of as-prepared GCE to obtain the PTC–NH2/GCE sensor.
2.5 Experimental measurements
The ECL performance of PTC–NH2/GCE for detecting DA was investigated in 3.0 mL 0.10 M PBS containing 90 mM K2S2O8. In this study, we found that dopamine could quench the ECL signal of PTC–NH2/GCE. The measurement was based on the change of the ECL signal (ΔI = I0 − It); here, It and I0 are the ECL intensity with and without DA, respectively.
3. Results and discussion
3.1 Characterization of the PTC–NH2
The surface topographies of PTC–NH2 hybrid were investigated by scanning electron microscope (SEM). As presented in Fig. 1A, PTC–NH2 exhibited irregularly quadrate shaped molecular islands, which is consistent with a previous report.1 Fig. 1B displays the FT-IR spectra of PTCDA (curve a) and PTC–NH2 (curve b). As shown in curve a, the absorption peak at 1599 cm−1 for condensed aromatic ring of the perylene skeleton and the peaks at 1773 cm−1, 1302 cm−1 and 1023 cm−1 for the side groups in PTCDA are clearly observed. As expected, the characteristic peak of PTCDA at 1599 cm−1 was also observed in curve b; moreover, the bending vibration peak of N–H at 1651 cm−1 and the stretching vibration of C–N at 1251 cm−1 were clearly observed in curve b. This fact indicated that the PTC–NH2 was successfully prepared.
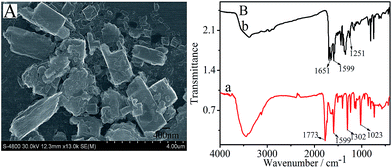 |
| Fig. 1 (A) SEM image of PTC–NH2; (B) FT-IR spectra of PTCDA (a) and PTC–NH2 (b). | |
3.2 ECL and electrochemical behavior of the PTC–NH2/GCE
The ECL behavior of PTC–NH2/GCE was detected in 0.10 M PBS without and with 90 mM K2S2O8, respectively. As seen in Fig. 2A, curve a depicted the ECL behavior of PTC–NH2/GCE in the absence of co-reactant K2S2O8. A very weak ECL signal was observed at −0.35 V, as shown in the amplified ECL profile of curve a (the inset of Fig. 2A). Moreover, a strong ECL signal was observed for PTC–NH2/GCE in the presence of 90 mM K2S2O8 (curve b of Fig. 2A). However, it was reported that K2S2O8 can act with the dissolved O2 to generate an ECL emission.18 Thus, to investigate whether the O2–S2O82− system can generate ECL emission under our working potential (−0.4–0.0 V) to interfere with the ECL response of the PTC–NH2–S2O82− system, we compared the ECL response of a bare electrode and PTC–NH2/GCE with 90 mM K2S2O8 in the potential range of −2.0–0.0 V. The results are shown in Fig. S1.† As seen from curve a, the O2–S2O82− system exhibited an ECL emission at bare GCE in the potential range of −2.0–1.0 V (the inset of Fig. S1†). Moreover, in curve b, the ECL emission in the potential range of −2.0–1.0 V and −0.8–0.0 V can be observed, which correspond to the ECL response of the O2–S2O82− system and PTC–NH2–S2O82− system, respectively. Obviously, the ECL emission resulting from the O2–S2O82− system do not interfere with our detection at the working potential (−0.4–0.0 V).
