DOI:
10.1039/C4RA14903C
(Paper)
RSC Adv., 2015,
5, 8552-8565
Enhanced CO2 transport properties of membranes by embedding nano-porous zeolite particles into Matrimid®5218 matrix
Received
24th November 2014
, Accepted 24th December 2014
First published on 24th December 2014
Abstract
A novel mixed matrix membrane (MMM) was fabricated by incorporating micro-sized nano-porous sodium zeolite-Y (NaY zeolite) into Matrimid®5218 matrix. The filler and the prepared membranes were characterized by X-ray diffraction (XRD), Fourier transform infrared-attenuated total reflectance (FTIR-ATR), scanning electron microscopy (SEM), and thermal gravimetric and derivative thermal gravimetric (TG/DTG) analyses. The effects of filler content (0–20 wt%), feed pressure (2–12 bar), operating temperature (35–75 °C) and mixed feed gas on CO2/CH4 transport properties of Matrimid/NaY were investigated. The results revealed that the Matrimid/NaY (15 wt%) displayed a CO2 permeability of 17.52 Barrer, more than two-fold increase with respect to the NaY-free counterpart. The corresponding CO2/CH4 selectivity was increased from 36.3 for Matrimid to 43.3 for Matrimid/NaY (15 wt%), (about 20%). The CO2 permselectivities of MMMs were greater than that of the Matrimid over the entire pressure range. As the operating temperature increased from 35 to 75 °C, CH4 permeability increased about 175% and 215% for Matrimid and Matrimid/NaY (15 wt%), respectively. While the CO2 permeability enhanced about 78% and 98%. The corresponding decreases in the CO2/CH4 selectivities were 35.27% and 37.14%, respectively. Moreover, the mixed gas experiment results indicated that CO2 permeability and CO2/CH4 selectivity for all membranes were lower than those of pure gas experiments, but with less severity for MMMs. The best CO2-selective membrane, Matrimid/NaY (15 wt%), represented the CO2 permeability of 15.19 Barrer with CO2/CH4 selectivity of 39.5 for a 10/90 vol% mixture of CO2 and CH4.
1. Introduction
CO2 is commonly found in natural gas streams at levels as high as 80%. The pipeline specifications for natural gas usually require CO2 content less than 2%, since it makes the gas streams acidic and corrosive during transportation and can damage the equipment and pipelines.1 Additionally, reduction of CO2 content decreases the sizes of the gas pipelines and equipment (due to the reduction of volumes of gas which should be transported) and increases the fuel-heating value.2,3 The CO2/CH4 separation has much potential to be employed in many industrial applications such as enhanced oil recovery (EOR), biogas upgrading and landfill gas purification.4,5
As an alternative for conventional methods, membrane gas separation technology has attracted strong attention over the past few years, due to its energy efficiency, higher flexibility, working at low temperature, ease of scale-up and small footprint.6–11 In order to design a successful polymeric membrane system, a polymer has to comply with certain criteria: must be chemically resistant, mechanically/thermally stable and include a reasonable permeability/selectivity.12–14 On the other hand, academic investigators and industrial suppliers have employed organic polymers as asymmetric nonporous membranes that offer many desired properties including low operating cost, ease of fabrication and excellent processability. Generally, polymeric membranes have a trade-off relationship between permeability and selectivity, and usually undergo the Robeson's upper bound limitation.1,15 On the other hand, the gas separation performance of molecular sieve membranes, has been constrained by trade-off between exceptional gas separation properties and endures high stability under harsh operational conditions and major drawbacks such as laborious and costly manufacturing of large surface areas for commercial exploitation.1 As an alternative route, there is a great deal of interest in embedding the filler materials in polymeric membranes to overcome the Robeson limitations while maintaining the mechanical flexibility of the polymeric matrix. This alternative type of membrane, which is known as a mixed matrix membrane (MMM), has appeared in many scientific literatures. Glassy polymers due to their excellent mechanical properties, tunable free cavities and intrinsic high selectivity were extensively employed for fabrication of MMMs.16,17 Polyimides are among the most used types of glassy polymers for CO2 separation.18 Matrimid®5218 is a prominent aromatic polyimide, which originally developed for use in the microelectronics industry, but it has been also employed as a polymer matrix with various inorganic filler such as zeolites and CMS to fabricate MMMs. Furthermore, Matrimid®5218 reveals the outstanding CO2/CH4 permselectivity among various commercially relevant polymers such as polysulfone, TB-bisA-polycarbonate and cellulose acetate.19
Hosseini et al.20 prepared Matrimid/MgO MMMs for CO2/CH4 separation. Their results revealed that the permeability was increased by 75% at 40 wt% loading. However, they observed a reduction in selectivity compared with the neat Matrimid. They also claimed that this behavior was attributed to MgO nanoparticle pore dimensions that are larger than the size ranges of penetrants. The best yield membrane was achieved, after a ten day silver ion treatment of MgO nanoparticles, at 20 wt% loading MMM with increasing the CO2/CH4 selectivity from 29.8 to 42.3. Chung et al.21 examined the Matrimid®5218/C60 MMMs in order to obtain the CO2 and CH4 gas permeation properties. They modified their filler with benzylamine to decline polymer rigidification and concluded that the overall permeability dropped while the CO2/CH4 selectivity remained unchanged. Liu et al.18 embedded the polyzwitterion coated carbon nanotubes (SBMA@CNT) into Matrimid®5218 matrix for CO2/CH4 separation application. Their results indicated that the MMM with approximately 5 wt% SBMA@CNT exhibited the reasonable CO2 permeability of 103 Barrer with CO2/CH4 selectivity of 36. This enhancement may be attributed to the incorporation of the SBMA@CNT composite particles which have two fundamental effects: (1) form interconnected channels for CO2 transport due to the facilitated transport effect of the quaternary ammonium in repeat unit of pSBMA, and (2) SBMA@CNT can develop the water uptake and adjust water state of membrane, which further increases CO2 permeability. Matrimid®5218 based MMMs containing the carbon aerogels were fabricated by Zhang et al.22 and the gas permeation properties of CO2 and CH4 were evaluated. The results demonstrated that the CO2/CH4 ideal selectivity of the membrane with 20% filler loading increased from 34.5 to 47.8. They emphasized that these results may be due to the penetration of polymer chains into the carbon mesopores and vanishing the non-selective voids between the Matrimid®5218/filler. Ahmad et al.16 incorporated the zeolite 4A into Matrimid®9725 matrix using low boiling point solvent dichloromethane (bp = 40 °C). The FESEM images indicated a homogeneous pattern at 20 wt% loading. Moreover, the gas separation properties represented that with increasing of filler loading CO2 permeability increased. The best-yield membrane (Matrimid®5218/zeolite 4A (30 wt%)) CO2 permeability and CO2/N2 selectivity were 48.34 Barrer and 23.35, respectively. Some reports were devoted to evaluate the favorable effects of embedding the β-zeolite into Matrimid®5218 to develop the MMM gas separation properties. Huang et al.23 fabricated Matrimid®5218/β-zeolite MMMs with wide range of filler loading (0–30 wt%). Their results revealed that a significant enhancement in CO2 permeability (up to 121%) over the pure Matrimid®5218, but the CO2/CH4 selectivity decreased remarkably. Their results indicated that increasing the CO2 permeability is not only due to the β-zeolite properties/content, but also depends on the filler uniform distribution in polymer matrix. In another work, Jiang et al.24 coated a polysulfone/β-zeolite (0–30 wt%) ultrathin layer on Matrimid®5218 support to form a composite hollow fibers MMM. The results demonstrated that with 30 wt% filler loading, the CO2/CH4 separation was 50% higher than that of the neat PSF/Matrimid®5218 membrane. They claimed that the increase in separation efficiency may be attributed to the partial pore blockage with the polymer chains and their local rigidification as well as desirable interaction of gas molecules/β-zeolite.
In our previous work,25 the zeolite ZSM-5 filled PSF/Matrimid®5218 MMMs were evaluated and the fabricated MMMs were considered for several gas permeation experiments. In addition, various polymers ratio (from 100% PSF, 70/30 (PSF/PI), 50/50, 30/70 and 100% PI) as well as different filler loadings (0–20 wt%) were examined. The results revealed that the PSF/PI (50/50) membrane with 10 wt% ZSM-5 loading had the reasonable permselectivity among the various prepared MMMs.
