DOI:
10.1039/C4RA14850A
(Paper)
RSC Adv., 2015,
5, 5312-5320
Thienopyrrole and selenophenopyrrole donor fused with benzotriazole acceptor: microwave assisted synthesis and electrochemical polymerization†
Received
19th November 2014
, Accepted 4th December 2014
First published on 4th December 2014
Abstract
Thieno-/selenophenopyrrole fused substituted benzotriazoles were synthesized by microwave assisted cyclization which dramatically reduced the reaction time. Incorporation of benzotriazole as an acceptor moiety in a fused system was studied for the first time. Single crystal X-ray crystallographic studies were performed on thienopyrrole and selenophenopyrrole fused benzotriazoles 4, 5, 6 and 7. Compound 4 and 5 crystalize via hydrogen bonding with an ethyl acetate molecule from the solvent. N-Methyl substituted compounds 6 and 7 were electrochemically polymerized and characterized by spectroelectrochemistry. The effects of changing the heteroatom of the terminally fused heterocycles on their photophysical and electrochemical properties were also explored in this report. Emission spectra of compound 6 and 7 showed solvatochromic effects with a large Stokes shift.
Introduction
Organic π-conjugated materials are of great importance for the development of organic electronic devices1 such as organic light emitting diodes (OLEDs),2–4 organic field effect transistors (OFETs)5–7 and organic solar cells (OSCs).8–10 Polycyclic aromatic hydrocarbons (PAHs), such as linear acene derivatives, were extensively studied as organic semiconductors.11,12 Long conjugated systems with multifused aromatic and heteroaromatic rings can be considered as ladder-type conjugated polymers.13 These ladder-type conjugated polymers are expected to be highly planar in nature, thereby resulting in rigid structural framework that helps in effective extended π-conjugation, dense molecular packing advantageous for getting higher charge carrier mobility. Additionally, ladder-type polymers are expected to afford a high thermal stability and a high resistance to chemical degradation. Ladder-type π-conjugated systems incorporating various heteroatoms (S, B, Si and N) have been synthesized and investigated as potential π-functional material.14
Low-band-gap (LBG) conjugated polymers are important category of conjugated polymers because their unique electronic properties and ability to absorb solar radiation efficiently. Various approaches have been used to obtain LBG polymers which include (i) the donor–acceptor (D–A) concept,10 (ii) fusion of aromatic ring(s) into the monomer structure to increase the quinoidal character of the resulting conjugated polymers and (iii) an atomistic approach.15,16 The donor–acceptor (D–A) concept is based on coupling of an electron rich donor (D) unit with an electron deficient acceptor (A) unit in an alternate manner in the polymer backbone. The fused ring systems incorporating electron rich moieties with electron deficient moieties are of growing interest in the field of organic electronics due to very good π-stacking in their solid state packing.17 Hsu and co-workers18 reported multifused systems using benzothiadiazole (BDT), benzoselenadiazole (BDS), and quinoxaline (QX) as the central acceptor units fused with dithienopyrrole as donor at the terminal positions. Nakamura et al.19 explored the synthesis and structure–property relationship of π-extended thiadiazoles fused with thienopyrrole and indole moieties. Balaji et al.20 reported the electropolymerization of thiadiazole fused indolo[2,3-a]carbazole to get stable polymer. But no spectroelectrochemical characterization was performed on any polydisperse systems of these multifused conjugated molecules.
Benzotriazole21 (BTAz) is explored in few reports22–26 as an acceptor in D–A–D conjugated system like the other popular accepting units BDT, BDS, and QX. Very few BTAz containing electroactive small molecules and polymers are reported to show significantly interesting properties.27 While the BDT and BDS units have been very recently reported to form fused conjugated D–A–D systems, there is no report on any thieneopyrrole fused BTAz. Herein we report the synthesis, electropolymerization and the optoelectronic properties of thieneopyrrole and selenophenopyrrole fused BTAz.
Results and discussion
Compound 1 (ref. 28) was subjected to Stille coupling reaction with tributyl(2-thienyl)tin29 to obtain compound 2 (Scheme 1). In the next step double reductive cyclization was carried out of compound 2 with PPh3 in o-dichlorobenzene solvent under microwave condition at the refluxing temperature for 15 min to get compound 4 in 67% yield. Compound 5 was synthesized by using Stille coupling of tributyl(2-selenyl)tin30 and compound 1 followed by the double reductive cyclization with 68% yield. Earlier reports of this type of double reductive cyclization using PPh3 and P(OEt)3 take longer time than microwave condition. Microwave irradiation generally speed up chemical reactions. The rate of chemical reactions can be ascertained on the basis of Arrhenius equation K = Ae−ΔG/RT. Microwave irradiation can induce an increase in molecular vibrations thereby increasing the pre-exponential factor A resulting in speed up of the reaction. Alternatively it can affect the free energy of activation leading to decrease in reaction time. The substrates bearing strongly electron-withdrawing substituents helps in the reductive deoxygenation of the nitro groups thereby resulting in rate enhancement of reductive cyclization reaction. In the earlier reports of thiadiazolo fused compounds the reaction yields were higher than in this report of benzotriazolo fused compounds. It can be attributed to the better electron-withdrawing ability of BDT than that of BTAz. We also found that microwave assisted synthesis reduces the reaction time dramatically for the key reductive cyclization step.31,32 N-alkylation of compounds 4 and 5 was carried out using methyl iodide in the presence of sodium hydroxide in DMF to get compound 6 and 7 in 66% and 75% yields, respectively. All of the compounds were fully characterized by using 1H and 13C NMR and mass spectrometry.