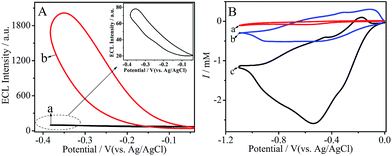 |
| Fig. 2 (A) ECL behaviors of PTC–NH2/GCE without (a) and with 90 mM K2S2O8 (b); inset: the enlarged image of curve a; (B) CV curves of bare GCE (a), PTC–NH2/GCE without (b) and with 90 mM K2S2O8 (c). | |
Abovementioned fact confirmed that the PTC–NH2 was a luminophore, and K2S2O8 as a co-reactant could significantly enhance the ECL signal of PTC–NH2, which is similar to the ECL pathways of other luminophore.11–13 The possible ECL mechanisms of PTC–NH2 with K2S2O8 as co-reactant are as follows:
|
PTC–NH2 + e → PTC–NH2˙−
| (1) |
|
S2O8˙2− → SO42− + SO4˙−
| (3) |
|
PTC–NH2˙− + SO4˙− → SO42− + PTC–NH2*
| (4) |
|
PTC–NH2 + SO4˙− → SO42− + PTC–NH2+
| (5) |
|
PTC–NH2˙− + PTC–NH2+ → PTC–NH2* + PTC–NH2
| (6) |
|
PTC–NH2* → PTC–NH2 + hv
| (7) |
It has been reported that the radicals generated from the co-reactants could react with electro-reduced semiconductors to produce ECL.16,17 In this work, semiconductor PTC–NH2 can be electro-reduced by injecting an electron from the working electrode into the conduction band of PTC–NH2 to form the negatively charged PTC–NH2˙− when the applied potential is more negative than the valence band of PTC–NH2 in the negative potential scan (eqn (1)). To confirm this conclusion, we investigated the electrochemical behavior of bare GCE and PTC–NH2/GCE in 0.10 M PBS without K2S2O8 using cyclic voltammetry (CV). The results are shown in Fig. 2B. As it can be seen, compared to the bare GCE without K2S2O8 (curve a), the PTC–NH2/GCE exhibited an obvious increase in the reduction current in the absence of K2S2O8 (curve b), which is owing to the electro-reduction of PTC–NH2. This fact confirmed the process of electron injection, which is shown in eqn (1).
Furthermore, the electrochemical behavior of PTC–NH2/GCE was also investigated in the presence of K2S2O8. As can be seen in Fig. 2B curve c, a broad peak at −0.52 V is observed. In contrast, no broad peak is found for the PTC–NH2/GCE in the absence of K2S2O8 (curve b), indicating that the broad peak observed at PTC–NH2/GCE (curve c) is due to the electro-reduction of the co-reactant S2O82− (eqn (2) and (3)).18,19 A similar CV scan was conducted at bare GCE in the presence of S2O82−and the result is exhibited in Fig. S2.† As it can be seen, a broad reduction peak of S2O82− was observed at −0.92 V, which is more negative than that at PTC–NH2/GCE (−0.52 V), indicating the fact that the S2O82− could be more easily electro-reduced on the PTC–NH2 modified electrode. During the cathodic scan, SO4˙−, a strong oxidant species, can be generated due to electro-reduction (eqn (2) and (3)). Subsequently, PTC–NH2* was generated when PTC–NH2˙− reacted with SO4˙−, and the electron transferred from PTC–NH2˙− to SO4˙− (eqn (4)). Another possible mechanism to generate PTC–NH2* is shown in eqn (5) and (6). The strong oxidant species, SO4˙−, could oxidize PTC–NH2 to PTC–NH2+ (eqn (5)). Subsequently, the PTC–NH2+ and PTC–NH2˙− could react with each other to generate the excited state PTCNH2* (eqn (6)). Finally, the excited state could fall to ground state, and light could be emitted (eqn (7)).