In the present study, NaY zeolite was incorporated into Matrimid®5218 matrix in order to form a novel MMMs to improve the CO2 separation properties. The FAU-type zeolite was chosen due to its larger pore size compared to the other types of zeolites, which can facilitates the activated diffusion for the gases molecules.26 Moreover, it provides a superior adsorptive molecular transport by differences in the adsorptivities of the gases.27 Compared with X-type zeolites with Si/Al ratio of 1–1.5, Y-type zeolites has higher Si/Al ratio about 1.5–3.0, which results in higher CO2 adsorption capacity. This is due to the fact that the number of cations which can be coordinated in the Y-types, is smaller than that of X-types.28 The filler and prepared membranes were characterized by XRD, FTIR-ATR, SEM and TG/DTG. Further investigations were performed on the effect of filler content, feed pressure, operating temperature and mixed feed gas on CO2/CH4 transport properties of MMMs. Finally, comparisons were made between the membrane performances of this work and other selected Matrimid-based MMMs.
2. Experimental
2.1. Materials
Matrimid®5218 (3,3′,4,4′-benzophenone tetracarboxylic dianhydride and diaminophenylindane), as a commercial polyimide with good thermal and mechanical properties,29 was purchased from Huntsman. Sodium Y zeolite (NaY) powder, with the Si
:
Al ratio of 2.53, was supplied by Sigma-Aldrich® (Saint Louis, MO, USA). Chloroform (CHCl3, bp 61 °C, >99.9%), was supplied by Merck (Darmstadt, Germany) and used without further purification. Test gases (CH4 and CO2 > 99.9%) were purchased from Sabalan Co., Tehran, Iran.
2.2. Membrane preparation
Generally, using a low boiling point solvents such as CHCl3 with bp = 61 °C for fabrication of MMMs, possess many advantages such as low evaporation time, low energy consumption and reduce the inorganic filler sedimentation. However, the low boiling point solvents suffer from problems associated with fast evaporation from the surface of a casted solution, which results in a membrane with defects and holes. Therefore, the procedure should be combined with controlled evaporation.16 In this regard, chloroform was chosen as the solvent for Matrimid, due to its sufficient evaporation rate to fast formation of a uniform polymer/particle composite after casting procedure. Matrimid powder was dried overnight at 120 °C in a vacuum oven. The NaY powder was also dried overnight at 100 °C in an oven that was followed by further drying for 2 days at 120 °C in a vacuum oven before use. A certain amount of NaY was well mixed into chloroform and the mixture was stirred for 24 h to form a well-dispersed mixture. Then, about 10% of the total Matrimid was added to the NaY/chloroform suspension and further stirred for 6 h. This was done to form a thin layer coating of Matrimid (in a dilute and low viscosity polymer solution) around the solid NaY particles. Afterwards, the remaining second part of Matrimid powder was added to form a 10 wt% solution and the final solution was allowed stirring for 24 h at room temperature. Next, the solution was degassed for 24 h at 25 °C. The final solution was then casted onto a clean glass plate with a casting knife (Elcometer 3580). To control the rate of solvent evaporation, a glass cover was placed over the film and subsequently removed after 24 h. To remove the residual solvent, the prepared membranes were detached from the plate and placed in a 100 °C vacuum oven for 48 h. For preparing the heat treated MMMs, the fabricated membranes were placed into a 150 °C vacuum oven for 48 h by special care for slow initial heating and final cooling rates. The membrane thicknesses were measured using a digital micrometer (Mitutoyo®, Seisakusho, Tokyo, Japan). Finally, the prepared MMMs were stored in a desiccator prior to characterization and gas permeation tests.
3. Results and discussion
3.1. Characterizations
3.1.1. XRD. XRD spectra of Matrimid along with those for NaY zeolite and Matrimid/NaY (15 wt%) are depicted in Fig. 1. These patterns were used to obtain further information about the structural changes in Matrimid microstructure as well as d-spacing during the addition of NaY zeolite. Generally, the XRD spectrum of polymer with large crystalline region reveals high intensity/sharp peaks, while low intensity/broader peaks confirm the amorphous region.30 Matrimid shows two low intensity broad peaks around 2θ = 15° and 22° attributing to the limited crystalline regions in its semi crystalline structure. Pure zeolite Y shows several high intensity/sharp peaks around 2θ = 6.1°, 18°, 22°, 24°, 28°, 30° and 37°. When the solid filler embedded into the polymer matrix, the XRD patterns contain the characteristic peaks of both the zeolite Y and Matrimid. However, the intensity of the main characteristic peak of Matrimid around 2θ = 15° is not distinguishable anymore and the indicative peak at 2θ = 22° is becoming weaker. This confirms that the primary semi crystalline internal structure of Matrimid is being changed throughout the addition of NaY zeolite which results in a drastically decrease of the polymer crystallinity.16 Moreover, for amorphous/semi crystalline glassy polymers, it is recommended to employ the most prominent XRD spectrum peaks for calculating the d-space values.31 Matrimid revealed two amorphous indicative peaks with d-spacings of 5.90 and 4.03 Å. By addition of filler into the polymer matrix, the peak of Matrimid having a d-spacing of 5.90 Å was gently vanished and the d-spacing of 4.03 Å for another peak was increased to 4.79 Å for Matrimid/NaY (15 wt%) membrane. The change in semi crystalline internal structure of Matrimid to an amorphous state and also the shift in d-spacing indicate that the permeability of MMMs could be increased in comparison with neat membrane. The incorporation of NaY zeolite into the Matrimid leads to shift the interstitial chain–chain distance and free volume distribution toward a looser and broader structure. In other words, the permeability enhancement as well as selectivity decrement can be predicted. These effects can be ascribed by the contribution of d-spacings/fractional free volumes enhancement, increase in polymer chains' mobility as well as reduction in chain packing density.32
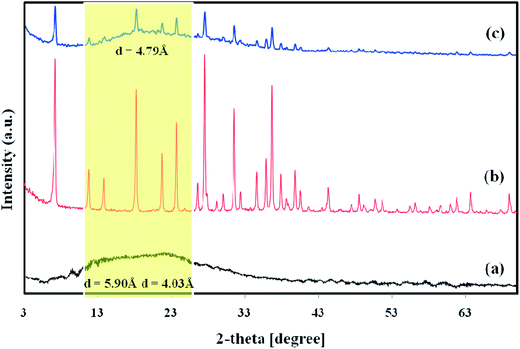 |
| Fig. 1 XRD spectra of (a) Matrimid, (b) NaY zeolite, and (c) Matrimid/NaY (15 wt%). | |
3.1.2. FTIR-ATR. FTIR-ATR spectra of the neat Matrimid, NaY zeolite and Matrimid/NaY (15 wt%) are illustrated in Fig. 2. The indicative peaks of Matrimid are determined in Fig. 2a. As can be seen, the carbonyl groups (C
O stretching bands) in Matrimid spectra are characterized by a peak at 1775 cm−1 which attributed to ketonic group (anti-symmetric stretch vibration of 5-membered imide ring carbonyl) and also another peak at 1670 cm−1 which attributed to imidic group (symmetric stretch vibration of benzophenone carbonyl).3,29 The peaks at 1498 and 1616 cm−1 are the stretching vibrations of aromatic double bonds (C
C stretching bands). The peaks of C–H stretching of aliphatic rings are presented at 2870 and 2962 cm−1, while the aromatic one is appeared at 3057 cm−1. The peaks of C–H stretching due to the CH2 deformation are appeared between 700 to 1000 cm−1. The spectrum of Matrimid demonstrates the characteristic bands at 1256, 1295 cm−1 owing to bending and stretching vibrations of C–N–C bond in the imide ring.33 The N–H bonds of Matrimid are observed at 1348 and 3484 cm−1, which are attributed to secondary amine bending and stretching vibrations, respectively.3 As shown in Fig. 2b, the indicative peaks of NaY zeolite reveal at 464, 660, 1036 cm−1 corresponds to the bending and stretching vibrations of the NaY zeolite structure framework (Si–Al–O). Indeed, the sharp peak with low intensity band at 464 cm−1 can be ascribed to the Si–Al–O bending mode. On the other hand, the peak at 660 cm−1 can be assigned to the Si–Al–O symmetric stretching vibration and has less intensity in comparison with the asymmetric stretching vibration of Si–Al–O bond at 1036 cm−1. Moreover, there is a broad peak at 3456.8 cm−1 in the NaY zeolite spectrum, correspond to the O–H hydrogen bonded to the oxygen ions of the framework. Besides, the intense band at 1670 cm−1 is the characteristic for the interlayer stretching and bending vibration mode in the water molecules.34 From the spectra of Matrimid/NaY (15 wt%) (Fig. 2c), it is evident that all characteristic imide bands are maintained. On the other hand, a new kind of peak emerges at 990–1010 cm−1 for the Matrimid/NaY (15 wt%), which may be attributed to the stretching vibrations of the NaY zeolite structure framework (as seen in Fig. 2b).35 Moreover the peaks of N–H bond observe at 3484 cm−1 and those for C–H bond appear at 2700 to 3100 cm−1, are drastically decreased due to overlapping the O–H stretching band of zeolite (a broad band at 3000–3600 cm−1). Finally, there were no new absorption peaks in the FTIR spectra of MMMs which indicates that chemical interaction between polymer/filler can be refused and NaY particles were physically linked to the Matrimid matrix.