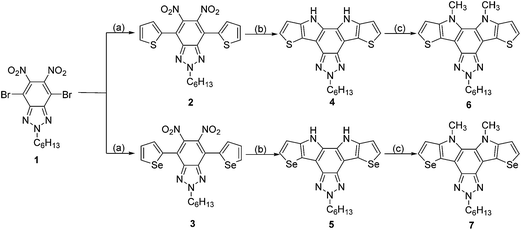 |
| Scheme 1 Synthesis of benzotriazoles 6 and 7. Reagents and conditions: (a) Pd(PPh3)4, tributyl(2-thienyl)tin for 2, tributyl(2-selenyl)tin for 3, toluene, reflux; (b) PPh3, o-dichlorobenzene, microwave, 180 °C, 15 min; (c) iodomethane, NaOH, DMF, rt. | |
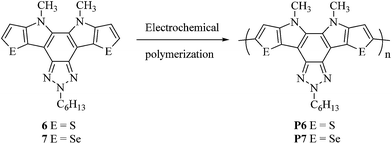 |
| Scheme 2 Synthetic route to the polymers. | |
Structural properties
The molecular structures of compound 4, 5, 6, and 7 were analyzed by single-crystal X-ray diffraction (SCXRD) technique. Single crystals of compound 4 and 5 were obtained by the slow evaporation method from their hexane/ethyl acetate (1/1) solutions, while that of compound 6 and 7 were obtained from slow evaporation from their hexane/DCM (1/1) solvent systems. SCXRD showed that these molecules are nearly planar and crystallized in the monoclinic space group. The dihedral angles between the central BTAz and aromatic heterole rings (C9, C10, C11 and C20; C16, C15, C14 and C19) in 6 (−178.0 and −170.2°) and in 7 (169.3 and 177.3°), respectively, implies a nearly planar framework due to the presence of nitrogen bridges in the fused system.
Compounds 4 and 5 co-crystallized with the ethyl acetate molecule that apparently came from the solvent system, in a 1
:
1 stoichiometry. In the crystal of compound 4 and 5 the ethyl acetate molecules were trapped by bifurcated N–H⋯O hydrogen bonding (2.021 Å & 2.106 Å) and (2.054 Å & 2.064 Å), respectively (Fig. 1). Compound 4 and 5 are very planar, whereas 6 and 7 are slightly puckered due to the steric interaction between the adjacent methyl groups on the nitrogen atoms (Fig. 2).
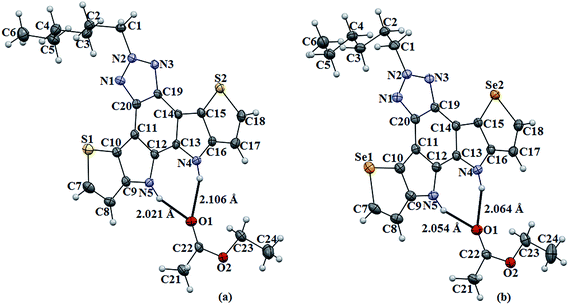 |
| Fig. 1 ORTEP representation of (a) 4 and (b) 5 (thermal ellipsoids are drawn at 50% probability level). | |
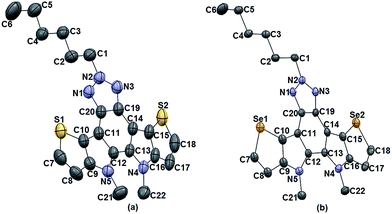 |
| Fig. 2 ORTEP representation of (a) 6 and (b) 7 (thermal ellipsoids are drawn at 50% probability level). H-atoms are omitted for clarity. | |
As shown in Fig. S1 and S2† compound 6 and 7 exhibit π-stacking interaction with a distance of about 3.507 Å and 3.469 Å, respectively. Both of them showed similar kind of interlocked packing pattern. Se⋯Se interaction with the distance of 3.771 Å and 3.761 Å respectively, in compound 7 was observed but no S⋯S interaction was present for compound 6 (Fig. 3).
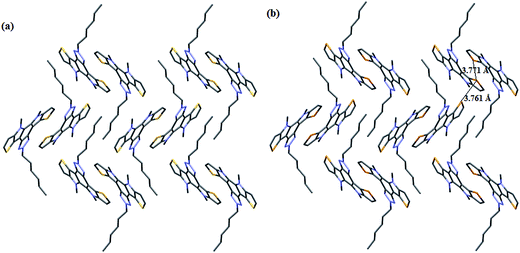 |
| Fig. 3 Crystal packing of (a) 6 and (b) 7. | |
Photophysical properties
The absorption spectra of these fused compounds were recorded in toluene (Fig. 4) and the corresponding data are summarized in Table 1. Both of them showed similar kind of absorption spectra in toluene solution with well defined vibronic structures in the π–π* transition bands. The appearance of multiple maxima in the UV region indicates the presence of coplanar and rigid conformation of fused ring system. Thienopyrole-fused BDT, BDS, or QX all of them shows two types of absorption bands; one is due to the π–π* transition in the range of 300–370 nm, the another is due to the charge transfer (CT) transition in the absorption region of 400–500 nm. On the contrary thienopyrole-fused BTAz absorbs only in the lower wavelength range that arises due to π–π* transition, no characteristic CT band was observed. It can be explained on the basis of the fact that BTAz is poorly electron withdrawing group than BDT, BDS, or QX. The presence of BTAz as an acceptor does not lead to any CT transition in the fused systems.
