DOI:
10.1039/C4RA14802A
(Paper)
RSC Adv., 2015,
5, 6323-6331
Cubic Ag2O nanoparticle incorporated mesoporous silica with large bottle-neck like mesopores for the aerobic oxidative synthesis of disulfide†
Received
18th November 2014
, Accepted 15th December 2014
First published on 16th December 2014
Abstract
Highly stable, environmentally benign cubic Ag2O nanoparticles dispersed on mesoporous silica with large bottle-neck like mesopores were synthesized and characterized by BET surface area analysis, HR TEM, EDX, FTIR and powder XRD studies. The Ag2O nanoparticles were homogeneously distributed having an average size of 20–40 nm. The activity of the catalyst was probed through an efficient aerobic chemoselective oxidation of thiols to disulfides in water under atmospheric oxygen as the cheapest oxidant. Alkyl, aryl and imine containing symmetrical disulfides can be easily obtained in high yields under mild reaction conditions with no over oxidized product. The efficiency of the catalyst was further demonstrated as the highly sensitive imine bond was well sustained under these mild reaction conditions. Moreover, unlike the other literature precedents, in this present work, single crystal structures of both simple symmetrical disulphide as well as imine containing disulphides are reported for the first time. The catalyst is very much water compatible and can be recycled and reused for at least five cycles. The standard leaching experiment proved that the reaction was heterogeneous with this recyclable catalyst.
Introduction
Disulfides are readily formed through covalent cross-linking and are one of the most important organic sulfur compounds possessing an exclusive chemistry both in biological1 (DNA cleavage properties, stabilization of peptides in proteins, and drug delivery systems) and chemical2 (protecting groups, vulcanizing agents, and oils for rubber and elastomers) processes. Disulfides are used as key intermediates for the synthesis of sulfinyl, sulfenyl compounds3 and sulphur containing heterocycles.4 As disulfides are relatively more stable to organic reactions such as oxidation, alkylation and acylation compared to the corresponding free thiols, the thiol groups can conveniently be protected as a disulfide during oxidation of certain other functional groups. The desired thiol can be generated from the disulfide either by reduction or by other sulfur–sulfur bond cleavage reactions such as CN–, OH– or hydrazines.5 Oxidation of thiols to the corresponding disulfides is a characteristic functional group transformation. This disulfide may possibly be further oxidized to give disulfide S-oxides (thiolsulfinates), disulfide S-dioxides (thiolsulfonates), and sulfonic acids.6 Consequently, considerable research has gone into controlling the initial oxidation to avoid over oxidations. Moreover, most of the thiols are toxic, have unpleasant odour and act as catalyst poisons. Therefore, from biological and practical points of view sweetening of catalyst poisons thiols2e to low volatile disulfides led us to investigate the introduction and applications of new member of this category of reagents in oxidation of thiols to the corresponding disulfides under neutral and mild conditions.
Reagents such as of MnO2,7 dichromates,8 borohydride exchange resin,9 DMSO/alumina,10 CBr4/K2CO3/18-crown-6/benzene,11 NaIO3/alumina,12a molybdate sulfuric acid/NaNO2,12b tetramethylammonium fluorochromate,13 cerium(IV) salt,14 copper salt,15 rhenium–sulfoxide complex,16a rhodium(I) complex,16b have been utilized for oxidation of thiols to disulfides. However, some of the reported reagents suffer from disadvantages such as toxicity, high price of some reagents, instability, hygroscopicity, low selectivity, long reaction time, difficulty of preparation, need for a large excess of the reagent and the over-oxidation of the disulfide to thiosulfinates and derivatives. Thus a milder, more selective and inexpensive reagent is still in demand. Solutions to these problems lead to the development of a good number of other oxidants, such as pyridinium chlorochromate,17 tributylammonium halochromates/silica gel,18a pyridinium fluorochromate,18b quinolinium dichromate,19a N-phenyltriazolinedione19b etc. The Burgess reagent20 has also been used for obtaining disulfides from thiols, with the high cost of this reagent being the main limitation of the method. However, with the increasing environmental concern, chemists have developed different catalysts with molecular oxygen as the primary oxidant which include diaryl tellurides under photosensitized conditions,21 Co(II)-Schiff base (Co-salophen),22a Mn(III)-Schiff base,22b vanadium polyoxometalate,23 20 mol% NaI/10 mol% FeCl3 (ref. 24) and 15 mol% Ni-nanoparticles.25 The oxidative dimerization of thiols with O2 was also possible in several ionic liquids,26a–c using laccases as catalyst,26d under sonication with Et3N in DMF.26e Using neutral Al2O3 under ball milling condition27a or using subcritical water under high pressure at 100 °C without catalyst.27b Despite some progress, most of these methods suffer from a number of disadvantages, including the use of toxic and/or expensive oxidants or catalysts. Some of the reagents employed are commercially unavailable and their preparation is tedious. Also, some catalysts suffer from low catalytic efficiency. In addition, many of the methods reported require the use of volatile organic solvents. Thus, there is still an interest in developing clean, fast, inexpensive, environmentally harmless oxidative methods that would produce the desirable disulfides in high yields.