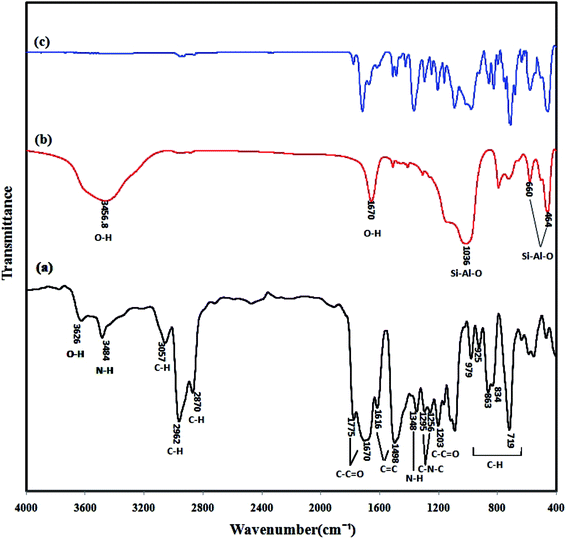 |
| Fig. 2 FTIR-ATR spectra of (a) Matrimid, (b) NaY zeolite, and (c) Matrimid/NaY (15 wt%). | |
3.1.3. SEM. Surface/cross sections of the Matrimid, and its MMMs containing 15 wt% and 20 wt% NaY loadings are illustrated in Fig. 3a–g. As can be seen in Fig. 3a, the dense symmetric cross-section of neat membrane reveals a crater-like pattern.3 The crater-like morphology of MMMs indicated adequate compatibility between the two phases, as it is clear in Fig. 3b–e. In fact, strong interaction of filler/polymer chains creates interfacial stress during fracturing process in liquid nitrogen for SEM analysis. The crater-like structure of MMMs creates due to mentioned stress that can deform the polymer matrix.36 The results confirmed outstanding interfacial adhesion between polymer/filler. As it is shown in Fig. 3d (single particles at the center of individual craters), at the Matrimid/NaY zeolite interface, the polymer layer entirely covered the eternal surface of particles and no detectable voids are recognized. Indeed, the SEM results demonstrate that NaY zeolite particles up to 15 wt% were uniformly distributed within the Matrimid without any aggregation. Furthermore, by incorporating of 15 wt% zeolite Y into Matrimid matrix, the rougher cross sections (in comparison with unfilled Matrimid membrane) and interconnected network morphology, were achieved. However there is some degree of particle aggregations at the highest filler loading (20 wt%), as it is observed in Fig. 3e. Superior filler dispersion into 3D network structure of polymer is a key factor to attain the outstanding separation performance.35 The used zeolite can improve the CO2 transport by greater sorption/diffusion through its micropores. On the other hand, the gas transport can freely accelerate through the space volumes between the aggregated fillers. Conversely, the important factors, which cause the agglomerates to form, are the cumulative effect of aggregates along with the immobilized polymer chains adjacent to the fillers. Hence, the created rigidified/non-interactive zones can hinder the CO2 diffusion pathways through the MMM. Accordingly, there is a trade-off relationship between mentioned factors, which should be considered for design the successful CO2-selective MMMs. Thus, the percolation threshold revealed at higher levels of filler loadings, i.e. 20 wt%, and the non-selective pathways developed through the MMMs. From these observations, it can be inferred that MMMs with 15% of NaY zeolites loading, offers the better conditions. This will be further proven by the performance of fabricated membranes. Fig. 3f and g depict the surface images of MMMs and indicate that the fine uniform filler dispersion was achieved. The size of NaY zeolite particles was acquired from SEM images, as it is observed in Fig. 3h. Results present a regular surface morphology with polyhedral shape, with about 140–1095 nm of particle diameter.
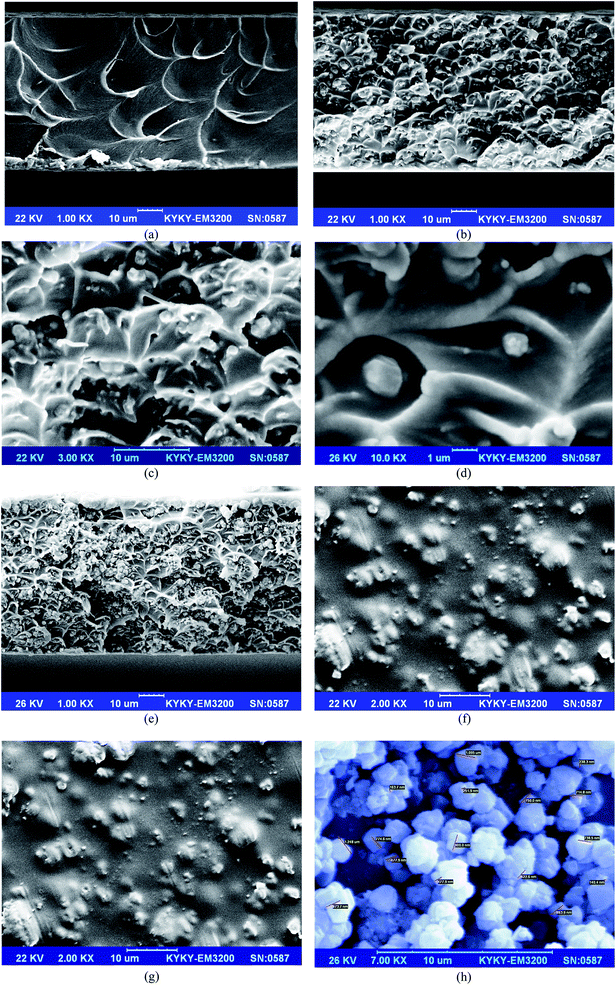 |
| Fig. 3 SEM images of (a) Matrimid, (b) Matrimid/NaY (15 wt%) cross-section, (c) Matrimid/NaY (15 wt%) cross-section at higher magnification, (d) Matrimid/NaY (15 wt%) cross-section at higher magnification (single particles at the center of individual craters), (e) Matrimid/NaY (20 wt%) cross-section, (f) Matrimid/NaY (15 wt%) top surface, (g) Matrimid/NaY (20 wt%) top surface, and (h) NaY zeolite powder. | |
3.1.4. TG/DTG. The thermal properties of Matrimid membrane and Matrimid/NaY zeolite MMMs were characterized by TGA analysis and the results are depicted in Fig. 4. As it is shown, the weight loss (TG) and derivative weight loss (DTG) curves of membranes reveal two-step weight loss: the first step at about 50–300 °C and the second major step at 470–700 °C. These are attributed to evaporation of the adsorbed water/solvent within the zeolite pores and the thermal decomposition of polymer, respectively. The weight reductions for Matrimid membrane are started at about 200 °C and 490.4 °C. On the other hand, for MMMs with various filler contents, the first peaks are appeared between 50–100 °C. In addition, the second peaks begin at 503 °C for 5 wt% filler loading, 511 °C for 10 wt% filler loading, 521.9 °C for 15 wt% filler loading and 536.7 °C for 20 wt% filler loading. With the increase in filler loadings, the values of the first weight loss enhanced from 14.87% (for 5 wt% filler loading) to 20.08% (for 20 wt% filler loading). This is ascribed to the more adsorbed water/solvent in higher zeolite content. Moreover, the thermal decomposition temperatures of MMMs are higher than that of pure Matrimid. In other words, the strong interaction of polymer/filler by forming hydrogen bonds, confines the thermal motion of polymer and by this, the needed energy for polymer chains movement/segmentation enhances. Thus, the thermal stability of membranes would be increased.3,35 A considerable weight loss appears in the range of 500–700 °C corresponds to the decomposition of polymers. All DTG plots of the polyimides reveal two peaks.37 As can be seen in Fig. 4 for Matrimid, the first peak is around 490−550 °C, while the second one is around 572 °C. At this temperature, the most chemical decomposition reactions of the polymer happened. Indeed, this is typically due to conversion of carbonyl group (C
O bonds) to either CO or CO2.38 The second decomposition peak is shifted to 581 °C, 588 °C, 599 °C and 606 °C, for 5 wt%, 10 wt%, 15 wt% and 20 wt% NaY loading, respectively. The residual weights of Matrimid and MMMs with 5–20 wt% filler loadings at 700 °C, are 55.1, 55.3, 56.7, 57.9 and 59.2%, respectively. These results affirm the higher thermal stability of the fabricated MMMs in comparison with the neat Matrimid.