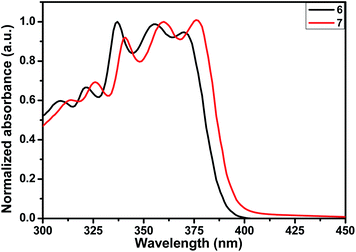 |
| Fig. 4 Normalized absorption spectra of 6 and 7 in toluene. | |
Table 1 Optical and electrochemical properties of fused systems 6 and 7
Compound |
λabs (nm) |
λem (nm) |
Stokes shift (nm) |
EHOMOa (eV) |
ELUMOb (eV) |
Eoptgc (eV) |
The HOMO energy levels were obtained from the equation EHOMO = −(4.8 − E1/2ferrocene + Eoxonset) eV. LUMO energy levels were calculated from the equation ELUMO = EHOMO + Eoptg. Calculated from Eoptg = hc/λonset. |
6 |
308, 321, 336, 354, 368 |
461 |
93 |
−5.33 |
−2.14 |
3.19 |
7 |
312, 324, 340, 358, 374 |
460 |
86 |
−5.16 |
−2.04 |
3.12 |
The absorption maxima for compound 7 is slightly shifted to the longer wavelength compared to that of compound 6. It can be explained on the basis of the fact that more electron rich selenophene ring is fused in the case of compound 7.
To study the effects of solvents on the photophysical properties, we have recorded the UV-vis and emission spectra in various nonpolar to polar solvents. Slight changes were observed for compound 6 and 7 in UV-vis spectra with solvent polarity (Fig. S3†). A very prominent solvatochromic effect was observed in the case of emission spectra of these two systems. With increase in the solvent polarity from toluene to DMSO, the emission maxima showed bathochromic shifts of 47 (422 to 469 nm) and 52 nm (420 to 472 nm) for compound 6 and 7, respectively (Fig. 5). Compounds 6 and 7 also showed large Stokes shift of 93 and 86 nm, respectively. Such kind of large Stokes shift and solvatochromic effect strongly supports a more polar electronic structures in the excited state than in the ground state.
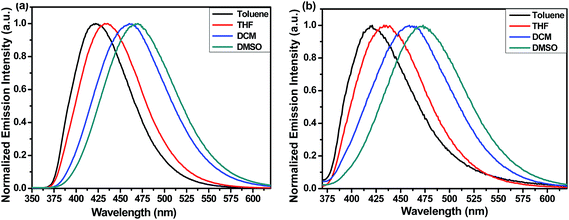 |
| Fig. 5 Normalized emission spectra of (a) 6 and (b) 7 in different solvents. | |
Electrochemical properties
The electrochemical properties of compound 4, 5, 6 and 7 were examined by cyclic voltammetry in dry acetonitrile using 0.1 M TBAPC as electrolyte. Oxidation onset of compound 6 (Fig. 6a) and 7 (Fig. 6c) were found to be 0.89 and 0.72 V, respectively. Whereas that of compound 4 and 5 (Fig. S5†) were found to be at 0.60 and 0.55 V, respectively. The HOMO energy levels were calculated to be −3.83, −3.88, −5.33 and −5.16 eV for compound 4, 5, 6 and 7, respectively. Oxidation peaks of unsubstituted 4 and 5 showed lower oxidation potential than the alkylated compound 6 and 7. The oxidation onset value (Eoxonset) shifted to lower potential on going from 6 to 7, clearly reflecting the difference of electron donating ability of the thiophene and selenophene moieties. Thus introduction of more electron rich fused selenophene rings in compound 7 resulted in the elevation of HOMO level compared to that of thiophene containing compound 6. This suggests that the difference in the donor ability of the thienopyrrole and selenophenopyrrole plays an important role in the electrochemistry of these compounds. The LUMO energy levels can be calculated by subtraction of the band gap values from the HOMO energy level values and they were found to be −2.14 and −2.04 eV for compounds 6 and 7, respectively (Table 1).