Fig. 3A displays the photoluminescence (PL) spectrum of the PTC–NH2 at 708 nm with an excitation wavelength of 450 nm. The ECL spectrum of PTC–NH2 was also investigated in the potential range of −0.4–0.0 V with 90 mM K2S2O8 as the co-reactant. The result is shown in Fig. 3B. A distinguished ECL spectrum peak at 700 nm was clearly observed. As expected, no obvious spectral shift between the PL and ECL was observed, indicating that the ECL peak energy basically matches with the energy of the optical band gap. The abovementioned facts are consistent with those of other semiconductors,20 indicating that the ECL emission is a band gap luminescence.21
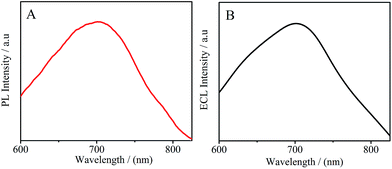 |
| Fig. 3 (A) The PL spectrum of PTC–NH2 solution at an excitation wavelength of 450 nm, (B) the ECL spectrum of the PTC–NH2/GCE in 0.10 M PBS containing 90 mM K2S2O8 obtained by cycling the potential range from −0.4 to 0.0 V (vs. Ag/AgCl). | |
3.3 Optimization of experimental conditions
The ECL signal from the PTC–NH2/GCE is affected by the pH of PBS and the concentration of K2S2O8. In this study, the abovementioned working conditions were optimized. The change of ECL intensity (ΔI) was tested at PTC–NH2/GCE in 0.10 M PBS containing 90 mM K2S2O8 and 500 nM DA by varying pH from 5.5 to 8.5. As can be seen in Fig. 4A, ΔI increased with increase in pH from 5.5 to 7.0, whereas decreased with increase in pH from 7.0 to 8.5. Thus, pH 7.0 was chosen as the optimal pH because the strongest ECL emission was produced at this value.
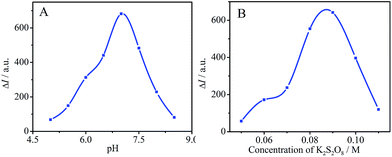 |
| Fig. 4 Effects of (A) the pH of tested solution and (B) the concentration of K2S2O8 on the ECL response at the PTC–NH2/GCE. | |
The effect of the concentration of K2S2O8 was tested at PTC–NH2/GCE in 0.10 M PBS (pH 7.0) containing different concentrations of K2S2O8 and 500 nM DA. The results are shown in Fig. 4B. It was found that the ΔI increased with the increasing concentration of K2S2O8 from 50 mM to 90 mM, and achieved the maximum value at 90 mM K2S2O8. Thus, the optimal concentration of 90 mM K2S2O8 was chosen for the ECL determination of DA in the subsequent investigation.
3.4 ECL detection of dopamine
In this study, it is found that DA could effectively quench the ECL signal at PTC–NH2/GCE in the potential range of −0.4–0.0 V with 90 mM K2S2O8 as co-reactant. Fig. 5 exhibits the ECL emission at PTC–NH2/GCE towards various concentrations of DA. Obviously, the ECL signal decreased linearly with the concentration of DA from 5.0 × 10−9 M to 1.1 × 10−6 M, and the linear regression equation is ΔI = 1.30C + 192 (R = 0.997) and the detection limit is 1.6 × 10−9 M (signal to noise = 3). The results suggest that the prepared PTC–NH2/GCE exhibited a good performance for the detection of DA. The quenching mechanism may be explained according to the Liu et al.'s work.12 They used CdTe quantum dots to construct a sensor for the detection of DA by quenching. In their work, the ECL quenching mechanism has been conformed to be an energy-transfer process. Moreover, the energy transfer resulted from the collision between the produced o-benzoquinone species and the excited state of QDs. In our work, the ECL quenching mechanism for detecting DA may be similar to the Liu's work, because it also involved an energy-transfer process, which results from the collision between the produced o-benzoquinone species and the excited state of PTC–NH2.
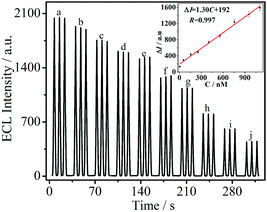 |
| Fig. 5 ECL responses of PTC–NH2/GCE towards different concentrations of DA: 0.00 (a), 5.00 (b), 55.0 (c), 155 (d), 255 (e), 405 (f), 555 (g), 755 (h), 955 (i), and 1105 (j) nM. Inset: calibration curve for DA. Here, ΔI = I0 − It; It and I0 are the ECL intensity with and without DA, respectively. | |
3.5 Stability and interference determination of the sensor
The stability is one of the major concerns for a sensor. Fig. 6 shows the ECL responses of PTC–NH2/GCE towards 600 nM DA under the optimum conditions. The relative standard deviation (R. S. D.) was 1.4% for 10 successive detections.