No doubt that, oxidation by molecular oxygen (O2) in air as stoichiometric oxidant offers one of the most environmentally benign and ideal oxidation processes in organic synthesis.28 However for the oxidation with molecular oxygen the use of a catalyst becomes inevitable. The traditional catalysts for catalytic oxidation can be classified into the following categories: (a) supported precious metals29 (b) metal oxides and supported non-precious metals30 and (c) mixtures of metal oxides and precious metals.31 Generally speaking, metal oxides and non-precious metals exhibited somewhat low efficiency and shot life span in spite of their availability. Among supported precious metals, silver has been drawing more and more attentions due to its availability and high activity for some oxidation reaction at low temperatures.32 However, most of metal oxides supports are mainly structurally nonuniform, the size and the shape of the obtained metal nanoparticles are hard to be controlled. The catalytic performance of the catalysts is directly related to the particle-size and the reaction tends to occur easily on smaller particles by virtue of the increased surface area.33 If the metal oxide nanoparticles can be confined within a nanoporous host material, this may restrict the size to which the metal oxide nanoparticles can grow.32a,b Mesoporous silica34 has been found to be the promising templates to control the shape and size of the occluded metal/metal oxide nanoparticles.32c–e Actually, it's great high surface area is in favor for a high dispersion of the active component on the surface of the supports as well as increased availability of active sites, which should show a favorable impact on catalytic activity.35
In this work, we present our efforts obtained in the mild transformation of thiols to disulfides in aqueous media using Ag incorporated high surface area mesoporous silica catalyst (MSAGN) with bottle-neck like large mesopore and the cheapest oxidant on earth (atmospheric oxygen). It can be used for preparing aromatic, heteroaromatic and aliphatic symmetrical disulfides. It is pertinent to mention that thiols containing highly sensitive imine bonds are well tolerated under this mild reaction condition. For this present purpose we have synthesized several silver incorporated silica catalysts with different weight percentages of silver and denoted as MSAGN-x (x = wt% of silver). Among them MSAGN-10 performs most efficiently as an oxidation catalyst for this present synthesis.
Results and discussions
Characterization of the catalysts
N2 adsorption analysis. N2 adsorption–desorption isotherm of the silver incorporated mesoporous silica catalyst is shown in Fig. 1. The isotherm is a typical type IV isotherm with desorption hysteresis loop, which could be attributed to the mesoporous nature of the sample.36 The presence of hysteresis loop is due the presence of bottle-neck like large mesopore in the material. The BET surface area calculated from this isotherm is 579.05 m2 g−1 which is reasonably high considering the loading of heavy metal Ag in the sample surface. The pore volume estimated for this sample is 0.4273 cc g−1. Corresponding pore size distribution of the sample estimated by the non-local density functional theory (NLDFT) model is shown inset of Fig. 1. Estimated pore dimensions for the sample MSAGN-10 are found to be 2.9 nm and 6.95 nm. Peak pore dimension of 2.9 nm could be assigned due to the use of template CTAB in the sample preparation, whereas peak at 6.95 is assigned due to the presence of interparticle voids. Under the present synthesis conditions interparticle pores are generated, as confirmed from the hysteresis in the N2 sorption isotherm and TEM data. Size of these interparticle pores are bigger than 4 nm and this helps in the formation of Ag2O nanoparticles at the interparticle voids.
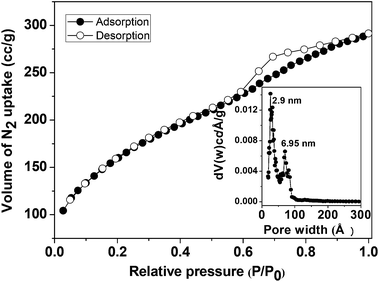 |
| Fig. 1 N2 adsorption–desorption isotherm of MSAGN-10 at 77 K. NLDFT pore size distribution is shown in the inset. | |
It is pertinent to mention that ink-bottle or large bottle neck like mesoporous silica with H2 type hysteresis loop has paramount importance as catalyst in organic synthesis, since the large transition states are easily accommodated inside the large pores and also the pore mouth is large enough to allow easy diffusion of organic molecules.
TEM analysis. TEM images of MSAGN-10 are shown in Fig. 2. A closer analysis of this electron microscopic data reveals that there is 3–10 nm pores in silica matrix. Some of the representative pores are shown in the TEM images (red dotted circled) (Panel a). This value matches well with the value obtained from N2 sorption analysis. In the TEM image (Panel b) we have circled one of the representative Ag2O nanoparticles which are having average size 20–40 nm and they are present in the matrix homogeneously.37
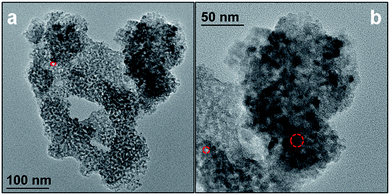 |
| Fig. 2 TEM image of MSAGN-10. | |
EDS elemental analysis. The chemical characterization for the material was carried out by EDX analysis. EDX analysis revealed that three elements –O, Si and Ag, existed in the material (Fig. 3). EDX chemical analysis confirmed that the expected silver incorporated mesoporous silica has successfully developed in this study. From the EDX elemental analysis a loading of silver of 0.886 mmol g−1 on silica surface was determined.
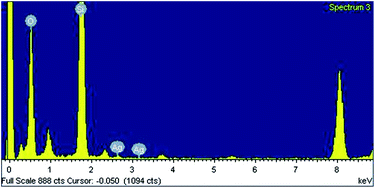 |
| Fig. 3 EDS spectrum of MSAGN-10. | |
Wide angle powder XRD pattern. Wide angle powder XRD pattern revealed the presence of cubic Ag2O nanoparticles in the mesoporous silica matrix. Crystalline planes corresponding to this cubic phase have been indexed in Fig. 4. This indexing agrees very well with the JCPDS 12-0793 (ref. 38) corresponding to a cubic phase of Ag2O.