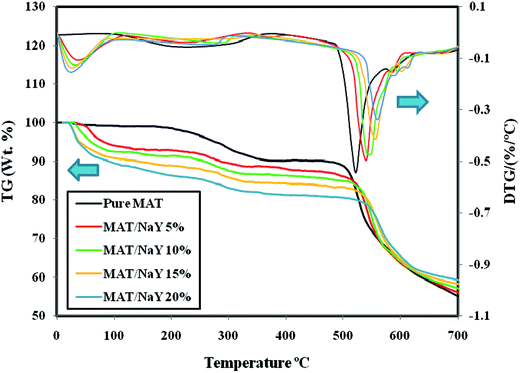 |
| Fig. 4 Thermal degradation of Matrimid membrane and Matrimid/NaY zeolite MMMs. | |
3.2. Pure gas permeation properties
3.2.1. Effect of particle loadings. For comparison and assistant elucidation, pure gas experiment was conducted at temperature of 35 °C and pressure of 2 bar. The results, which were obtained by averaging values from three replicates, are presented in Table 1. The results indicated that with increasing the NaY zeolite content, both CO2 and CH4 permeabilities increased significantly. The CH4 permeability was increased from 0.23 Barrer for Matrimid to 0.41 Barrer for 15 wt% NaY zeolite loading MMMs. This result is attributed to increase in polymer d-spacing and free volume in the presence of the particles, as it is in conformity with the results of XRD and reported by other researchers.39,40 On the other hand, the strong interaction between quadrupole moments of CO2 molecules with oxygen atom of hydroxyl group of NaY zeolite (molecular orientation/hydrogen bonding), results in a considerable CO2 permeability enhancement.3,39 In other words, in the glassy polymers the presence of various kinds of particles can disrupt the chains and structural regularity at polymer/filler interface leading to enhance the polymer free volume. Thus, CO2 with higher condensability and lower kinetic diameter (in comparison with CH4) reveals higher permeability through the fabricated membranes.25,41 Indeed, the Matrimid/NaY (15 wt%) displayed CO2 permeability of 17.52 Barrer, more than two-fold increase with respect to the NaY-free counterpart which showed CO2 permeability of 8.34 Barrer. As it is shown in Table 1, the CO2/CH4 selectivity increased with increasing the filler loading from 36.3 for Matrimid to 43.3 for Matrimid/NaY (15 wt%) (about 20%). This can confirm the superior interfacial adhesion/strong compatibility between polymer matrix/filler as previously elucidated in the results of SEM. Furthermore, this is in conformity with the results of FTIR. The hydroxyl groups of NaY zeolite have a good affinity with the N–H bonds of Matrimid and this can cause a more compatibility with polymer matrix. As can be seen in Fig. 2c, the physical interaction of the N–H group of Matrimid (secondary amine stretching band exists at 3484 cm−1) and the O–H hydrogen groups of NaY zeolites (O–H hydrogen bonded to the oxygen ions of the framework exists at 3456.8 cm−1), creates a strong hydrogen bonding. This may display by vanishing the mentioned peaks in Matrimid/NaY (15 wt%) spectra. Hasegawa et al.28 synthesized various FAU-type zeolite membranes with different Si/AI ratios and evaluated the CO2, N2 and CH4 permeances across the membranes. Their results revealed that the gas permeances were in the order of CO2 > N2 > CH4. They explained that two main factors were affect the results: the former is the strong adsorption rate of CO2 into the NaY zeolite, in comparison with CH4 and N2, and the latter is the smaller kinetic diameter of CO2 (3.3 Å) compared with CH4 (3.8 Å) and N2 (3.64 Å). Moreover, their results indicated that the gas permeation rates are related to the size of zeolitic pores, although the pores are larger than the penetrant molecule sizes. Herein, the strong interaction of CO2 with the NaY zeolite as well as the poor interaction of CH4 molecule with filler results in the high permselectivities of Matrimid/NaY MMMs. In comparison with the neat membrane, CO2 and CH4 permeabilities of Matrimid/NaY (20 wt%) membrane were significantly increased about 164% and 245%, respectively. However, the CO2/CH4 selectivity was drastically decreased to 27.6 (about 24%). This sharp increase/decrease in permeability/selectivity at 20 wt% zeolite loading can be due to reaching a “percolation threshold” (critical volume fraction of the filler) at higher loadings of 20 wt%. In this situation, the continuous channels created with dispersed zeolite phase within the continuous polymer phase and approximately all the fillers are connected to the channels (see Fig. 3e). Hence, the gases can permeate through the two interpenetrating, continuous phases.42 Accordingly, the MMM cannot suitably detect the interacting (CO2) and non-interacting (CH4) gas molecules which indicates a decrease in solubility selectivity.43,44 On the other hand, at higher loadings of 20 wt%, the particle agglomeration results in the rigidified zones with lower mobility of polymer that can enhances the diffusivity selectivity. It is obvious that the membrane performance failed at this filler content.45 Here, for a closer look at these two principal parameters (solubility and diffusivity selectivity), one feels the need to further explore the diffusivity and solubility of gases through the MMMs. The diffusivity and solubility coefficients of gases were calculated using time-lag method and the results are collected in Table 2. The results indicated that gas diffusivity selectivities (DCO2/DCH4) of all the MMMs decreased in comparison with Matrimid membrane. In contrary, the gas solubility selectivities (SCO2/SCH4) of MMMs were drastically increased. The results are in conformity with the outcomes reported by Ghaffari Nik44 and Pechar et al.,46 respectively for FAU/EMT and ZSM-2 zeolites incorporated in polyimide membranes for the CO2/CH4 separation. In fact, the trends of CO2 and CH4 diffusivity and solubility into MMMs were in contrast. The lack of remarkable interaction between CH4 molecules and NaY zeolite resulted in decreasing the CH4 solubility. Besides, an increase in NaY zeolite content results in the enhancement of CH4 diffusivity, because of facilitated movement through the large pores of NaY zeolite without any detectable interaction. On the other hand, CO2 is a quadrupolar molecule and has a favorable electrostatic interaction with Na+ ions in the zeolite pores.44 This great sorption of CO2 in the zeolite may lead to its diffusivity reduction. In other words, NaY zeolite is in the class of large pore zeolites (pore size of 0.74 nm). Therefore, the gas separation mechanism of the NaY zeolite is preferential adsorption of CO2 on the cation sites and it cannot separate the penetrants based on size exclusion. Hence, the CO2 solubility was dramatically increased, when the NaY zeolite embedded into the Matrimid. The CO2 solubility increased from 2.98 up to 7.36 (about 147%) by increasing the NaY zeolite loading in MMM up to 15 wt%, while the CH4 solubility decreased from 0.251 to 0.233 (about 7%). In conclusion, it can be said that the governed separation mechanism of dense Matrimid membranes is solution–diffusion, while the gases diffusion into MMMs is chiefly controlled by CO2 adsorption on the cation sites. Thus, it fortifies the idea that, the changes in transport properties of the prepared membranes can be due to the effect of fillers on membranes structure in a molecular level. These effects are the main reasons of increasing CO2/CH4 selectivities of zeolite filled MMMs compared to the neat polymer membranes.37 As clearly discussed before, for Matrimid/NaY (20 wt%), the gases diffusivity were significantly increased, due to reaching a percolation threshold. Hence, the MMMs ideal selectivity reduced due to diffusivity selectivity drop.29 At this point, the size-selective nature of MMMs was destroyed, due to the uncontrollable increasing of free volume as well as the voids formation.
