DOI:
10.1039/C4RA13558J
(Paper)
RSC Adv., 2015,
5, 9683-9694
Surface-active drug loaded lipopolymeric nanohybrid aerosol therapy: potential non-invasive way to mitigate lipopolysaccharide mediated inflammation in murine lungs†
Received
31st October 2014
, Accepted 5th January 2015
First published on 6th January 2015
Abstract
In the present study, surface-active lipopolymeric nanohybrids (LPH) measuring 200–300 nm were developed and evaluated for their efficacy as non-invasive aerosol therapy to reduce lung inflammation and lung injury in rats caused by 5 mg per kg body weight intratracheally administered lipopolysaccharide (LPS). The nanoparticles comprised of phospholipids DPPC and POPG along with polymeric anti-oxidant D-α-tocopheryl polyethylene glycol 1000 succinate (TPGS) or dexamethasone disodium phosphate (DXP) or both. The formulation closely mimics surface tension modulation properties like innate pulmonary surfactant (PS). The dual drug LPH (Dual-LPH) aerosol therapy provided maximum beneficial effect compared to individual drug loaded nanoparticle aerosol treatment. Chest X-rays and lung tissue histopathological studies revealed that Dual-LPH aerosol therapy at a dose of 200 mg phospholipid + 50 mg TPGS + 2 mg DXP per kg body weight significantly reduced inflammation, damage to the alveolar network and pulmonary hemorrhage in LPS injured rats. Significant reduction in levels of neutrophils, total protein, oxidative stress and inflammatory cytokines like TNF-α, IL-1β and IL-6 in bronchoalveolar lavage fluid (BALF) was seen in the Dual-LPH treated group. BALF capillary patency studies revealed rise in capillary patency from 0% for LPS injured group to 99.7 ± 0.1% for Dual-LPH treated group, which is comparable to that of innate surfactant in healthy conditions, indicating that Dual-LPH treatment helped in opening occluded airways. Surface-active TPGS helped in scavenging the free radicals in the injured lungs whereas DXP helped in modulating the inflammation pathway. This multipronged approach can thus serve as a potential non-invasive treatment modality for reducing lung inflammation in lung injury cases.
1 Introduction
Aerosolized environmental pollutants, bacteria, viruses and nanoengineered non-biodegradable particles have been known to cause lung injuries1,2 leading to lung inflammation, pulmonary edema and alveolar epithelial cell apoptosis.3,4 Pulmonary surfactant (PS), a complex mixture of lipids and proteins that line the air–liquid interface is crucial for normal respiratory process and preventing collapse of the alveolar network. In the case of lung inflammation/injury, the high oxidative stress alters the lipid composition of PS and its functional nanostructures like lamellar bodies, tubular myelin5 rendering it dysfunctional,6 augmenting respiratory distress and aggravating lung injury. Steroids are potent anti-inflammatory drugs commonly used in lung inflammation cases and administered systemically for maximum therapeutic effect.7 However many of these steroids also exert high mineralocorticoid effects warranting their cautious systemic use.
Localized delivery in the lungs in aerosolized form would not only reduce the steroid dose but also serve as non-invasive route to directly deliver drug to target region thereby potentially lowering the systemic side effects. The method of generation of drug aerosol, its composition and particle size greatly determines the extent and distribution of drugs in the lungs. Clinically many corticosteroids have been administered as inhalable dry powder or nebulizable solutions for treating asthma, chronic obstructive pulmonary disease.8 These aerosolized drug carriers measuring 1–5 μm in size are designed to deliver drugs in the conducting bronchial airways by sedimentation process but have limited reach to remote alveolar region which is highly inflamed in lung injury cases.9 Nanoparticles measuring <0.5 μm can be of potential benefit as they can travel to greater depth in the lungs and deposit drugs in the remote alveolar region via diffusion process.9,10 In severe lung injury cases inactivation of PS by high-localized oxidative stress increases alveolar air–liquid surface tension leading to collapse of alveolar network and atelectasis. Current clinically used nebulized drug solutions or dry powder inhalation formulations are not sufficiently surface-active to open up these occluded/collapsed airways thereby impairing drug deposition in the inflamed alveoli. Intratracheal administration of exogenous PS like Curosurf™ and Survanta™ has been quite successful in restoring airway patency and alleviation of respiratory distress in neonatal respiratory distress syndrome where PS is deficient.11 These exogenous PS have been of limited success in lung injury cases, as they are rendered dysfunctional by the high oxidative stress in alveolar milieu.12–14 In addition, these surfactants have reported to raise some immunological concerns due their animal origin.13 Hydrophilic polymers like polyethylene glycol, dextran and chitosan addition have reportedly shown to improve resistance to PS inactivation.15,16 However till date they have being just used as adjuvant to surfactant therapy and not extensively investigated for potential use as therapeutic drug delivery systems for treating lung inflammation. There is a need for drug loaded nanoparticles system that can mimic PS, be more resistant to oxidative stress and which is capable of opening up occluded airways and deliver drug into the remotest alveolar region. We have developed aerosolizable amphiphilic polymer–lipid self assembled nanohybrids that mimics PS structurally and functionally; as well as concomitantly and non-invasively deliver anti-oxidants and anti-inflammatory drugs in lipopolysaccharide (LPS) injured lungs. The developed nanohybrids have been designed to be robust enough to retain surface tension modulating properties in oxidative stress environment and simultaneously help in replenishing the depleted PS reserves in the inflamed lungs.