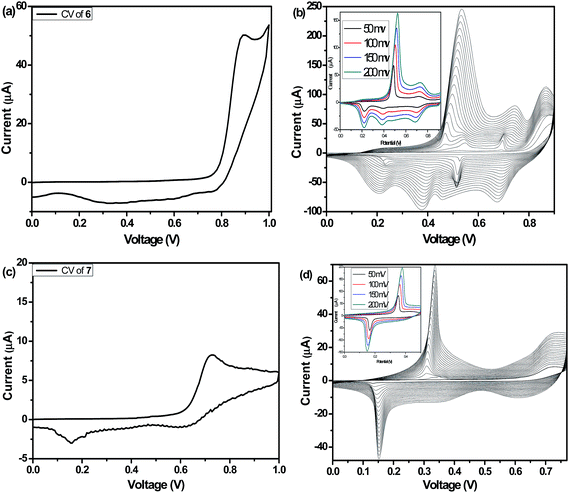 |
| Fig. 6 CV of (a) 6 and (c) 7 in ACN and 0.1 M TBAPC on a Pt electrode at a scan rate of 50 mV s−1 vs. Ag/AgCl wire. Multisweep electrochemical polymerization of (b) 6 and (d) 7 on a Pt electrode in ACN and 0.1 M TBAPC at a scan rate of 50 mV s−1 vs. Ag/AgCl wire. (Inset) CV of (b) P6 and (d) P7 produced in monomer free ACN and 0.1 M TBAPC as a function of scan rate. | |
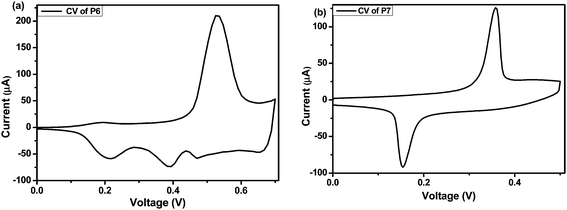 |
| Fig. 7 CV of (a) P6 and (b) P7 using ACN/TBAPC solvent/electrolyte system. | |
Electrochemical polymerization of compound 6 (Fig. 6b) and 7 (Fig. 6d) along with the unsubstituted analogous 4 and 5 (Fig. S5†) were carried out in dry acetonitrile containing 0.1 M TBAPC as supporting electrolyte at the Pt-disk working electrode at a scan rate of 50 mV s−1 (Scheme 2). The current density was increased gradually upon repetitive scanning indicating the deposition of polymer layer on platinum disk electrode. Scan rate dependence of these polymer films were also studied in the range of 50–200 mV s−1. Both the polymers exhibited linear scaling of the cathodic and anodic current with increase in scan rate (inset of Fig. 6b and d) and (inset of Fig. S5b & d†) suggesting in the formation of electroactive and non-diffusive polymer film.
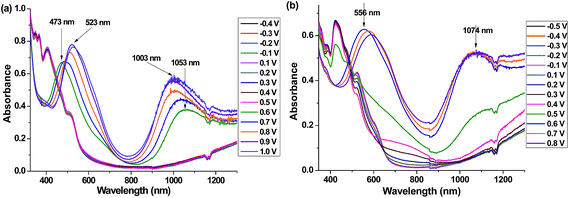 |
| Fig. 8 Spectroelectrochemistry of (a) P6 (between −0.4 and 1.1 V) and (b) P7 (between −0.5 and 1.0 V) in a comonomer free 0.1 M TBAPC/ACN solution. | |
Electrochemistry of these polymers were carried out in dry acetonitrile solvent using TBAPC as the supporting electrolyte with platinum disk electrode precoated with polymer film in a monomer free solution (Fig. 7). The oxidation onset potential for P4, P5, P6 and P7 were found to be 0.22, 0.08, 0.45 and 0.31 V, that correspond to the HOMO levels of −4.66, −4.52, −4.89 and −4.75 eV, respectively. Similar to the trend observed for monomers, the oxidation onset potentials of P4 and P5 are lower than that of P6 and P7, respectively. The HOMO level was found to be destabilized more in the case of P7 compared to that of P6 as expected from the better electron donating ability of selenophene than that of thiophene. The calculations of the LUMO levels were carried out relative to the HOMO and absorption onsets. The LUMO levels for P6 and P7 were found to be −2.76 and −2.90 eV respectively. The low lying LUMO of P7 can be attributed to the presence of more polarizable selenium atom.
Spectroelectrochemistry
In the absorption spectra of P6 in thin film showed absorption maxima at 349, 366, 405 and 509 nm whereas, that of P7 at 357, 383, 423, 522 nm (Fig. 8). The polymer films were studied with spectroelectrochemistry to probe their optical changes and electrochemical stability upon anodic doping as these displayed distinct oxidation peaks in CV but negligible reduction peaks.33 Thin films of P6 and P7 were deposited on indium tin oxide (ITO) coated glass slides using constant potentials of 0.85 and 0.7 V, respectively to attain a charge of 50 mC. The spectroelectrochemical changes were investigated using a UV-vis-NIR spectrophotometer in a comonomer free, 0.1 M TBAPC/ACN solvent/electrolyte system with increasing the applied potential from −0.4 to 1.0 V and −0.5 to 0.8 V for P6 and P7, respectively (Fig. 8). Upon increase in potential, a sharp decrease in the intensity of the UV-vis absorption bands (arises due to the π–π* transition in the neutral polymer) for both the polymers were observed. Oxidation of the polymers resulted in the color change of yellow to brown and orange to purple for P6 and P7 films, respectively. At 0.6 V two new UV-vis bands originated (in consumption of the neutral state absorption peaks) at 473 and 1053 nm in the absorption spectrum of P6 due to the formation of singly charged species (polaron) and doubly charged species (bipolaron), respectively (Fig. 8a). In between the potential range of 0.6–1.0 V the peak positions of the polaronic and bipolaronic species shifted to higher and lower wavelengths by 50 nm, respectively. This could be attributed to the discrete structural changes during the formation of the polaron and bipolaron which in turn affect the newly formed intermediate energy levels. The polaronic (556 nm) and bipolaronic species (1074 nm) of P7 were found at lower potential (Fig. 8b) than that was observed for P6. Though the peak position for the polaron shifted upon application of higher potential, the bipolaronic peak remained unaltered. This may be attributed to the better charge carrying ability34–36 of the selenium atom compared to sulfur, which could provide improved stability to the doped polymer chain of P7 compared to P6. This is the first report of any spectroelectrochemistry experiment on polymeric system of multifused chalcogenopyrrole.