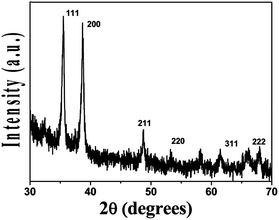 |
| Fig. 4 Wide angle powder XRD pattern of MSAGN-10. | |
Fourier transform infrared spectroscopy. FTIR spectra of Ag/SiO2 (MSAGN-10) in the region of 400–4000 cm−1 are shown in Fig. 5. In the IR spectra, water bands observed at around 3459 and 1634 cm−1 corresponding to stretching and bending vibrations indicate the hygroscopic nature of the powdered samples. Strong bands observed at 1095 and 969 cm−1 were assigned to the stretching vibration of Si–O–Si. The bands at 802 and 468 cm−1 were for the internal bonds of the tetrahedral SiO4 structural units.39
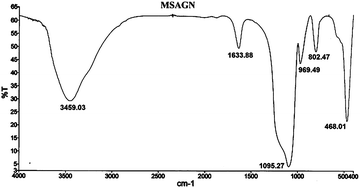 |
| Fig. 5 FTIR spectra of MSAGN-10 catalyst. | |
Recently, disulfide bond formation reactions have engrossed substantial research interest, because of the abundance of this disulfide functionality in biological relevant compounds. Herein, the disulfide bond formation reaction was studied with several catalysts under various reaction conditions. The oxidation of benzenethiol to diphenyl disulfide was chosen as model reaction to evaluate the catalytic activity of different catalysts (Table 1). Formation of disulfide bond was realized by FTIR analysis of the products. From the FTIR spectra in Fig. 6 it is clear that the peak at 2567.1 cm−1 corresponding to –S–H bond in the spectrum (a) has disappeared in the spectrum (b), and a new peak at 462.8 cm−1 appeared corresponding to the newly formed –S–S– bond in spectrum (b). The progress of this reaction can be visually monitored in naked eye as the reactant thiophenol is liquid and the product diphenyl disulfide is a white crystalline solid.
Table 1 Optimization of reaction conditiona

|
Entry |
Catalyst |
Solvents (4 mL) |
Yieldb (%) |
TON |
Reaction conditions: thiophenol (1 mmol), different catalysts (0.1 mmol for homogeneous catalysts and 20 mg for heterogeneous catalysts), different solvents, 5 h, reflux. Isolated yields. |
1 |
I2/O2 |
AcOH |
62 |
12.4 |
2 |
CuI/NBS |
Toluene |
69 |
13.8 |
3 |
CuI/O2 |
H2O |
77 |
15.4 |
4 |
CeO2/O2 |
H2O |
20 |
4.0 |
5 |
FeCl3/O2 |
Toluene |
49 |
9.8 |
6 |
Cu(OAc)2 |
Toluene |
62 |
12.4 |
7 |
CuBr/O2 |
Toluene |
61 |
12.2 |
8 |
Cu–SiO2/O2 |
H2O |
74 |
14.8 |
9 |
AgNO3/O2 |
Toluene |
55 |
11.0 |
10 |
Ag2O/O2 |
H2O |
64 |
12.8 |
11 |
SiO2/O2 |
H2O |
0 |
0 |
12 |
MSAGN-10/O2 |
H2O |
91 |
102.7 |
13 |
MSAGN-10/tBuOOH |
H2O |
85 |
95.9 |
14 |
MSAGN-10/N2 |
H2O |
0 |
0 |
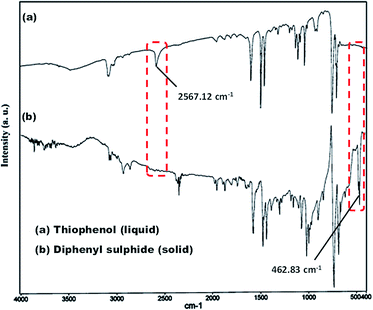 |
| Fig. 6 FTIR spectra of (a) thiophenol and (b) diphenyl disulfide. | |
Owing to very importance of these compounds and keeping in mind the possibility of the disulfide to undergo over oxidation to disulfide S-oxides, disulfide S-dioxides, and sulfonic acids, it becomes a tremendous task to control the reactions by appropriate choice of the reaction conditions as well as the catalysts. Initially different common oxidation catalysts were used with appropriate stoichiometric oxidants as shown in Table 1. Some of them showed good activity for oxidation of thiol in the initial stages of reaction. However with the advancement of reaction the selectivity for disulfide decreases due to the formation of side products like disulfide S-oxides, disulfide S-dioxides, and sulfonic acids. Therefore, at the end of the reaction, when no thiophenol were left, the yield of the disulfide was not satisfactory. Compared to the catalysts mentioned in Table 1, the present Ag/SiO2 (MSAGN-10) afforded better yield and very high selectivity for disulfide formation. Selectivity did not decrease even after the reaction was allowed to continue for 8 h (Fig. 7). Being heterogeneous and ease of separation of the catalyst from the reaction mixture, Ag/SiO2 (MSAGN-10) was chosen as a right choice of catalyst for the best yield of disulfide.
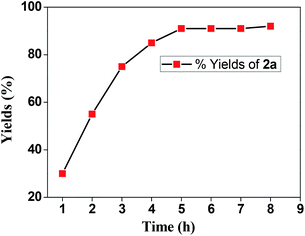 |
| Fig. 7 Wide selectivity to diphenyldithiol vs. time plots for the oxidation of thiophenol in the presence of molecular oxygen catalyzed by MSAGN-10. | |
In order to fully establish the role of Ag/SiO2 (MSAGN-10) in the present case, a control reaction was performed which showed that mesoporous silica, without any added metal, was not able to catalyze the disulfide formation reaction (entry 11). Interestingly, also in the absence of air (entry 14) the oxidation reaction could not proceed. Further, when bulk Ag2O was used as the catalyst under identical reaction conditions, only a very poor yield of diphenyldithiol was observed (entry 10). These results suggest that porosity and high surface area of silver incorporated mesoporous silica are crucial for this catalytic reaction.
The reaction was also studied with varied percentage of silver on silica and the results are represented in Table 2. From the Table 2 it is found that 10% by weight of Ag on silica exhibits best performance for the present synthesis.
Table 2 Effect of silver loadings on the yield of the disulfidea

|
Entry |
wt% of silver (x) |
Yieldb (%) |
TON |
Reaction conditions: thiophenol (1 mmol), 20 mg MSAGN-x (x = 2, 5, 10, 20 wt%), water, 5 h, reflux in air. Isolated yields. |
1 |
2 |
21 |
23.7 |
2 |
5 |
58 |
65.5 |
3 |
10 |
91 |
102.7 |
4 |
20 |
81 |
91.4 |
The reusability of the silver incorporated mesoporous silica catalyst (MSAGN-10) in the disulfide bond formation reaction was also examined by using thiophenol as a reference substrate in aqueous medium. The kinetics of the fresh catalyst was evaluated as shown in Fig. 8. The yield did not increase substantially after 8 h. Also we had performed the kinetic study with the recycled catalyst for the next consecutive four runs. The results of kinetic plots suggested that the catalyst retained almost same efficiency as that of fresh catalyst.