Nanohybrids composed of phospholipids 1,2-dipalmitoyl-sn-glycerol-3-phosphatidylcholine (DPPC) and 1-palmitoyl-2-oleoyl-sn-glycerol-3-phosphoglycerol ammonium salt (POPG) at 9
:
1 w/w ratio along with surface active polymeric anti-oxidant D-α-tocopheryl polyethylene glycol 1000 succinate (TPGS) and an anti-inflammatory drug dexamethasone disodium phosphate (DXP) were developed. DPPC and POPG are also present in PS. They are known to play crucial role in adsorption kinetics17,18 and form an ideal mixture at the above ratio.19 TPGS is a conjugate of vitamin E and polymer PEG 1000. PEGs have been well documented to improve the PS resistance to surfactant dysfunction.16 Unlike the conventional “only lipid” surfactants, the lipopolymeric nanohybrid provides a unique combination of lipids, drug and polymer TPGS for replenishing functional phospholipid pool and simultaneously deliver drugs to the inflamed alveoli. TPGS was added at 25 wt% of phospholipid for maximum beneficial effect on surface activity of the phospholipids.17,18 Based on literature evidence, DXP dose was limited to 2 mg per kg body weight.20 Lipopolysaccharide (LPS) is known to elicit an acute inflammatory response in lungs progressing to lung injury3 and has been used to model for respiratory infection and sepsis condition in animal studies.21 In the present study, intratracheally instilled LPS from E. coli O111:B4 was used to elicit inflammatory response in rat lungs. Surface activity and efficacy of three aerosolized drug loaded nanoparticles viz. dexamethasone loaded nanovesicles [DPPC
:
POPG (9
:
1 w/w)]
:
DXP (1
:
0.01 w/w) nanovesicles (DXP-NV), TPGS loaded nanohybrids [DPPC
:
POPG (9
:
1 w/w)]
:
TPGS (1
:
0.25 w/w) (TPGS-LPH) and dexamethasone and TPGS loaded nanohybrids [[DPPC
:
POPG (9
:
1 w/w)]
:
TPGS (1
:
0.25w/w)]
:
DXP (1
:
0.01 w/w) lipopolymeric nanohybrids (Dual-LPH) were evaluated for their ability to reduce oxidative stress in LPS mediated lung injury.