Table 1 Pure gas permeabilities (in Barrer), ideal selectivities of Matrimid and its MMMs at 35 °C and 2 bar
Membrane |
CO2 permeability |
CH4 permeability |
CO2/CH4 selectivity |
Matrimid |
8.34 |
0.23 |
36.3 |
Matrimid/NaY (5 wt%) |
12.17 |
0.31 |
38.9 |
Matrimid/NaY (10 wt%) |
15.02 |
0.38 |
39.8 |
Matrimid/NaY (15 wt%) |
17.52 |
0.41 |
43.3 |
Matrimid/NaY (20 wt%) |
22.00 |
0.80 |
27.6 |
Table 2 CO2 and CH4 diffusivity and solubility in Matrimid and its MMMs at 35 °C and 2 bar
Membrane |
D × 10−8 [cm2 s−1] |
S × 10−2 [cm3 (STP)/cm3 cm Hg] |
Selectivity |
CO2 |
CH4 |
CO2 |
CH4 |
DCO2/DCH4 |
SCO2/SCH4 |
Matrimid |
2.800 |
0.917 |
2.980 |
0.251 |
3.053 |
11.872 |
Matrimid/NaY (5 wt%) |
2.683 |
1.257 |
4.535 |
0.248 |
2.134 |
18.285 |
Matrimid/NaY (10 wt%) |
2.585 |
1.551 |
5.807 |
0.244 |
1.667 |
23.799 |
Matrimid/NaY (15 wt%) |
2.381 |
1.736 |
7.360 |
0.233 |
1.371 |
31.587 |
Matrimid/NaY (20 wt%) |
3.252 |
2.881 |
6.764 |
0.276 |
1.128 |
24.508 |
3.2.2. Effect of pressure. Fig. 5 depicts the effect of feed pressure (ranging from 2–12 bar) on the permeability/selectivity for Matrimid/NaY membranes with different filler loadings from 0 to 20 wt%. As can be seen, CO2 permeability decreases with increasing the feed pressure, for all the filler loadings from 0 to 20 wt%. The permeability decrease of glassy polymers with increasing the upstream pressure stems from the decreasing solubility of the polymer (gradual saturation of microvoids), which has been predicted by the dual-mode sorption.39 Moreover, for MMMs with filler loadings from 0 to 15 wt%, the CO2 selectivity was superior than that of the Matrimid membrane over the entire pressure range. Since both the kinetic (diameter and moment) and thermodynamic (condensability) properties of CO2 are more appropriate than CH4 for passing through the membranes, it is well-founded that the same behavior could be observed for the CO2/CH4 selectivities. The slight decrease in selectivity of glassy polymer based (cellulose acetate and Matrimid) MMMs with increasing feed pressure at range less that plasticization pressure (the plasticization pressure of Matrimid has been reported between 11–12 bar20,47), has been reported by Visser.48 In other words, increasing the feed pressure leads to more compactness of polymer matrix, which results in decrease in FFV and consequently reduction of gas diffusivity through the membranes. On the other hand, with increasing the pressure, the solubility decreases and the interaction between partially charged CO2 molecules and O-atoms of the hydroxyl groups of NaY zeolite induces a contraction of the framework, which results in reducing the pore sizes.39 As previously mentioned, the noticeable decline in selectivity of the Matrimid/NaY (20 wt%) membrane is due to reaching a percolation threshold of the NaY zeolite particles.
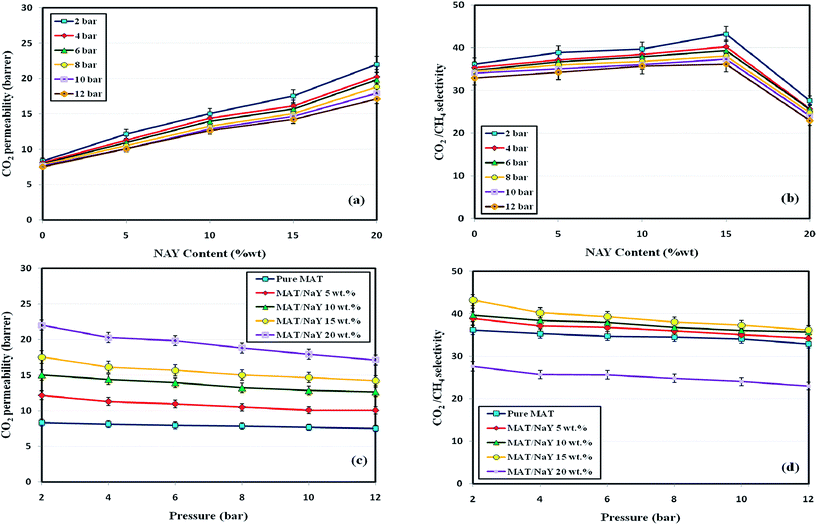 |
| Fig. 5 The effect of feed pressure on CO2 permeability and CO2/CH4 selectivity in term of filler content, at 35 °C. | |
3.2.3. Effect of operating temperature. Fig. 6 illustrates the effect of feed temperature (35–75 °C) on CO2 permeability and CO2/CH4 selectivity of the prepared membranes at 2 bar. The results indicated that CH4 permeability pursues the typical activated diffusion mechanism. The increase in temperature resulted in higher CH4 permeabilities. When the operating temperature increased from 35 to 75 °C, CH4 permeability increased nearly 175% for Matrimid and 215% for Matrimid/NaY (15 wt%), respectively. For CO2 permeability, the improvements were just about 78% and 98% for aforementioned membranes. The corresponding decreases in the CO2/CH4 selectivities were observed as 35.27% and 37.14%, respectively. Fig. 6 demonstrates a typical Arrhenius relation between permeability and temperature. In order to investigate the temperature dependency of the gas permeabilities through the membranes, the Arrhenius equation was applied and the activation energy for permeation (EP) was determined by: |
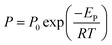 | (1) |
where P, P0, R and T are the permeability of the gas, pre-exponential factor, universal gas constant and the absolute temperature, respectively. It must be noted that P0 is independent of temperature and has the same unit as the permeability (Barrer). Table 3 presents the activation energies for the permeation of CO2 and CH4 through the Matrimid and its MMMs at 2 bar and the comparison with the values reported in other literatures. The two principal factors, which affect the EP of the gases are the penetrants molecular sizes and their interaction with the polymer matrix (solubility).51 The gases with lower EP values, like CO2, reveal a superior permeability. In contrary, non-interacting gases such as CH4 and N2, have higher EP values.16 Indeed, the gas pairs with greater difference in EP show more temperature dependency of permselectivity.52 According to the literature, CH4 has a small negative heat of sorption (ΔHs) that result in slight decrease in solubility with increasing temperature. Moreover, CO2 has a large negative ΔHs which causes a considerable decrease in solubility at higher temperatures.16,53 On the other hand, increasing the temperature causes an increase in mobility of the penetrant molecules as well as the polymer segmental chain mobility. Hence, required energy for diffusion (Ed) is positive in an activated diffusion process and the activated state theory indicates the required Ed is a linear function of molecular size of the gases.53 The solution–diffusion mechanism shows the relation between Ep, ΔHs and Ed with the eqn (2):as temperature increases, two opposite effects are happened: CO2 solubility decreases while its diffusivity enhances considerably. Diffusivity enhancement overcomes the solubility reduction that results in slight increasing in CO2 permeability. This is due to the fact that the temperature effect is more pronounced for gas diffusivity compared to solubility, thus permeability is enhanced. For the gases with a larger kinetic diameter (here CH4), superior positive Ed values results in higher positive Ep values. In this study, the increase in temperature has greater effect on CH4 permeability in comparison with CO2. Therefore, the CO2/CH4 selectivity decreased. Finally, the estimated EP of gases is higher for the MMMs than for Matrimid membranes, as can be seen in Table 3. This may be due to the chain rigidification in the Matrimid/NaY MMMs.54
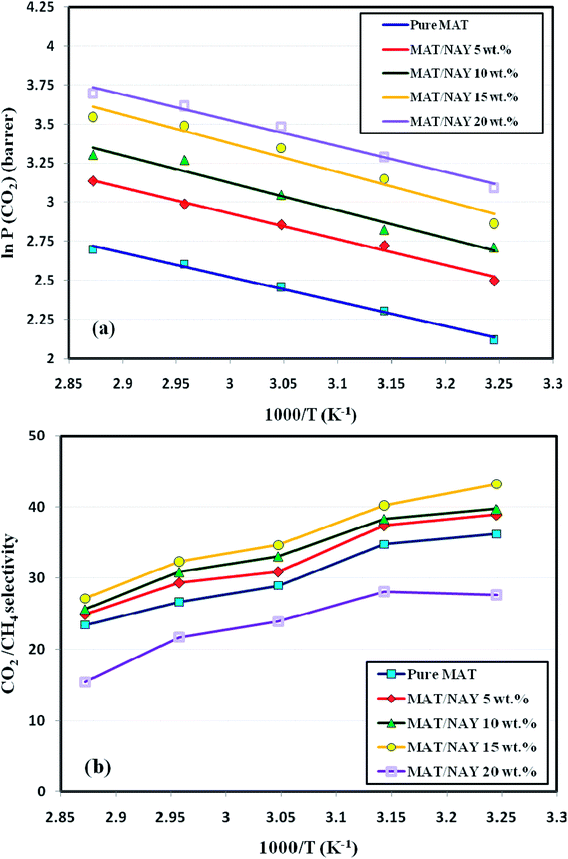 |
| Fig. 6 Effect of operating temperature on (a) CO2 permeability, and (b) CO2/CH4 selectivity of membranes (representative Arrhenius plot ln P(CO2) and α vs. 1/T). | |
Table 3 Activation energies for the permeation of CO2 and CH4 in Matrimid and its MMMs at 2 bar
Membrane |
Ep (kJ mol−1) |
Reference |
CO2 |
CH4 |
Matrimid®5218 |
5.90 |
24.90 |
49 |
Matrimid®5218 |
12.70 |
23.70 |
14 |
Matrimid®5218 |
9.03 |
15.26 |
50 |
Matrimid®5218 |
13.01 |
23.10 |
This work |
Matrimid/NaY (5 wt%) |
13.75 |
23.82 |
This work |
Matrimid/NaY (10 wt%) |
14.59 |
24.24 |
This work |
Matrimid/NaY (15 wt%) |
15.35 |
25.53 |
This work |
Matrimid/NaY (20 wt%) |
13.76 |
26.27 |
This work |
3.3. Mixed gas permeation