Generally sudden changes in optical properties of the conjugated polymers under small potential difference is rare.37 In the present work the formation of a polaron and a bipolaron of the polymers was observed within a bias difference of 0.1 V (between 0.5 and 0.6 V, 0.4 and 0.5 V for P6 and P7, respectively), which could be attributed to fully oxidized polymer chains. This may be caused by two major reasons. Firstly, the presence of methylated pyrrole rings in the comonomer increases the electron rich nature of the fused polymer chains similar to the case of methyl substituted polyanilines.38 Secondly, the significant π-stacking between the multifused molecules of 6 and 7 in solid, which if present in polymer could lead to closely-stacked polymer chains that in turn facilititates the phonon hoping process.
Theoretical calculations
DFT calculations using Gaussian 09 program39 and applying periodic boundary condition (PBC)40 with B3LYP functional and the 6-31G(d) basis set41 were performed on 6, 7, P6, and P7 (hexyl chains were replaced by methyl groups) to gain insight into the frontier energy levels and to correlate them with the experimental findings. Any kind of geometrical twist was not observed in the polymer backbone of P6 and P7 (side view on Table 3), that results in uninterrupted π-conjugation along polymer chain. DFT calculations showed that the band gaps obtained for 6 and 7 are 3.71 and 3.68 eV, respectively, that are in good agreement with experimentally obtained values (Table 1). For the polymers P6 and P7 theoretical calculations revealed that the band gap decreases on going from P6 to P7 as a result of the presence of selenophenopyrrole moiety in the latter case that increases the effective conjugation due to the presence of selenophene ring (Table 2).42
Table 2 Electrochemical properties of polymers P6 and P7
Polymers |
EHOMOa (eV) |
ELUMOb (eV) |
Band gap (eV) |
Exp.c |
Calcd.d |
The HOMO energy levels were obtained from the equation EHOMO = − (4.8 − E1/2ferrocene + Eoxonset) eV. LUMO energy levels were calculated from the equation ELUMO = EHOMO + Eoptg. Calculated from Eoptg = hc/λonset. DFT calculated band gaps. |
P4 |
−4.66 |
— |
— |
— |
P5 |
−4.52 |
— |
— |
— |
P6 |
−4.89 |
−2.76 |
2.13 |
2.13 |
P7 |
−4.75 |
−2.90 |
1.85 |
2.05 |
Table 3 Optimized structures of P6 and P7
Polymer |
Top view |
Side view |
HOCO (eV) |
LUCO (eV) |
P6 |
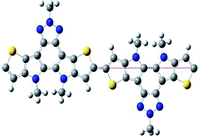 |
 |
−4.04 |
−1.91 |
P7 |
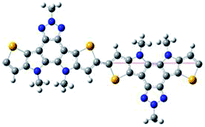 |
 |
−4.02 |
−1.96 |
Conclusion
In summary we have synthesized two new π-extended triazoles fused with thienopyrrole and selenophenopyrrole moieties using the Stille coupling reaction of 1 with the corresponding aryltin derivative followed by the PPh3 mediated reductive cyclization under microwave condition that decreases the reaction time dramatically. SCXRD analysis of compound 4 and 5 revealed the formation of unique supramolecular network via hydrogen bonding with the solvent ethyl acetate molecule. The SCXRD study indicates nearly planar structures of compound 6 and 7 with dense crystal packing. From the emission spectra strong support was observed for more polar excited state. The electrochemical properties revealed that in compound 7 the HOMO–LUMO energy gap decreases due to the presence of more electron rich selenophene unit. Spectroelectrochemical characterization showed the formation of a polaron and a bipolaron of the polymers P6 and P7 within a bias difference of 0.1 V. To the best of our knowledge it is the first report of electrochemical polymerization of π-extended triazoles fused with thienopyrrole and selenophenopyrrole moieties.
Experimental details
General measurement and characterization
All reactions were performed under nitrogen atmosphere to maintain the dry condition. Dry toluene and THF were distilled from sodium/benzophenone prior to use. Thiophene, selenophene, tributyltinchloride (Bu3SnCl), Pd(PPh3)4, triphenylphosphine (PPh3) and tetrabutylammonium perchlorate (TBAPC) were purchased from Aldrich and used without further purification. o-Dichlorobenzene was purchased from spectrochem and used without further purification. n-BuLi (1.6 M in hexane) was purchased from Neo Synth. 1H NMR and 13C NMR spectra of the compounds were taken on Jeol ECS 400 MHz spectrometer with CDCl3 or DMSO-d6 as the solvent, and chemical shifts (δ) are reported in parts per million (ppm) relative to tetramethylsilane as the internal standard values. The coupling constants (J) are given in Hz. Microwave synthesis was done using microwave synthesis reactor – Anton Paar Monowave 300, and the glass vial capped with silicone septum. Electrochemical studies were carried out with a Princeton Applied Research 263A potentiostat using platinum (Pt) disk electrode as the working electrode, a platinum wire as counter electrode and an AgCl-coated Ag wire as the reference electrode. Nonaqueous Ag/AgCl wire was prepared by dipping silver wire in a solution of FeCl3 and HCl. Pt disk electrodes were polished with alumina and washed with water, and acetone and were dried with nitrogen gas before use to remove any incipient oxygen. Electropolymerization was done on ITO-coated glass as a working electrode. The polymer films were deposited on ITO-coated glass electrode with dimensions of 5 × 0.7 cm2 at monomer's first oxidation potential (V) versus Ag/AgCl and a passing charge of 50–100 mC in 0.1 M TBAPC/ACN. Before examining the optical properties of polymer films, the films were rinsed with ACN. UV-vis-near infrared (NIR) spectra were recorded on a HITACHI U-4100 UV-vis-NIR spectrophotometer. In spectrochemical measurements, the working electrode was an ITO coated glass slide, the counter electrode was a platinum wire, and nonaqueous Ag/AgCl was used as the reference electrode. All electrochemical potentials were reported against Ag/AgCl taking ferrocene as external standard, the E1/2ferrocene is +0.37 V.