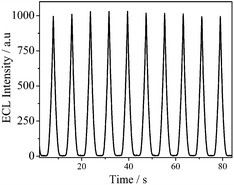 |
| Fig. 6 The ECL stability of PTC–NH2/GCE to 600 nM DA. | |
The long term stability of PTC–NH2/GCE was also investigated in 0.10 M PBS with 90 mM K2S2O8 and 600 nM DA. The sensor was used to detect DA once a day for ten days. It was found that the ECL signal at the sensor only decrease by 13.2% after ten days.
The selectivity of the PTC–NH2/GCE was also investigated using L-serine (L-Ser), L-arginine (L-Arg), L-cysteine (L-Cys), Mg2+, ascorbic acid, uric acid, glucose and SO42− as interfering substances. The PTC–NH2/GCE was exposed to these interfering substances (0.10 M) under the optimum conditions. As shown in Fig. 7, the change of the ECL signal (ΔI = I0 − It) caused by the interfering substances can almost be ignored when compared to the case when DA is present, indicating the excellent selectivity of PTC–NH2/GCE for DA.
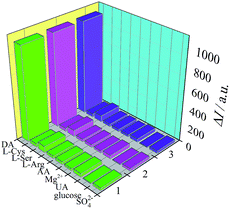 |
| Fig. 7 The selectivity of the PTC–NH2/GCE:DA (500 nM), L-Cys (0.10 M), L-Ser (0.10 M), L-Arg (0.10 M), AA (0.10 M), Mg2+ (0.10 M), UA (0.10 M), glucose (0.10 M) and SO42− (0.10 M). | |
3.6 Analytical application of the sensor in real samples
To further evaluate the real application of the PTC–NH2/GCE, the sensor was applied to test DA in a dopamine hydrochloride injection sample. The results are exhibited in Table S1.† The recoveries ranged between 92.9% and 105% in the dopamine hydrochloride injection sample, indicating that the PTC–NH2/GCE has promising applications for the detection of DA in real samples.
Conclusions
This paper investigated the cathodic ECL behavior of PTC–NH2 with K2S2O8 as the co-reactant for the first time and discussed the application of PTC–NH2 in ECL sensing. Based on the fact that DA could quench the ECL signals of the PTC–NH2 modified electrode, the detection of DA was achieved, confirming that PTC–NH2 would be a new, promising and efficient water-soluble luminophore for application in ECL sensing. Moreover, this study offers an effective and simple technique for DA analysis.
Acknowledgements
This work was supported by the National Natural Science Foundation of China (21075100, 21275119), Ministry of Education of China (Project 708073), Research Fund for the Doctoral Program of Higher Education (RFDP) (20110182120010), the Natural Science Foundation of Chongqing City (CSTC-2011BA7003, CSTC-2014JCYJA20005, CSTC-2010BB4121), Science and Technology Commission of Beibei (2012–27), Medical Scientific Research Projects of Health Bureau of Chongqing (2012-2-286), and the Fundamental Research Funds for the Central Universities (XDJK2012A004, XDJK2013C115). Specialized research fund for the doctoral program of higher education (swu113029) in China is also acknowledged.
Notes and references
- Y. Zhuo, P. X. Yuan, R. Yuan, Y. Q. Chai and C. L. Hong, Biomaterials, 2008, 29, 1501–1508 CrossRef CAS PubMed.
- T. C. Werner, J. Chang and D. M. Hercules, J. Am. Chem. Soc., 1970, 92, 5560–5565 CrossRef CAS.
- S. K. Lee, Y. Zu, A. Herrmann, Y. Geerts, K. Mullen and A. J. Bard, J. Am. Chem. Soc., 1999, 121, 3513–3520 CrossRef CAS.
- J. E. Dick, C. Renault, B. K. Kim and A. J. Bard, J. Am. Chem. Soc., 2014, 136, 13546–21354 CrossRef CAS PubMed.