2 Materials and methods
2.1 Materials
Synthetic DPPC (>99% purity) and POPG (>99% purity) were purchased from Lipoid GmbH, Ludwigshafen, Germany and used without further purification. HPLC grade chloroform and methanol were used for preparation of DPPC and POPG stock solutions. D-α-tocopheryl polyethylene glycol 1000 succinate (TPGS) was obtained as gift sample from Eastman Chemicals, USA. USP grade dexamethasone disodium phosphate (DXP) was gifted by Kirit works tablets Mumbai, India. Methanol and acetone analytical grade used for cleaning the Langmuir–Blodgett trough were obtained from Sisco Research Laboratories, Mumbai, India. 18.2 MΩ cm resistivity ultrapure deionized water obtained from Milli Q UV plus system (Millipore Corp., USA) was used for subphase preparation. Sodium chloride (99.9% pure), sodium hydroxide (NaOH) and analytical grade calcium chloride dihydrate (CaCl2·2H2O) used for preparing the subphase were purchased from Sisco Research Laboratories Mumbai, India. Phosphotungstic acid and uranyl acetate were purchased from Qualigen, Mumbai, India. Dulbecco's modified essential medium (DMEM), bovine serum albumin, fetal bovine serum (FBS) and 1% antibiotic antimycotic solutions was purchased from Himedia, Mumbai, India. Human adenocarcinomic alveolar basal epithelial cells (A549 cell lines) were purchased from National Centre for Cell Science (NCCS) Pune, India. Lyophilized and γ-irradiated lipopolysaccharide from E. coli O111:B4 was purchased from Sigma Aldrich, USA. RayBio® rat serum and tissue lysate TNF-α, IL-1β and IL-6 estimation 96 well plates ELISA kits were purchased from RayBiotech Inc., USA. Ketamine and xylazine were purchased from local pharmacy against prescription.
2.2 Methods
2.2.1 Dynamic surface tension modulation: mixed films compression–expansion studies. The dynamic surface tension modulation property was evaluated using Langmuir–Blodgett trough (KSV Instruments, Finland). Lipid mixed films were studied on a Teflon trough of 323 × 75 sq mm having capacity to accommodate ∼220 ml of subphase. The subphase used was 0.9% saline containing 2 mM CaCl2·2H2O with pH adjusted to 7.4 using 0.1 N NaOH. The temperature of subphase in the trough was maintained at 37 ± 0.5 °C by means of an external circulating water bath. The mixed films formed at the interface was compressed and expanded laterally by two horizontally moving Delrin barriers at a speed of 121 mm min−1. This speed although, not a true representative of rate of breathing, is the maximum speed at which the films can be compressed without destabilizing the lipid film. Sand blasted gold Wilhelmy plate was used to detect surface pressure. The electronic feedback system measures the change in the surface pressure at the interface as the lipid film is compressed and expanded. Change in surface pressure (π) is noted for the change in the surface area (A) at constant temperature yielding surface pressure area (π/A) isotherms.Surface pressure is related to surface tension (γ) by the following equation
where
γ0 = surface tension of clean subphase
γ = surface tension in presence of the surfactant.
The Teflon trough was first cleaned with deionized water followed by methanol. The trough was filled with 0.9% saline containing 2 mM CaCl2·2H2O (pH 7.4). Organic solutions of DPPC
:
POPG, TPGS-LPH, DXP-NV and Dual-LPH prepared in chloroform
:
methanol (2
:
1 v/v) mixture were spread at the interface using Hamilton glass syringe. All the films were evaluated by addition of different proportions of POPG, TPGS and DXP to DPPC film of mean molecular area of 110 Å2. Volumes of the samples spread at the interface were adjusted so as to have same amount of DPPC as required for 110 Å2 DPPC film.17 The film was allowed to dry for 30 minutes before compression. All the studies were carried out at physiological temperature and the films were alternately compressed and expanded at 121 mm min−1 to simulate breathing process and data collected was from second compression–expansion cycle. The mixed films were compressed to maximum 15 percent of original surface area. Minimum surface tension (i.e. maximum surface pressure) achieved at the lowest film surface area was noted from the second compression cycles in all the experiments. Unlike first compression–expansion cycle that is representative of first breath at birth, second compression–expansion cycle data was selected as it provides better assessment of formulations surface tension modulation capability as well as ability to respread and readsorb to hyphophase as would be expected during normal breathing process. Although we had started with known amount of lipids, drugs and polymer at the interface, some of it is lost into the hypophase during the first compression cycle and would re-adsorb depending on the adsorption rates of the individual materials. Hence, the amount of lipid, drugs and polymer that is available for second compression cycle need not necessarily be the same as in first compression cycle and is difficult to estimate. As the films were compressed and expanded to known surface area and because of difficulty in accurately determining how many lipid, drug and polymer molecules are at the air–liquid interface at the start of the second compression cycle, the surface pressure changes were plotted against percent surface area as X-axis instead of mean molecular area.