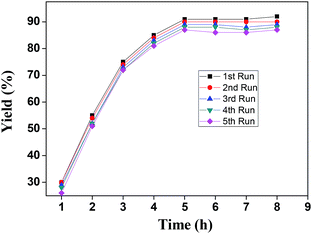 |
| Fig. 8 Kinetics plot demonstrating the recycling efficiency of the mesoporous MSAGN-10 catalyst. | |
With the optimized conditions in hand, to further extend the scope and generality of this protocol the applicability of various thiols was next assessed for this reaction. Both electron-withdrawing (Scheme 1, entries 2–5) and electron donating substituents (Scheme 1, entries 6–9), in the aromatic ring, gave the desired products with good to excellent yields. The presence of substituents at the ortho position did not exert any obvious effect on the yield of the reaction (Scheme 1, entries 5, 6 and 9). It was pleasing to find that sterically bulky naphthalene-2-thiol also reacted very efficiently with no side reactions (Scheme 1, entry 9). The reaction was also compatible with aliphatic thiols bearing alkyl chains (Scheme 1, entry 12), and alicyclic thiols (Scheme 1, entry 11). Differently substituted thiophenol compounds were studied here, and the MSAGN-10 catalyst exhibited good catalytic activity in all cases with no over oxidized product in aqueous medium.
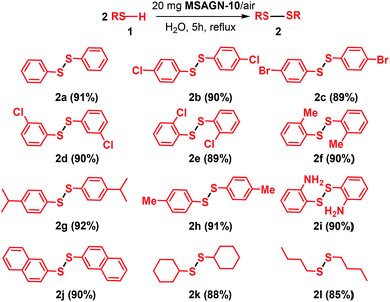 |
| Scheme 1 Substrate scope and synthesized disulphides. | |
It is pertinent to mention that highly sensitive imine bond is well sustained under this mild reaction condition. Therefore, when the compounds (3) were subjected to reflux in presence of MSAGN-10 catalyst, compound (4) was formed in excellent yield without the rapture of C
N bond (Scheme 2). This type of compounds have novel application as template for the growth metal oxide nano particle on their surface,40 as precursors of sulphur containing heterocycles like benzothiazoles41 and benzospirothiazoles41,42 etc.
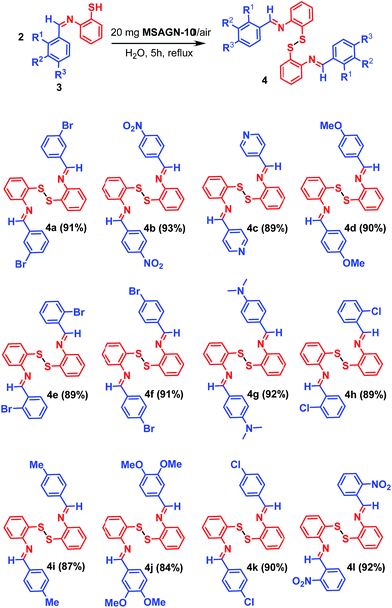 |
| Scheme 2 Substrate scope and synthesized imine containing disulphides. | |
At the outset of our work, to the best of our knowledge there was no literature precedent in which a single crystal structure of simple symmetrical disulphide was reported. In most of the earlier works the structure determination mainly relied on FTIR analysis. The conversion of –S–H to –S–S– could be realized from the IR spectrum as the peak at 2567 cm−1 corresponding to –S–H of thiophenol disappeared and reappeared at 463 cm−1 corresponding to –S–S– in the product, indicating the presence of a disulphide. Gratifyingly, we confirmed the structure of diphenyl disulphide compound (2a) unambiguously through an X-ray single crystal analysis (CCDC 1024098) (Fig. 9). Furthermore we also presented X-ray single crystal structure of compound (4f) (CCDC 1024099) to prove unambiguously that the imine bond was intact after formation of the –S–S– bond from the corresponding compound (Fig. 10). The crystal data and details concerning data collection and structural refinement are given in Table 3.
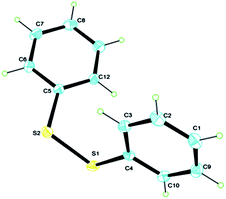 |
| Fig. 9 ORTEP representation of 2a (CCDC 1024098). | |
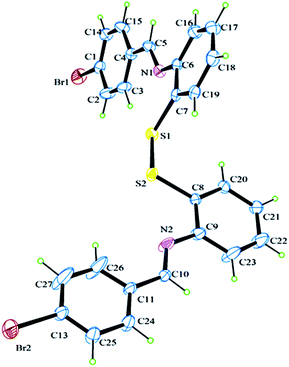 |
| Fig. 10 ORTEP representation of 4f (CCDC 1024099). | |
Table 3 Detailed analysis data of the X-ray crystal structure of the compound 2a and 4f
Crystal descriptions |
Compound 2a |
Compound 4f |
Formula |
C12H10S2 |
C26H18Br2N2S2 |
Formula weight |
218.32 |
582.34 |
Crystal system |
Orthorhombic |
Triclinic |
Space group |
P2(1)2(1)2(1) |
P![[1 with combining macron]](https://www.rsc.org/images/entities/char_0031_0304.gif) |
a [Å] |
5.6420(4) |
7.625(2) |
b [Å] |
8.1176(6) |
7.802(2) |
c [Å] |
23.6684(17) |
20.002(5) |
α [°] |
90 |
87.587(3) |
β [°] |
90 |
88.794(3) |
γ [°] |
90 |
83.332(3) |
V [Å3] |
1084.00(14) |
1180.7(5) |
Z |
4 |
2 |
D(calc) [g cm−3] |
1.338 |
1.638 |
Absorption coefficient μ [mm−1] |
0.446 |
3.627 |
Absorption correction |
Empirical |
Empirical |
F(000) |
456.0 |
580.0 |
Crystal size [mm] |
0.80 × 0.560 × 0.40 |
0.30 × 0.28 × 0.25 |
Temperature (K) |
296 |
296 |
Radiation [Å] |
MoKα (λ = 0.71073) |
MoKα (λ = 0.71073) |
Theta min–max [°] |
1.72, 26.65 |
1.02, 23.710 |
Collection range |
−6 ≤ h ≥ 7; −10 ≤ k ≥ 10; −26 ≤ l ≥ 27 |
−8 ≤ h ≥ 8; −8 ≤ k ≥ 8; −22 ≤ l ≥ 22 |
Reflections collected |
2191 |
3537 |
Independent reflections (Rint) |
1947 (0.0335) |
2323 (0.0336) |
R, wR2, S |
0.0313, 0.1022, 0.799 |
0.0467, 0.1583, 0.714 |
CCDC number |
1024098 |
1024099 |
A probable mechanistic pathway for this disulfide bond formation reaction is shown in Scheme 3. Here the reaction mechanism can be expressed as eqn (1)–(3).