- J. Han, Y. Zhuo, Y. Q. Chai and R. Yuan, Chem. Commun., 2014, 50, 3367–3369 RSC.
- Y. Zhuo, N. Liao, Y. Q. Chai, G. F. Gui, M. Zhao, J. Han, Y. Xiang and R. Yuan, Anal. Chem., 2014, 86, 1053–1060 CrossRef CAS PubMed.
- H. J. Liu, R. Yuan, Y. Q. Chai, L. Mao, X. Yang, Y. Zhuo and Y. L. Yuan, Talanta, 2011, 84, 387–392 CrossRef CAS PubMed.
- C. M. Cheng, Y. Huang, X. Q. Tian, B. Z. Zheng, Y. Li, H. Y. Yuan, D. Xiao, S. P. Xie and M. M. F. Choi, Anal. Chem., 2012, 84, 4754–4759 CrossRef CAS PubMed.
- M. Hesari, M. S. Workentin and Z. F. Ding, RSC Adv., 2014, 4, 29559–29562 RSC.
- F. Y. Liu, Y. L. Gao, W. Li and J. Shao, RSC Adv., 2014, 4, 34003–34007 RSC.
- S. Y. Deng, J. P. Lei, X. N Yao, Y. Huang, D. J. Lin and H. X. Ju, J. Mater. Chem. C, 2013, 1, 299–306 RSC.
- X. Liu, H. Jiang, J. P. Lei and H. X. Ju, Anal. Chem., 2007, 79, 8055–8060 CrossRef CAS PubMed.
- R. Cui, Y. P. Gu, L. Bao, J. Y. Zhao, B. P. Qi, Z. L. Zhang, Z. X. Xie and D. W. Pang, Anal. Chem., 2012, 84, 8932–8935 CrossRef CAS PubMed.
- L. Wu, J. S. Wang, J. S. Ren, W. Li and X. G. Qu, Chem. Commun., 2013, 49, 5675–5677 RSC.
- N. L. Ritzert, T. T. Truong, G. W. Coates and H. D. Abruna, J. Phys. Chem. C, 2014, 118, 924–932 CAS.
- J. Wang, Y. Shan, W. W. Zhao, J. J. Xu and H. Y. Chen, Anal. Chem., 2011, 83, 4004–4011 CrossRef CAS PubMed.
- Y. Shan, J. J. Xu and H. Y. Chen, Chem. Commun., 2010, 46, 5079–5081 RSC.
- H. Niu, R. Yuan, Y. Q. Chai, L. Mao, Y. L. Yuan, Y. Zhuo, S. R. Yuan and X. Yang, Biosens. Bioelectron., 2011, 26, 3175–3180 CrossRef CAS PubMed.
- G. F. Jie, B. Liu, H. C. Pan, J. J. Zhu and H. Y. Chen, Anal. Chem., 2007, 79, 8055–8060 CrossRef PubMed.
- C. Z. Wang, Y. F. E, L. Z. Fan, Z. H. Wang, H. B. Liu, Y. L. Li, S. H. Yang and Y. L. Li, Adv. Mater., 2007, 19, 3677–3681 CrossRef CAS.
- S. C. Yan, Z. S. Li and Z. G. Zou, Langmuir, 2010, 26, 3894–3901 CrossRef CAS PubMed.
Footnote |
† Electronic supplementary information (ESI) available: Fig. S1 ECL responses of bare GCE (a) and PTC–NH2/GCE (b) in 0.10 M pH 7.0 PBS with 90 mM K2S2O8 under the scanning potential in the range of −2.0–0.0 V. Fig. S2 CV curve of bare GCE with 90 mM K2S2O8. Table S1. Recoveries of DA in hydrochloride injection sample at PTC–NH2/GCE. The toxicity test of PTC–NH2 on a cell using MTT assay. See DOI: 10.1039/c4ra16387g |
|
This journal is © The Royal Society of Chemistry 2015 |