Synthesis
The synthesis of compound 1 and 2 are followed from the procedures.17
Synthesis of compound 3. In a 100 mL three neck round bottomed flask under nitrogen atmosphere was added compound 1 (1 g, 2.216 mmol), 2-tributylstannyl selenophene (2.32 g, 5.54 mmol) in dry toluene as solvent. The reaction mixture was purged with nitrogen for 15 min, then Pd(PPh3)4 (0.05 mmol) was added and the reaction mixture was refluxed overnight. Then the solvent was evaporated and the desired compound was isolated by silica gel column chromatography with hexane/dichloromethane (4/1) to yield 3 as orange solid (800 mg, 66%). Mp. 135 °C, 1H NMR (400 MHz, CDCl3, δ/ppm): 8.39 (m, 2H), 7.68 (m, 2H), 7.42 (m, 2H), 4.80 (t, J = 7.2 Hz, 2H), 2.13 (m, 2H), 1.36–1.33 (m, 6H), 0.87 (t, J = 7 Hz, 2H). 13C NMR (100 MHz, CDCl3, δ/ppm): 141.5, 139.1, 136.9, 134.2, 132.5, 130.2, 121.0, 57.9, 30.8, 29.8, 26.1, 22.3, 14.0. HRMS (M + H)+: calculated for C20H20N5O4Se2+ 553.9840, found 553.9830.
Synthesis of compound 4. In a microwave tube o-dichlorobenzene (2 mL), compound 2 (350 mg, 0.76 mmol) and PPh3 (2 g, 7.64 mmol) were added and heated at 180 °C for 15 min in the microwave reactor. Then the solvent was evaporated under reduced pressure and the reaction mixture was subjected to column chromatography (silica gel, 60–120 mesh) using hexane/ethyl acetate (1/1) to yield 4 as colourless solid (200 mg, 67%). Mp. 70 °C, 1H NMR (400 MHz, CDCl3, δ/ppm): 11.58 (br s, 2H), 7.54 (d, J = 4 Hz, 2H), 7.39 (d, J = 4 Hz, 2H), 4.76 (t, J = 5.8 Hz, 2H), 2.05 (m, 2H), 1.33 (m, 6H), 0.84 (t, J = 5.8 Hz, 2H). 13C NMR (100 MHz, CDCl3, δ/ppm): 140.7, 134.5, 127.6, 125.6, 117.1, 112.7, 104.0, 55.0, 30.6, 29.7, 25.6, 21.9, 13.7. HRMS (M + H)+: calculated for C20H20N5S2+ 394.1155, found 394.1201.
Synthesis of compound 5. In a microwave tube o-dichlorobenzene (2 mL), compound 3 (500 mg, 0.906 mmol) and PPh3 (2.36 g, 9.06 mmol) were added and heated in the microwave reactor at 180 °C for 15 min. Then the solvent was evaporated under reduced pressure and the reaction mixture was subjected to silica gel column chromatographic separation using hexane/ethyl acetate (3/2) to yield 5 as colourless solid (300 mg, 68%). Mp. 100 °C, 1H NMR (400 MHz, CDCl3, δ/ppm): 11.64 (br s, 2H), 8.03 (d, J = 5.3 Hz, 2H), 7.65 (d, J = 5.3 Hz, 2H), 4.75 (t, J = 7.2 Hz, 2H), 2.03 (m, 2H), 1.33–1.25 (m, 6H), 0.85 (t, J = 7.2 Hz, 2H). 13C NMR (100 MHz, CDCl3, δ/ppm): 141.5, 134.9, 129.0, 126.8, 116.0, 115.5, 107.2, 55.0, 30.6, 29.8, 25.7, 21.9, 13.8. HRMS (M + H)+: calculated for C20H20N5Se2+ 490.0044, found 490.0370.
Synthesis of compound 6. A mixture of compound 4 (100 mg, 0.25 mmol), iodomethane (0.1 mL, 1.65 mmol), and sodium hydroxide (100 mg, 2.5 mmol) in DMF was stirred at rt for 6 h. Then the reaction mixture was poured into water and the resulting suspension was extracted with ethyl acetate. The ethyl acetate layer was collected and dried over Na2SO4. The solvent was removed by rotary evaporation, and the residue was purified by silica gel column chromatography with hexane/ethyl acetate (4/1) to yield 6 as white solid (70 mg, 66%). Mp. 160 °C, 1H NMR (400 MHz, CDCl3, δ/ppm): 7.40 (d, J = 5.3 Hz, 2H), 7.14 (d, J = 5.3 Hz, 2H), 4.80 (t, J = 7.2 Hz, 2H), 4.22 (s, 6H), 2.17 (m, 2H), 1.40–1.35 (m, 6H), 0.88 (t, J = 6.8 Hz, 2H). 13C NMR (100 MHz, CDCl3, δ/ppm): 146.1, 135.5, 130.8, 126.1, 118.5, 110.8, 107.9, 55.9, 38.0, 31.3, 30.3, 26.3, 22.4, 13.9. HRMS (M + H)+: calculated for C22H24N5S2+ 422.1473, found 422.1474.