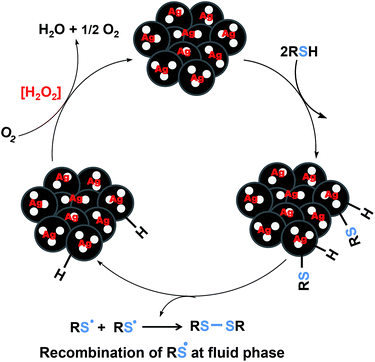 |
| Scheme 3 Probable mechanistic pathway for the mesoporous MSAGN-10 catalyzed aerobic oxidative synthesis of disulphides using molecular oxygen as oxidant. | |
Following the reaction as in eqn (1), the thiol RSH moiety adsorbed on the Ag2O nanoparticle on silica surface induces oxidative addition of the metal center to give Ag–SR and Ag–H species. Two RS˙ radicals then come out from the solid surface and combine with each other in the fluid phase, making possible the formation of the disulfide compound RS–SR.43 If the recombination would be in the solid phase then sterically bulky imine containing thiophenols (3) would not react efficiently to give even more bulky product (4) because of steric repulsion with the catalyst surface. The Ag–H intermediate will reduce molecular oxygen O2 and produce hydrogen peroxide, which is decomposed into water and O2 according to eqn (3) and closing up the catalytic cycle. The reaction performed in N2 atmosphere did not show any catalytic activity. This result demonstrates that the catalyst was only active in the presence of oxygen and again supports our possible catalytic cycle.
Catalyst stability and recycling
Hot-filtration test. To confirm the heterogeneous nature of catalyst and catalytic activity bound to the solid phase, a hot-filtration test was performed. The thiophenol (1 mmol) and mesoporous MSAGN-10 (20 mg) were mixed together in water (4 mL) on an oil bath under reflux. The catalyst was filtered off (from the hot reaction mixture) after 1 h. The filtered reaction mixture was then refluxed without the catalyst for the next 5 h; no further formation of the corresponding product was observed, indicating that no homogeneous catalyst was involved. Atomic absorption spectroscopic analysis of the liquid phase obtained by filtering (hot) the reaction mixture after 5 h revealed that no silver was leached from mesoporous MSAGN-10 and the prepared catalyst is heterogeneous in nature.
Effect of aerial oxygen or moisture towards the activity of the catalyst. In the presence of MSAGN-10 (preheated at 100 °C for 5 hours) the reaction of benzenethiol to diphenyl disulphide occurred with 91% yield without formation of any other side-products. The same reaction in the presence of MSAGN-10 after being exposed to ambient atmosphere for 10 days produced similar observation. Therefore, the catalyst had considerable stability towards heat, air and moisture.
Catalyst recyclability. The reusability of the bottle-neck like mesoporous MSAGN-10 catalyst was examined for benzenethiol to diphenyl disulfide formation reaction in water by using the recycled catalyst. After completion of the reaction, the reaction mixture was diluted with EtOAc (10 mL) and extracted with EtOAc and the catalyst was separated by simple filtration and was washed thoroughly with acetone and distilled water and dried. The product was collected from the ethyl acetate mixture. The catalyst was heated at 150 °C for 4 h and reused for next catalytic cycle. The catalyst was recycled for five times (Fig. 11). The separated catalyst was dry under vacuum. To know the stability of the reused catalyst, TEM and IR analysis of reused catalyst were examined. The results are shown in the ESI, Fig. S1 and S2† respectively.
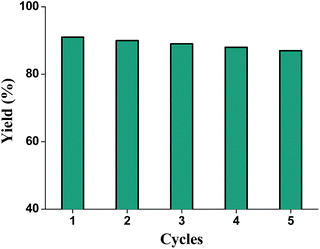 |
| Fig. 11 Recycling efficiency of the silver incorporated mesoporous bottle neck like silica catalyst (MSAGN-10) in the oxidation of thiophenol. | |
Conclusion
In conclusion, Ag2O nanoparticle dispersed on mesoporous silica with large bottle neck like mesopores has been synthesized and characterized by using sophisticated analytical techniques like N2 sorption analysis, HR TEM in combination with EDS elemental analysis, FTIR and powder X-ray diffraction studies. The scope of the method is almost general since it is successful for aliphatic, aromatic (with electron-rich and electron-poor substituents), and heteroaromatic thiols. Additionally, it is compatible with the presence of other functional groups in the molecule. Particularly highly sensitive imine bond is well retained under this mild condition. Synthesis using water as a solvent is of paramount importance for its sustainable green impact.44 Further, water and oxygen were the only by-product which added to its attractiveness. High catalytic efficiency of mesoporous MSAGN-10 reported herein for the synthesis of disulfides may open up new opportunities in heterogeneous catalysis for the synthesis of value-added disulfides. Moreover, this catalytic oxidation system takes on the clean, safety and operationally simple characteristic, and the yields of the products are high, so the oxidation method meets the needs of contemporary green chemistry and is suitable for practical synthesis.