The mixed gas permeation of the fabricated membranes at 35 °C (CO2 partial pressure: 0.2 bar, feed composition: 10% CO2–90% CH4) was evaluated and the composition of the permeate gases was measured by the gas chromatography method (GC). The related results are shown in Table 4. The results indicated that CO2 permeability and CO2/CH4 selectivity of all membranes were lower than that of pure gas experiments (see Table 1). For Matrimid membrane, CO2 permeability decreased from 8.34 to 6.66 (around 20%), when the feed gas was a mixture of 10% CO2–90% CH4. Furthermore, the CO2/CH4 selectivity reduced from 36.3 to 30.0 (about 17%). The reduction of CO2/CH4 permselectivity is due to the high concentration of CH4 in the mixture. Generally, the CO2/CH4 competitive sorption causes the decline in polyimide membranes performance under mixed gas conditions.55 In other words, simultaneous presence of CH4 with CO2 in the feed stream makes it difficult for CO2 to pass through the membrane. Chen et al.56 showed that the performance of Matrimid membrane reduced under mixed gas conditions. Their results provided that CO2 permeability and CO2/CH4 selectivity of Matrimid membrane reduced about 22% and 16%, respectively. The presence of CH4 reduces the CO2 solubility coefficient, which results in an overall decline in CO2 permeability.57 For MMMs, a similar to pure Matrimid trend was perceived, but with a less severity. For example, CO2 permeability and CO2/CH4 selectivity of Matrimid/NaY (15 wt%) decreased about 14% and 9%, respectively. Hasegawa et al.58 studied the separation of CO2 using Y-type zeolite membranes. Their results indicated that the diffusivity of small non-interacting gases (i.e. CH4 and N2) are not strongly affected by penetrant adsorption and drastically depends on the size of micropores. In contrary, CO2 has a surface transport explained by the molecules jumping from one adsorption site to another and as a result, this interaction with zeolite pore affects the CO2 diffusivity. In another work, Kusakabe et al.27 investigated the gas permeation properties of the NaY-type zeolite membranes for various equimolar gas mixtures and compared the results with their pure gas experiments. The results showed that although the kinetic diameters of the gases were in order of C3H8 > C2H6 > CH4 > CO2 > H2, but the order of single-component gas permeability was not directly related to the kinetic diameters of the gases. The CO2 permeability was extremely higher than that to the other gases. This effect was ascribed to more CO2 adsorption in NaY-type zeolites in comparison with other gases.28 Incorporation of NaY zeolite into the Matrimid matrix causes more CO2 adsorption and consequently more CO2 solubility. Therefore, the MMMs under mixed gas conditions have a high CO2 adsorption. However, CO2 serves a low portion of the feed gas and hence, its higher solubility results only in a less reduction of MMMs performances in comparison with pristine Matrimid membrane.
Table 4 Mixed gas permeabilities (in Barrer), real selectivities of Matrimid and its MMMs at 35 °C and 2 bar (CO2 partial pressure: 0.2 bar, feed composition: 10% CO2–90% CH4)
Membrane |
CO2 permeability |
CH4 permeability |
CO2/CH4 selectivity |
Matrimid |
6.66 |
0.22 |
30.0 |
Matrimid/NaY (5 wt%) |
9.99 |
0.30 |
33.2 |
Matrimid/NaY (10 wt%) |
12.96 |
0.37 |
35.0 |
Matrimid/NaY (15 wt%) |
15.19 |
0.39 |
39.5 |
Matrimid/NaY (20 wt%) |
18.77 |
0.83 |
22.6 |
3.4. Comparison with other works
Comparisons among the performance of the fabricated membranes and other selected Matrimid®5218 and Matrimid®5218-based membranes are summarized in Table 5. As can be seen, the results of this work are comparable with the literature. Incorporation of the NaY zeolite into Matrimid matrix resulted in considerable enhancement in both CO2 permeability and CO2/CH4 selectivity. Moreover, the results revealed that the MMMs performances are strongly depended on the polymer property, filler structure, filler/polymer interaction, operating temperature, feed pressure and feed composition. Comparison with the other literature results reveals that one of the main advantages of the present MMMs is exploiting benign properties of NaY zeolite for membrane gas separation, due to its outstanding adhesion to Matrimid. In fact, the Matrimid/NaY MMMs showed an attractive CO2 permeability (more than a two-fold growth) along with a satisfactory enhancement in CO2/CH4 selectivity.
Table 5 A comparison between the membrane performances of this work and other selected Matrimid®5218 based MMMs
Membrane |
Filler (wt%) |
T (°C) |
p (bar) |
PCO2 (Barrer) |
αCO2/CH4 |
Ref. |
Mixed gas results. |
Matrimid®5218 |
0 |
25 |
2.0 |
6.20 |
42.80 |
18 |
Matrimid®5218/SBMA@CNT |
10 |
25 |
2.0 |
4.10 |
97.00 |
18 |
Matrimid®5218 |
0 |
30 |
2.0 |
8.84 |
33.98 |
59 |
Matrimid®5218/NHs |
10, 15 |
30 |
2.0 |
12.28, 17.58 |
33.32, 61.04 |
59 |
Matrimid®5218 |
0 |
35 |
2.5–2.7 |
7.29 |
34.71 |
36 and 60 |
Matrimid®5218/Cu–BPY–HFS |
10, 20 |
35 |
2.7 |
7.81, 9.88 |
31.93, 27.62 |
36 |
Matrimid®5218/ZSM-5 |
10 |
35 |
2.5 |
9.01 |
30.03 |
60 |
Matrimid®5218/Meso-ZSM-5 |
10 |
35 |
2.5 |
8.27 |
67.19 |
60 |
Matrimid®5218/Meso-ZSM-5 |
20 |
35 |
2.5 |
8.62 |
66.70 |
60 |
Matrimid®5218/MCM-48 |
10 |
35 |
2.5 |
9.35 |
33.39 |
60 |
Matrimid 5218 |
0 |
35 |
1.0 |
8.34 |
1.22 |
61 |
Matrimid/13X |
43 |
35 |
1.0 |
33.40 |
6.86 |
61 |
Matrimid®5218 |
0 |
35 |
2.0 |
8.34 |
36.27 |
This work |
Matrimid®5218 |
0 |
35 |
2.0 |
6.66a |
30.00a |
This work |
Matrimid®5218/NaY |
10 |
35 |
2.0 |
15.02 |
39.74 |
This work |
Matrimid®5218/NaY |
15 |
35 |
2.0 |
17.52 |
43.27 |
This work |
Matrimid®5218/NaY |
10 |
35 |
2.0 |
12.96a |
35.01a |
This work |
Matrimid®5218/NaY |
15 |
35 |
2.0 |
15.19a |
39.45a |
This work |
4. Conclusions
Matrimid/NaY MMMs were prepared by incorporating NaY zeolite (0–20 wt%) for CO2 separation. XRD patterns showed that the incorporation of NaY zeolite into the Matrimid leaded to a shift in interstitial chain–chain distance and free volume distribution toward a looser and broader structure. In other words, the permeability enhancement can be predictable that these effects can be ascribed to the contribution of d-spacings/fractional free volumes enhancement, increase in polymer chains mobility as well as reduction in chain packing density. FTIR spectra showed a new peak emerges at 990–1010 cm−1 for the Matrimid/NaY, which may be attributed to the stretching vibrations of the NaY zeolite structure framework. There were no new absorption peaks in FTIR spectra of MMMs, which indicated that the NaY particles were physically linked to the Matrimid matrix. SEM observations confirmed outstanding interfacial adhesion between polymer and filler. The external surface of particles was entirely covered by the polymer and no detectable voids were recognized. Indeed, the SEM results demonstrated that NaY zeolite particles up to 15 wt% were uniformly distributed within the Matrimid matrix without any aggregation. TG/DTG analyses indicated that the thermal decomposition temperatures of MMMs were higher than that of pure Matrimid. The strong interaction of polymer/filler by forming hydrogen bonds, confines the thermal motion of polymer and by this, the required energy for polymer chain movement/segmentation enhances. Thus, the thermal stability of membranes would increase. The gas permeation results showed that the best-yield Matrimid/NaY (15 wt%) MMM, revealed the superior CO2 permeability of 17.52 and 15.19 Barrer with CO2/CH4 selectivity of 43.3 and 39.5 for pure and mixed gas experiments, respectively.