Synthesis of compound 7. A mixture of compound 5 (100 mg, 0.205 mmol), iodomethane (0.09 mL, 1.33 mmol), and sodium hydroxide (82 mg, 2.05 mmol) in DMF was stirred at rt for 8 h. Then the reaction mixture was poured into water and the resulting suspension was extracted with ethyl acetate. The ethyl acetate layer was collected and dried over Na2SO4. The solvent was removed by rotary evaporation, and the residue was purified by silica gel column chromatography with hexane/ethyl acetate (3/1) to yield 7 as white solid (80 mg, 75%). Mp. 190 °C, 1H NMR (400 MHz, CDCl3, δ/ppm): 7.38 (d, J = 5.3 Hz, 2H), 7.12 (d, J = 5.3 Hz, 2H), 4.80 (t, J = 7.2 Hz, 2H), 4.18 (s, 6H), 2.17 (m, 2H), 1.40–1.34 (m, 6H), 0.88 (t, J = 7.2 Hz, 2H). 13C NMR (100 MHz, CDCl3, δ/ppm): 146.1, 135.5, 130.8, 126.1, 118.5, 110.8, 107.9, 55.9, 38.0, 31.3, 30.3, 26.3, 22.4, 13.9. HRMS (M + H)+: calculated for C22H24N5Se2+ 518.0357, found 518.0370.
Acknowledgements
We thanks CSIR, India for funding. SG and AB thanks CSIR for research fellowship.
References
- Handbook of Thiophene-Based Materials: Applications in Organic Electronics and Photonics, ed. I. F. Perepichka and D. F. Perepichka, John Wiley & Sons, New York, 2009, vol. 1 and 2 Search PubMed.
- J. H. Burroughes, D. D. C. Bradley, A. R. Brown, R. N. Marks, K. Mackay, R. H. Friend, P. L. Burn and A. B. Holmes, Nature, 1990, 347, 539 CrossRef CAS.
- A. K. Asatkar, A. Bedi and S. S. Zade, Isr. J. Chem., 2014, 54, 467 CrossRef CAS.
- I. F. Perepichka, D. F. Perepichka, H. Meng and F. Wudl, Adv. Mater., 2005, 17, 2281 CrossRef CAS.
- H. Sirringhaus, P. Brown, R. H. Friend, M. Nielsen, K. Bechgaard, B. Langeveld-Voss, A. Spiering, R. Janssen, E. Meijer, P. Herwig and D. de Leeuw, Nature, 1999, 401, 685 CrossRef CAS PubMed.
- H. Sirringhaus, Adv. Mater., 2014, 26, 1319 CrossRef CAS PubMed.
- J. Mei, Y. Diao, A. L. Appleton, L. Fang and Z. Bao, J. Am. Chem. Soc., 2013, 135, 6724 CrossRef CAS PubMed.
- T.-Y. Chu, J. Lu, S. Beaupre, Y. Zhang, J.-R. Pouliot, S. Wakim, J. Zhou, M. Leclerc, Z. Li, J. Ding and Y. Tao, J. Am. Chem. Soc., 2011, 133, 4250 CrossRef CAS PubMed.
- L. Dou, J. You, Z. Hong, Z. Xu, G. Li, R. A. Street and Y. Yang, Adv. Mater., 2013, 25, 6642 CrossRef CAS PubMed.
- R. S. Kularatne, H. D. Magurudeniya, P. Sista, M. C. Biewer and M. C. Stefan, J. Polym. Sci., Part A: Polym. Chem., 2013, 51, 743 CrossRef CAS.
- J. E. Anthony, Angew. Chem., Int. Ed., 2008, 47, 452 CrossRef CAS PubMed.
- S. S. Zade and M. Bendikov, Angew. Chem., Int. Ed., 2010, 49, 4012 CrossRef CAS PubMed.
- H. S. Chandak and S. S. Zade, Org. Electron., 2014, 15, 2184 CrossRef CAS PubMed.
- A. Fukazawa and S. Yamaguchi, Chem.–Asian J., 2009, 4, 1386 CrossRef CAS PubMed.
- G. L. Gibson, T. M. McCormick and D. S. Seferos, J. Am. Chem. Soc., 2012, 134, 539 CrossRef CAS PubMed.
- S. Das, P. B. Pati and S. S. Zade, Macromolecules, 2012, 45, 5410 CrossRef CAS.
- L. Biniek, I. Bulut, P. Lévêque, T. Heiser and N. Leclerc, Tetrahedron Lett., 2011, 52, 1811 CrossRef CAS PubMed.
- Y.-J. Cheng, C.-H. Chen, Y.-J. Ho, S.-W. Chang, H. A. Witek and C.-S. Hsu, Org. Lett., 2011, 13, 5484 CrossRef CAS PubMed.
- S.-I. Kato, T. Furuya, A. Kobayashi, M. Nitani, Y. Ie, Y. Aso, T. Yoshihara, S. Tobita and Y. Nakamura, J. Org. Chem., 2012, 77, 7595 CrossRef CAS PubMed.
- G. Balaji, W. L. Shim, M. Parameswaran and S. Valiyaveettil, Org. Lett., 2009, 11, 4450 CrossRef CAS PubMed.
- N. Akbaşoğlu, A. Balan, D. Baran, A. Cirpan and L. Toppare, J. Polym. Sci., Part A: Polym. Chem., 2010, 48, 5603 CrossRef.