Experimental
Materials and instrumentation
The 1H- and 13C-NMR analyses were carried out on Bruker-Advance Digital 300 MHz instruments in CDCl3 with TMS as an internal reference. IR spectra were recorded in KBr pellets in reflection mode on a Perkin Elmer RX-1 FTIR spectrophotometer. CHN analysis was performed using a Perkin-Elmer 2400 Series II CHN analyzer. X-ray single crystal analysis was performed in a Bruker-AXS SMART APEX II diffractometer equipped with a graphite monochromator. N2 sorption analysis was performed on a Quantachrome Autosorb 1C surface area analyzer at liquid nitrogen temperature (77 K). Prior to the analysis the sample was degassed at 413 K for 3 h under high vacuum. Transmission electron microscopic images were recorded on a JEOL 2010 TEM operated at 200 kV in Indian Association for the Cultivation of Science, Jadavpur, Kolkata 700032, India. Powder X-ray diffraction (PXRD) patterns of the Ag-loaded samples were recorded on a Bruker AXS D-8 Advance diffractometer operated at 40 kV voltage and 40 mA current.
Preparation of catalyst (MSAGN-10)
In a typical procedure, 390 g (390 mL) water and 317 g (400 mL) MeOH were taken together in a 1 litre open beaker fitted with a magnetic stirrer. Then 3.52 g CTAB (cetyltrimethylammonium bromide) was added at room temperature (30–35 °C) and stirred for 30 min. After a clear solution was obtained, tetraethylorthosilicate (TEOS) was added drop wise from a dropping funnel under stirring condition. Next, 133.34 mg (this weight corresponds to MSAGN-10) AgNO3 was added and the stirring was continued for another 5 min. Then 10 mL 0.4 N NaOH solution was added drop wise taking the time period of 1 h. The stirring was continued for the next 8 h at room temperature and then aged overnight (12–14 h) at room temperature. It was filtered and washed thoroughly with deionized water and dried at 35–40 °C for 5 days. The dry powder was calcined at 550 °C for 6–8 h under static air.
Representative procedure for the atmospheric oxidation of thiols to disulphides (2)
A mixture of thiol 1 (1 mmol), and mesoporous MSAGN-10 catalyst (20 mg) in water (4 mL) were heated under reflux for 5 h. After completion of the reactions (monitored by disappearance of the starting material in the thin layer chromatography), ethyl acetate (10 mL) was added to the whole reaction mixture and the solid catalyst was separated from the mixture by filtration. The recovered catalyst was washed several times with water and then acetone, dried in a desiccator and stored for another consecutive reaction run. Organic compounds were afforded by evaporation of the solvent in a rotary evaporator and the residue was subjected to silica gel (60–120 mesh) column chromatography using ethyl acetate–petroleum ether (0
:
100) as eluents. The products were characterized by standard analytical techniques such as 1H & 13C NMR, FTIR, elemental analysis, melting point determination, HRMS, X-ray crystallographic analysis and all gave satisfactory results.
Representative procedure for the atmospheric oxidation of imine thiols to disulphides (4)
A mixture of imine thiol 3 (1 mmol), and mesoporous MSAGN-10 catalyst (20 mg) in water (4 mL) were heated under reflux for 5 h. After completion of the reactions (monitored by disappearance of the starting material in the thin layer chromatography), ethyl acetate (10 mL) was added to the whole reaction mixture and the solid catalyst was separated from the mixture by filtration. The recovered catalyst was washed several times with water and then acetone, dried in a desiccator and stored for another consecutive reaction run. Organic compounds were afforded by evaporation of the solvent in a rotary evaporator and the solid residue was finally recrystallized from ethyl acetate–petroleum ether. The products were characterized by standard analytical techniques such as 1H & 13C NMR, FTIR, elemental analysis, melting point determination, X-ray crystallographic analysis and all gave satisfactory results.
Acknowledgements
We thank the Council of Scientific and Industrial Research, New Delhi for fellowship (SRF to both PD and SR, SPM to BB).
Notes and references
-
(a) Y. Kanda and T. Fukuyama, J. Am. Chem. Soc., 1993, 115, 8451 CrossRef CAS;
(b) B. D. Palmer, G. W. Newcastle, A. M. Thompson, M. Boyd, H. D. H. Showalter, A. D. Sercel, D. W. Fry, A. J. Kraker and W. A. Dennyyrosine, J. Med. Chem., 1995, 38, 58 CrossRef CAS;
(c) B. Schmidt, S. Lindman, W. Tong, G. Lindeberg, A. Gogoll, Z. Lai, M. Thornwall, B. Synnergren, A. Nilson, C. J. Welch, M. Sohtell, C. Westerlund, F. Nyberg, A. Karlen and A. Hallberg, J. Med. Chem., 1997, 40, 903 CrossRef CAS PubMed;
(d) G. Pattenden and A. Shuker, J. Chem. Soc., Perkin Trans. 1, 1992, 1215 RSC;
(e) K. D. Lee, G. Saito and J. A. Swanson, Adv. Drug Delivery Rev., 2003, 55, 199 CrossRef.
-
(a) Organic Sulfur Chemistry: Structure and Mechanism, ed. S. Oae, CRS Press, Boca Raton FL, USA, 1991 Search PubMed;
(b) R. J. Cremlyn, An Introduction to Organosulfur Chemistry, Wiley & Sons, New York, 1996 Search PubMed;
(c) H.-W. Engels, in Ullmann's Encyclopedia of Industrial Chemistry, ed. B. Elvers, S. Hawkins, W. Russey and G. Schulz, Cambridge, New York, 5th edn, 1993, vol. A23, p. 365 Search PubMed;
(d) K. Ramadas and N. Srinivasan, Synth. Commun., 1995, 25, 227 CrossRef CAS;
(e) D. L. Holbrook, Handbook of Petroleum Refining Processes, ed. R. A. Meyers, McGraw-Hill, New York, 1996, ch. 11.3 Search PubMed.