Acknowledgements
We hereby would like to thank Hamidreza Sanaeepur (Membrane Processes Research Laboratory (MPRL), Petrochemical Engineering Department, Amirkabir University of Technology (Tehran Polytechnic), Mahshahr Campus, Mahshahr, Iran) for his kind assistance all through the project.
References
- M. Rezakazemi, A. Ebadi Amooghin, M. M. Montazer-Rahmati, A. F. Ismail and T. Matsuura, State-of-the-art membrane based CO2 separation using mixed matrix membranes (MMMs): An overview on current status and future directions, Prog. Polym. Sci., 2014, 39, 817–861 CrossRef CAS PubMed.
- M. Zamani Pedram, M. Omidkhah, A. Ebadi Amooghin and R. Yegani, Facilitated transport by amine-mediated poly(vinyl alcohol) membranes for CO2 removal from natural gas, Polym. Eng. Sci., 2014, 54, 1268–1279 Search PubMed.
- F. Dorosti, M. Omidkhah and R. Abedini, Fabrication and characterization of Matrimid/MIL-53 mixed matrix membranes for CO2/CH4 separation, Chem. Eng. Res. Des., 2014, 92, 2439–2448 CrossRef CAS PubMed.
- A. Ebadi Amooghin, H. Sanaeepur, A. R. Moghadassi, A. Kargari, D. Ghanbari and Z. Sheikhi Mehrabadi, Modification of ABS membrane by PEG for capturing carbon dioxide from CO2/N2 streams, Sep. Sci. Technol., 2010, 45, 1385–1394 CrossRef.
- Y. Zhang, J. Sunarsoc, S. Liud and R. Wang, Current status and development of membranes for CO2/CH4 separation: A review, Int. J. Greenhouse Gas Control, 2013, 12, 84–107 CrossRef CAS PubMed.
- M. Zamani Pedram, M. Omidkhah and A. Ebadi Amooghin, Synthesis and characterization of diethanolamine-impregnated cross-linked polyvinylalcohol/glutaraldehyde membranes for CO2/CH4 separation, J. Ind. Eng. Chem., 2014, 20, 74–82 CrossRef CAS PubMed.
- A. Ebadi Amooghin, M. Zamani Pedram, M. Omidkhah and R. Yegani, A novel CO2-selective synthesized amine-impregnated cross-linked polyvinylalcohol/glutaraldehyde membrane: fabrication, characterization, and gas permeation study, Greenhouse Gases: Sci. Technol., 2013, 3, 378–391 CrossRef.
- A. Ebadi Amooghin, H. Sanaeepur, A. Kargari and A. Moghadassi, Direct determination of concentration-dependent diffusion coefficient in polymeric membranes based on the Frisch method, Sep. Purif. Technol., 2011, 82, 102–113 CrossRef PubMed.
- A. Ebadi Amooghin, P. Moradi Shehni, A. Ghadimi, M. Sadrzadeh and T. Mohammadi, Mathematical modeling of mass transfer in multicomponent gas mixture across the synthesized composite polymeric membrane, J. Ind. Eng. Chem., 2013, 19, 870–885 CrossRef CAS PubMed.
- R. Abedini, M. Omidkhah and F. Dorosti, Highly permeable poly(4-methyl-1-pentyne)/NH2-MIL 53 (Al) mixed matrix membrane for CO2/CH4 Separation, RSC Adv., 2014, 4, 36522–36537 RSC.
- P. Moradi Shehni, A. Ebadi Amooghin, A. Ghadimi, M. Sadrzadeh and T. Mohammadi, Modeling of unsteady–state permeation of gas mixture through a self-synthesized PDMS membranes, Sep. Purif. Technol., 2011, 76, 385–399 CrossRef CAS PubMed.
- H. Sanaeepur, A. Ebadi Amooghin, A. Moghadassi, A. Kargari, S. Moradi and D. Ghanbari, A novel acrylonitrile–butadiene–styrene/poly(ethylene glycol) membrane: preparation, characterization, and gas permeation study, Polym. Adv. Technol., 2012, 23, 1207–1218 CrossRef CAS.
- H. Sanaeepur, A. Ebadi Amooghin, A. Moghadassi and A. Kargari, Preparation and characterization of acrylonitrile–butadiene–styrene/poly(vinyl acetate) membrane for CO2 removal, Sep. Purif. Technol., 2011, 80, 499–508 CrossRef CAS PubMed.
- B. Zornoza, C. Tellez and J. Coronas, Mixed matrix membranes comprising glassy polymers and dispersed mesoporous silica spheres for gas separation, J. Membr. Sci., 2011, 368, 100–109 CrossRef CAS PubMed.
- L. M. Robeson, Correlation of separation factor versus permeability for polymeric membranes, J. Membr. Sci., 1991, 62, 165–185 CrossRef CAS.
- J. Ahmad and M. B. Hagg, Development of Matrimid/zeolite 4A mixed matrix membranes using low boiling point solvent, Sep. Purif. Technol., 2013, 115, 190–197 CrossRef CAS PubMed.
- S. L. Liu, L. Shao, M. L. Chua, C. H. Lau, H. Wang and S. Quan, Recent progress in the design of advanced PEO-containing membranes for CO2 removal, Prog. Polym. Sci., 2013, 38, 1089–1120 CrossRef CAS PubMed.
- Y. Liu, D. Peng, G. He, S. Wang, Y. Li, H. Wu and Z. Jiang, Enhanced CO2 Permeability of Membranes by Incorporating Polyzwitterion@CNT Composite Particles into Polyimide Matrix, ACS Appl. Mater. Interfaces, 2014, 6, 13051–13060 CAS.
- D. F. Sanders, Z. P. Smith, R. Guo, L. M. Robeson, J. E. McGrath, D. R. Paul and B. D. Freeman, Energy-efficient polymeric gas separation membranes for a sustainable future: A review, Polymer, 2013, 54, 4729–4761 CrossRef CAS PubMed.
- S. S. Hosseini, Y. Li, T. S. Chung and Y. Liu, Enhanced gas separation performance of nanocomposite membranes using MgO nanoparticles, J. Membr. Sci., 2007, 302, 207–217 CrossRef CAS PubMed.
- T. S. Chung, S. S. Chan, R. Wang, Z. Lu and C. He, Characterization of permeability and sorption in Matrimid/C60 mixed matrix membranes, J. Membr. Sci., 2003, 211, 91–99 CrossRef CAS.
- Y. Zhang, I. H. Musselman, J. P. Ferraris and K. J. Balkus Jr, Gas permeability properties of mixed-matrix matrimid membranes containing a carbon aerogel: a material with both micropores and mesopores, Ind. Eng. Chem. Res., 2008, 47, 2794–2802 CrossRef CAS.
- Z. Huang, J. F. Su, X. Q. Su, Y. H. Guo, L. J. Teng and C. M. Yang, Preparation and permeation characterization of β-zeolite-incorporated composite membranes, J. Appl. Polym. Sci., 2009, 112, 9–18 CrossRef CAS.
- L. Y. Jiang, T. S. Chung and S. Kulprathipanja, Fabrication of mixed matrix hollow fibers with intimate polymer–zeolite interface for gas separation, AIChE J., 2006, 52, 2898–2908 CrossRef CAS.
- F. Dorosti, M. Omidkhah, M. Z. Pedram and F. Moghadam, Fabrication and characterization of polysulfone/polyimide–zeolite mixed matrix membrane for gas separation, Chem.–Eng. J., 2011, 171, 1469–1476 CrossRef CAS PubMed.
- G. Dong, H. Li and V. Chen, Challenges and opportunities for mixed-matrix membranes for gas separation, J. Mater. Chem. A, 2013, 1, 4610–4630 CAS.
- K. Kusakabe, T. Kuroda, K. Uchino, Y. Hasegawa and S. Morooka, Gas Permeation Properties of Ion-Exchanged Faujasite-Type Zeolite Membranes, AIChE J., 1999, 45, 1220–1226 CrossRef CAS.