- S. C. Price, A. C. Stuart, L. Yang, H. Zhou and W. You, J. Am. Chem. Soc., 2011, 133, 4625 CrossRef CAS PubMed.
- L. Zhang, C. He, J. Chen, P. Yuan, L. Huang, C. Zhang, W. Cai, Z. Liu and Y. Cao, Macromolecules, 2010, 43, 9771 CrossRef CAS.
- W. W. H. Wong, J. Subbiah, S. R. Puniredd, W. Pisula, D. J. Jones and A. B. Holmes, Polym. Chem., 2014, 5, 1258 RSC.
- J. L. Banal, J. Subbiah, H. Graham, J.-K. Lee, K. P. Ghigginoa and W. W. H. Wong, Polym. Chem., 2013, 4, 1077 RSC.
- J.-K. Lee, M. C. Gwinner, R. Berger, C. Newby, R. Zentel, R. H. Friend, H. Sirringhaus and C. K. Ober, J. Am. Chem. Soc., 2011, 133, 9949 CrossRef CAS PubMed.
- D. Baran, A. Balan, S. Celebi, B. M. Esteban, H. Neugebauer, N. S. Sariciftci and L. Toppare, Chem. Mater., 2010, 22, 2978 CrossRef CAS.
- T. L. Tam, H. Li, Y. M. Lam, S. G. Mhaisalkar and A. C. Grimsdale, Org. Lett., 2011, 13, 4612 CrossRef CAS PubMed.
- M. Manuela, M. Raposo, A. Maurício, C. Fonseca and G. Kirsch, Tetrahedron, 2004, 60, 4071 CrossRef PubMed.
- Y. A. Udum, S. Tarkuc and L. Toppare, Synth. Met., 2009, 159, 361 CrossRef CAS PubMed.
- J. I. G. Cadogan, Organophosphorous Reagents in Organic Synthesis, Academic Press, London, 1979, ch. 6 Search PubMed.
- A. W. Freeman, M. Urvoy and M. E. Criswell, J. Org. Chem., 2005, 70, 5014 CrossRef CAS PubMed.
- Q. Sun, H. Wang, C. Yang and Y. Li, J. Mater. Chem., 2003, 13, 800 RSC.
- A. Patra and M. Bendikov, J. Mater. Chem., 2010, 20, 422 RSC.
- A. Bedi, S. P. Senanayak, K. S. Narayan and S. S. Zade, Macromolecules, 2013, 46, 5943 CrossRef CAS.
- A. Bedi and S. S. Zade, Macromolecules, 2013, 46, 8864 CrossRef CAS.
- M. Leclerc, J. Guay and L. H. Dao, Macromolecules, 1989, 22, 649 CrossRef CAS.
- M. Leclerc, J. Guay and L. H. Dao, J. Electrochem. Soc., 1988, 21, 251 Search PubMed.
- M. J. Frisch, G. W. Trucks, H. B. Schlegel, G. E. Scuseria, M. A. Robb, J. R. Cheeseman, G. Scalmani, V. Barone, B. Mennucci, G. A. Petersson, H. Nakatsuji, M. Caricato, X. Li, H. P. Hratchian, A. F. Izmaylov, J. Bloino, G. Zheng, J. L. Sonnenberg, M. Hada, M. Ehara, K. Toyota, R. Fukuda, J. Hasegawa, M. Ishida, T. Nakajima, Y. Honda, O. Kitao, H. Nakai, T. Vreven, J. A. Montgomery Jr, J. E. Peralta, F. Ogliaro, M. Bearpark, J. J. Heyd, E. Brothers, K. N. Kudin, V. N. Staroverov, R. Kobayashi, J. Normand, K. Raghavachari, A. Rendell, J. C. Burant, S. S. Iyengar, J. Tomasi, M. Cossi, N. Rega, J. M. Millam, M. Klene, J. E. Knox, J. B. Cross, V. Bakken, C. Adamo, J. Jaramillo, R. Gomperts, R. E. Stratmann, O. Yazyev, A. J. Austin, R. Cammi, C. Pomelli, J. W. Ochterski, R. L. Martin, K. Morokuma, V. G. Zakrzewski, G. A. Voth, P. Salvador, J. J. Dannenberg, S. Dapprich, A. D. Daniels, O. Farkas, J. B. Foresman, J. V. Ortiz, J. Cioslowski and D. J. Fox, Gaussian 09, Revision E.01, Gaussian, Inc., Wallingford, CT, 2009 Search PubMed.
- S. S. Zade and M. Bendikov, Org. Lett., 2006, 8, 5243 CrossRef CAS PubMed.
- W. Koch and M. C. Holthausen, A Chemist's Guide to Density Functional Theory, Wiley-VCH, New York, 2000 Search PubMed.
- S. S. Zade and M. Bendikov, Chem.–Eur. J., 2009, 15, 8613 CrossRef CAS PubMed.
Footnote |
† Electronic supplementary information (ESI) available: 1H and 13C NMR spectra of all new compounds and optimized structures, absolute energies, absorption spectra, picture of HOMO and LUMO, and coordinates for optimized structure of 6, 7, P6 and P7, CV of polymers. CCDC 999722 and 1021386–1021388. For ESI and crystallographic data in CIF or other electronic format see DOI: 10.1039/c4ra14850a |
|
This journal is © The Royal Society of Chemistry 2015 |