-
(a) A. L. Schwan and R. R. Strickler, Org. Prep. Proced. Int., 1999, 31, 579 CrossRef CAS;
(b) W. Ge and Y. Wei, Green Chem., 2012, 14, 2066 RSC.
- J. Srogl, J. Hývl, Á. Révészb and D. Schröder, Chem. Commun., 2009, 3463 RSC.
- S. N. Maiti, P. Spevak, M. P. Singh and R. G. Micetich, Synth. Commun., 1988, 18, 575 CrossRef CAS.
- M. Montazerozohori, S. Joohari, B. Karami and N. Haghighat, Molecules, 2007, 12, 694 CrossRef CAS.
- E. P. Papadopoulos, A. Jarrar and C. H. Issidoides, J. Org. Chem., 1966, 31, 615 CrossRef CAS.
- C. Lopez, A. Conzales Cossio and C. Palomo, Synth. Commun., 1985, 15, 1197 CrossRef CAS.
- J. Choi and N. M. Yoon, J. Org. Chem., 1995, 60, 3266 CrossRef CAS.
- M. Hirano, S. Yakabe, H. Monobe and T. Marimoto, J. Chem. Res., Synop., 1998, 472 RSC.
- E. Abele, R. Abele and E. Lukevics, J. Chem. Res., Synop., 1999, 624 RSC.
-
(a) M. Hirano, S. Yakabe, K. Ando and T. Morimoto, J. Chem. Res., Synop., 1998, 816 RSC;
(b) M. Montazerozohori, B. Karami and M. Azizi, ARKIVOC, 2007, i, 99 CrossRef.
- H. Imanieh, S. Ghamami, M. K. Mohammadi and A. Jangjoo, Russ. J. Gen. Chem., 2007, 77, 282 CrossRef CAS.
- D. N. Dhar and A. K. Bag, Indian J. Chem., Sect. B: Org. Chem. Incl. Med. Chem., 1984, 23, 974 Search PubMed.
- J. Choi and N. M. Yoon, J. Org. Chem., 1995, 60, 3266 CrossRef CAS.
-
(a) J. B. Arterburm, M. C. Perry, S. L. Nelson, B. R. Dible and M. S. Holguin, J. Am. Chem. Soc., 1997, 119, 9309 CrossRef;
(b) K. Tanaka and K. Ajiki, Tetrahedron Lett., 2004, 45, 5677 CrossRef CAS PubMed.
- E. J. Corey and D. L. Boger, Tetrahedron Lett., 1978, 28, 2461 CrossRef.
-
(a) M. K. Mohammadi, S. Ghammamy, S. Zarrinabadi, M. H. Farjam and B. Sabayan, Chin. J. Chem., 2010, 28, 2199 CrossRef CAS;
(b) M. N. Bhattacharjee, M. K. Chauduri and H. S. Dasgupta, Synthesis, 1982, 588 CrossRef CAS.
-
(a) G. S. Chaubey, S. M. Das and K. Mahanti, Croat. Chem. Acta, 2003, 76, 287 CAS;
(b) A. Christoforou, G. Nicolaou and Y. Elemes, Tetrahedron Lett., 2006, 47, 9211 CrossRef CAS PubMed.
- S. C. Banfield, A. T. Omori, H. Leish and T. Hudlcky, The cost of the Burgess reagent from Aldrich is $50–60 per gram, J. Org. Chem., 2007, 72, 4989 CrossRef CAS PubMed.
- M. Oba, K. Tanaka, K. Nishiyama and W. Ando, J. Org. Chem., 2011, 76, 4173 CrossRef CAS PubMed.
-
(a) P. J. Chai, Y. S. Li and C. X. Tan, Chin. Chem. Lett., 2011, 22, 1403 CrossRef CAS PubMed;
(b) M. Montazerozohori and L. Z. Fradombe, Phosphorus, Sulfur Silicon Relat. Elem., 2010, 185, 509 CrossRef CAS.
- A. Dewan, U. Bora and D. K. Kakati, Heteroat. Chem., 2012, 23, 231 CrossRef CAS.
- N. Iranpoor and B. Zeynizadeh, Synthesis, 1999, 49 CrossRef CAS PubMed.
- A. Saxena, A. Kumar and S. Mozumdar, J. Mol. Catal. A: Chem., 2007, 269, 35 CrossRef CAS PubMed.
-
(a) D. Singh, F. Z. Galetto, L. C. Soares, O. E. D. Rodrigues and A. L. Braga, Eur. J. Org. Chem., 2010, 14, 2661 CrossRef;
(b) S. Thurow, V. A. Pereira, D. M. Martinez, D. Alves, G. Perin, R. G. Jacob and E. J. Lenardão, Tetrahedron Lett., 2011, 52, 640 CrossRef CAS PubMed;
(c) S. M. S. Chauhan, A. Kumar and K. A. Srinivas, Chem. Commun., 2003, 2348 RSC;
(d) H. T. Abdel-Mohsen, K. Sudheendran, J. Conrad and U. Beifuss, Green Chem., 2013, 15, 1490 RSC;
(e) J. L. G. Ruano, A. Parra and J. Alemán, Green Chem., 2008, 10, 706 RSC.
-
(a) T. Chatterjee and B. C. Ranu, RSC Adv., 2013, 3, 10680 RSC;
(b) R. Ozen and F. Aydin, Monatsh. Chem., 2006, 137, 307 CrossRef CAS.
-
(a) P. Hu, Q. Wang, Y. Yan, S. Zhang, B. Zhang and Z. Wang, Org. Biomol. Chem., 2013, 11, 4304 RSC;
(b) A. K. Patra, A. Dutta, M. Pramanik, M. Nandi, H. Uyama and A. Bhaumik, ChemCatChem, 2014, 6, 220 CrossRef CAS.