- Y. Hasegawa, T. Tanaka, K. Watanabe, B. H. Jeong, K. Kusakabe and S. Morooka, Separation of CO2–CH4 and CO2–N2 systems using ion-exchanged FAU-type zeolite membranes with different Si/A1 ratios, Korean J. Chem. Eng., 2002, 19, 309–313 CrossRef CAS.
- F. Moghadam, M. R. Omidkhah, E. Vasheghani-Farahani, M. Z. Pedram and F. Dorosti, The effect of TiO2 nanoparticles on gas transport properties of Matrimid®5218-based mixed matrix membranes, Sep. Purif. Technol., 2011, 77, 128–136 CrossRef CAS PubMed.
- J. H. Kim and Y. M. Lee, Gas permeation properties of poly(amide-6-b-ethylene oxide)–silica hybrid membranes, J. Membr. Sci., 2001, 193, 209–225 CrossRef CAS.
- F. Aziz and A. F. Ismail, Preparation and characterization of cross-linked Matrimid® membranes using para-phenylenediamine for O2/N2 separation, Sep. Purif. Technol., 2010, 73, 421–428 CrossRef CAS PubMed.
- S. S. Hosseini, M. M. Teoh and T. S. Chung, Hydrogen separation and purification in membranes of miscible polymer blends with interpenetration networks, Polymer, 2008, 49, 1594–1603 CrossRef CAS PubMed.
- M. Inagaki, N. Ohta and Y. Hishiyama, Aromatic Polyimides as Carbon Precursors, Carbon, 2013, 61, 1–21 CrossRef CAS PubMed.
- S. S. Rayalu, J. S. Udhoji, S. U. Meshram, R. R. Naidu and S. Devotta, Estimation of crystallinity in flyash-based zeolite-A using XRD and IR spectroscopy, Curr. Sci., 2005, 89, 2147–2151 CAS.
- L. Lin, A. Wang, M. Dong, Y. Zhang, B. He and H. Li, Sulfur removal from fuel using zeolites/polyimide mixed matrix membrane adsorbents, J. Hazard. Mater., 2012, 203–204, 204–212 CrossRef CAS PubMed.
- Y. Zhang, I. H. Musselman, J. P. Ferraris and K. J. Balkus Jr, Gas permeability properties of Matrimid® membranes containing the metal–organic framework Cu–BPY–HFS, J. Membr. Sci., 2008, 313, 170–181 CrossRef CAS PubMed.
- X. Y. Chen, H. Vinh-Thang, D. Rodrigue and S. Kaliaguine, Amine-functionalized mil-53 metal–organic framework in polyimide mixed matrix membranes for CO2/CH4 separation, Ind. Eng. Chem. Res., 2012, 51, 6895–6906 CrossRef CAS.
- S. Zhao, Z. Q. Shi, C. Y. Wang and M. M. Chen, Structure and surface elemental state analysis of polyimide resin film after carbonization and graphitization, J. Appl. Polym. Sci., 2008, 108, 1852–1856 CrossRef CAS.
- S. Shahid and K. Nijmeijer, Performance and plasticization behavior of polymer–MOF membranes for gas separation at elevated pressures, J. Membr. Sci., 2014, 470, 166–177 CrossRef CAS PubMed.
- S. Basu, A. Cano-Odena and I. F. J. Vankelecom, Asymmetric Matrimid (R)/Cu-3(BTC) (2) mixed-matrix membranes for gas separations, J. Membr. Sci., 2010, 362, 478–487 CrossRef CAS PubMed.
- R. Abedini, M. Omidkhah and F. Dorosti, Hydrogen separation and purification with poly(4-methyl-1-pentyne)/MIL 53 mixed matrix membrane based on reverse selectivity, Int. J. Hydrogen Energy, 2014, 56, 7897–7909 CrossRef PubMed.
- R. W. Baker, in Membrane technology and applications, John Wiley & Sons Ltd., West Sussex, United Kingdom, 3rd edn, 2012 Search PubMed.
- H. Dogan and N. D. Hilmioglu, Zeolite-filled regenerated cellulose membranes for pervaporative dehydration of glycerol, Vacuum, 2010, 84, 1123–1132 CrossRef CAS PubMed.
- O. G. Nik, X. Y. Chen and S. Kaliaguine, Amine-functionalized zeolite FAU/EMT-polyimide mixed matrix membranes for CO2/CH4 separation, J. Membr. Sci., 2011, 379, 468–478 CrossRef CAS PubMed.
- O. G. Nik, X. Y. Chen and S. Kaliaguine, Functionalized metal organic framework–polyimide mixed matrix membranes for CO2/CH4 separation, J. Membr. Sci., 2012, 413–414, 48–61 CrossRef CAS PubMed.
- T. W. Pechar, M. Tsapatsis, E. Marand and R. Davis, Preparation and characterization of a glassy fluorinated polyimide zeolite-mixed matrix membrane, Desalination, 2002, 146, 3–9 CrossRef CAS.
- T. M. Wing, Asymmetric gas separation membranes with superior capabilities for gas separation, US Pat. WO 2008/076599, 2008.
- T. Visser, Mixed Matrix Plasticization Phenomena in Asymmetric Membranes, Ph.D. Thesis, University of Twente, The Netherlands, 2006.
- S. Shishatskiy, C. Nistor, M. Popa, S. P. Nunes and K. V. Peinemann, Polyimide asymmetric membranes for hydrogen separation: influence of formation conditions on gas transport properties, Adv. Eng. Mater., 2006, 8, 390–397 CrossRef CAS.
- H. Y. Zhao, Y. M. Cao, X. L. Ding, M. Q. Zhou and Q. Yuan, Effects of cross-linkers with different molecular weights in cross-linked Matrimid 5218 and test temperature on gas transport properties, J. Membr. Sci., 2008, 323, 176–184 CrossRef CAS PubMed.
- R. Pal, Permeation models for mixed matrix membranes, J. Colloid Interface Sci., 2008, 317, 191–198 CrossRef CAS PubMed.
- J. A. de Sales, P. S. O. Patricio, J. C. Machado, G. G. Silva and D. Windmoller, Systematic investigation of the effects of temperature and pressure on gas transport through polyurethane/poly(methylmethacrylate) phase-separated blends, J. Membr. Sci., 2008, 310, 129–140 CrossRef CAS PubMed.
- M. Sadrzadeh, K. Shahidi and T. Mohammadi, Effect of operating parameters on pure and mixed gas permeation properties of a synthesized composite PDMS/PA membrane, J. Membr. Sci., 2009, 342, 327–340 CrossRef CAS PubMed.
- M. Moaddeb and W. J. Koros, Gas transport properties of thin polymeric membranes in the presence of silicon dioxide particles, J. Membr. Sci., 1997, 125, 143–163 CrossRef CAS.
- C. A. Scholes, G. W. Stevens and S. E. Kentish, Membrane gas separation applications in natural gas processing, Fuel, 2012, 96, 15–28 CrossRef CAS PubMed.
- G. Q. Chen, C. A. Scholes, G. G. Qiao and S. E. Kentish, Water vapor permeation in polyimide membranes, J. Membr. Sci., 2011, 379, 479–487 CrossRef CAS PubMed.
- P. S. Tin, T. S. Chung, Y. Liu, R. Wang, S. L. Liu and K. P. Pramoda, Effects of cross-linking modification on gas separation performance of Matrimid membranes, J. Membr. Sci., 2003, 225, 77–90 CrossRef CAS PubMed.
- Y. Hasegawa, K. Watanabe, K. Kusakabe and S. Morooka, The separation of CO2 using Y-type zeolite membranes ion-exchanged with alkali metal cations, Sep. Purif. Technol., 2001, 22–23, 319–325 CrossRef.
- X. Li, M. Wang, S. Wang, Y. Li, Z. Jiang, R. Guo, H. Wu, X. Z. Cao, J. Yang and B. Wang, Constructing CO2 transport passageways in Matrimids membranes using nanohydrogels for efficient carbon capture, J. Membr. Sci., 2015, 474, 156–166 CrossRef CAS PubMed.
- Y. Zhang, K. J. Balkus Jr, I. H. Musselman and J. P. Ferraris, Mixed-matrix membranes composed of Matrimid® and mesoporous ZSM-5 nanoparticles, J. Membr. Sci., 2008, 325, 28–39 CrossRef CAS PubMed.
- H. H. Yong, H. C. Park, Y. S. Kang, J. Won and W. N. Kim, Zeolite-filled polyimide membrane containing 2,4,6-triaminopyrimidine, J. Membr. Sci., 2001, 188, 151–163 CrossRef CAS.
|
This journal is © The Royal Society of Chemistry 2015 |