-
(a) Z. Qu, S. Shen, D. Chen and Y. Wang, J. Mol. Catal. A: Chem., 2012, 356, 171 CrossRef CAS PubMed;
(b) C. B. Zhang, H. He and K. Tanaka, Appl. Catal., B, 2006, 65, 37 CrossRef CAS PubMed.
- X. F. Tang, Y. G. Li, X. M. Huang, Y. D. Xu, H. Q. Zhu, J. G. Wang and W. J. Shen, Appl. Catal., B, 2006, 62, 265 CrossRef CAS PubMed.
- C. Y. Li, Y. N. Shen, M. L. Jia, S. S. Sheng, M. F. Adebajo and H. Y. Zhu, Catal. Commun., 2008, 9, 355 CrossRef CAS PubMed.
-
(a) J. Taghavimoghaddam, G. P. Knowles and A. L. Chaffee, J. Mol. Catal. A: Chem., 2012, 358, 79 CrossRef CAS PubMed;
(b) G. Laugel, J. Arichi, M. Molière, A. Kiennemann, F. Garin and B. Louis, Catal. Today, 2008, 138, 38 CrossRef CAS PubMed;
(c) A. Martinez, C. López, F. Márquez and I. Diaz, J. Catal., 2003, 220, 486 CrossRef CAS;
(d) D. Yin, W. Li, W. Yang, H. Xiang, Y. Sun, B. Zhong and S. Peng, Microporous Mesoporous Mater., 2001, 47, 15 CrossRef CAS;
(e) Y. Ohtsuka, Y. Takahashi, M. Noguchi, T. Arai, S. Takasaki, N. Tsubouchi and Y. Wang, Catal. Today, 2004, 89, 419 CrossRef CAS PubMed.
- M. N. Khan, S. Pal, T. Parvin and L. H. Choudhury, RSC Adv., 2012, 2, 12305 RSC.
-
(a) S. Ray, P. Manna and C. Mukhopadhyay, Ultrason. Sonochem., 2015, 22, 22 CrossRef CAS PubMed;
(b) S. Ray, A. Bhaumik, M. Pramanik and C. Mukhopadhyay, RSC Adv., 2014, 4, 15441 RSC;
(c) S. Ray, A. Bhaumik, A. Dutta, R. J. Butcher and C. Mukhopadhyay, Tetrahedron Lett., 2013, 54, 2164 CrossRef CAS PubMed;
(d) S. Ray, B. Banerjee, A. Bhaumik, A. Dutta and C. Mukhopadhyay, Catal. Commun., 2015, 59, 97 CrossRef PubMed.
- P. Wang, C. N. Moorefield and G. R. Newkome, Angew. Chem., Int. Ed., 2005, 44, 1679 CrossRef CAS PubMed.
- S. K. Das, M. K. Bhunia, D. Chakraborty, A. R. Khuda-Bukhsh and A. Bhaumik, Chem. Commun., 2012, 48, 2891 RSC.
- A. Mitra and A. Bhaumik, Mater. Lett., 2007, 61, 659 CrossRef CAS PubMed.
- M.-J. Kim, S. Kim, H. Park and Y.-D. Huh, Bull. Korean Chem. Soc., 2011, 32, 3793 CrossRef CAS.
-
(a) S. Duhan, S. Devi and M. Srivastava, Indian J. Pure Appl. Phys., 2010, 48, 271 CAS;
(b) C. H. Bartholomew and R. J. Farrauto, J. Catal., 1976, 45, 41 CrossRef CAS;
(c) J. Phillips, A. Auroux, J. Massardier and A. Renouprez, J. Phys. Chem., 1993, 97, 3565 CrossRef CAS.
- H. Sharma, N. Singh and D. O. Jang, Green Chem., 2014, 16, 4922 RSC.
- T. A. Rokob, L. Rulíšek, J. Šrogl, Á. Révész, E. L. Zins and D. Schröder, Inorg. Chem., 2011, 50, 9968 CrossRef CAS PubMed.
- M. Bakthadoss, R. Selvakumar and J. Srinivasan, Tetrahedron Lett., 2014, 55, 5808 CrossRef CAS PubMed.
- M. G. White and J. W. Hightower, J. Catal., 1983, 82, 185 CrossRef CAS.
-
(a) P. Das, R. J. Butcher and C. Mukhopadhyay, Green Chem., 2012, 14, 1376 RSC;
(b) C. Mukhopadhyay, P. Das and R. J. Butcher, Org. Lett., 2011, 13, 4664 CrossRef CAS PubMed;
(c) P. Das, S. Ray and C. Mukhopadhyay, Org. Lett., 2013, 15, 5622 CrossRef CAS PubMed;
(d) S. Ray, M. Brown, A. Bhaumik, A. Dutta and C. Mukhopadhyay, Green Chem., 2013, 15, 1910 RSC;
(e) S. Ray, A. Bhaumik, A. Dutta and C. Mukhopadhyay, Catal. Sci. Technol., 2013, 3, 1267 RSC;
(f) P. Das, A. Dutta, A. Bhaumik and C. Mukhopadhyay, Green Chem., 2014, 16, 1426 RSC;
(g) S. Ray, P. Das, A. Bhaumik, M. Pramanik and C. Mukhopadhyay, Recoverable Recyclable Catal., 2014, 1, 34 Search PubMed;
(h) S. Ray, P. Das, A. Bhaumik, A. Dutta and C. Mukhopadhyay, Appl. Catal., A, 2013, 458, 183 CrossRef CAS PubMed.
Footnote |
† Electronic supplementary information (ESI) available. CCDC 1024098 and 1024099. For ESI and crystallographic data in CIF or other electronic format see DOI: 10.1039/c4ra14802a |
|
This journal is © The Royal Society of Chemistry 2015 |
Click here to see how this site uses Cookies. View our privacy policy here.