2.2.2 Preparation of lipopolymeric nanohybrid (LPH) and nanovesicles. LPH were prepared by thin film hydration method.18 Briefly DPPC and POPG were dissolved in chloroform
:
methanol (2
:
1 v/v) mixture and dried in a rotary vacuum evaporator at 40 °C to form thin films. The lipid films were then hydrated with 0.9% saline containing 2 mM CaCl2·2H2O (pH 7.4) with TPGS or TPGS + DXP at 45 °C for 60 minutes with continuous stirring yielding 40 mg phospholipid per ml LHP suspension. Samples were probe sonicated (150V/T Ultrasonic Homogenizer, BioLogics, Inc, USA) for 1 minute at 40% power settings. Samples were diluted with hydration media to yield 1 mg phospholipid per ml LPH for electron microscopy studies. DXP loaded nanovesicles were prepared in same manner but without addition of TPGS.
2.2.3 Dual-LPH atomic force microscopy (AFM) and transmission electron microscopy (TEM) imaging. Atomic force microscopy of the nanoparticles spread on glass cover slip (10 mm × 10 mm) was done using Nanoscope IV scanning probe microscope (Digital Instruments, Santa Barbara, USA) with phosphorous-doped silicon tip having radius less than 10 nm. The 5 × 5 μm substrate area was scanned in tapping mode at 1 Hz to obtain a topographical image of the particles. TEM imaging was done by negative staining of the samples with osmium tetroxide and uranyl acetate and visualized under TEM (PHILIPS, CM200, USA) at 120 kV.
2.2.4 Capillary patency of the formulation. Capillary surfactometer compared the surface activity of the nanoparticles in terms of their capacity to maintain the capillaries patent in comparison to natural pulmonary surfactant.17 Open-ended capillaries with middle constricted region of 0.25 mm nominal inner diameter were used to simulate terminal airways. 0.5 μl of formulation suspension is instilled in the constricted portion of capillary and connected to the bellows and maintained at 37 ± 0.1 °C. Air is forced from lower to upper end of capillary. Resistance to air flow due to pathway occlusion is measured via pressure transducer. As air is forced, a point is reached when the liquid is pushed out of the constricted portion thereby opening the air passage and reducing the air resistance to zero (patent capillary). A functional surface-active formulation unlike deactivated/dysfunctional surfactant does not re-occlude the narrow region and maintains the capillary patent. The percentage opening of the capillary for a period of 120 seconds was recorded.
2.2.5 Cellular uptake of LPH. Confocal Laser Scanning Microscopy (CLSM) was done to visualize nanoparticles cellular uptake and mechanism of internalization in human adenocarcinoma lung epithelial cells (A549 cell line) that have features of alveolar type 2 cells (AE2) that synthesize PS. A549 cell line is a well-known in vitro model that has been extensively used to study surfactant synthesis pathway and particulate materials interactions.22,23 Since A549 cells closely resemble alveolar type 2 cells, the particle interaction with A549 cells would also correlate to normal alveolar type 2 cells to great extent. Cellular uptake of Dual-LPH particles by A549 cells was carried out. Fluorescent dye calcein loaded Dual-LPH nanoparticles were prepared by thin film hydration method as explained in the section 2.2.2 with 0.1% w/v calcein added during hydration phase. Unencapsulated dye was separated by centrifugation at 25
000g for 10 minutes. A549 cells were grown on a sterile coverslip (1 × 105 cells per well) in sterile 24 well tissue culture plates (Nunc, USA) containing 1 ml DMEM and incubated for 24 h. The used media was discarded after 24 h and replaced with 1 ml of 0.1% w/v calcein solution or calcein-Dual-LPH (1 mg lipid per ml) particles suspended in DMEM media and incubated for 3 h at 37 °C in incubator under 5% CO2 and 95% air atmosphere. A549 cells were also incubated with calcein-Dual-LPH (1 mg ml−1) at 4 °C in refrigerator. After 3 h, sample solutions were discarded and the coverslips with adhering cells washed with phosphate buffer and fixed with 10% formaldehyde in phosphate buffer saline. The samples were mounted using glycerol on glass slides and used for confocal laser scanning microscopy (CLSM). Slides were viewed on Olympus Lx81-LWPO microscope (Japan) at 490 nm excitation and 525 nm emission wavelength filters with argon laser under 60× water immersion objective piece and 10× eyepiece. Single plane and Z-scan fluorescence imaging of the cells was done at same exposure level for all the samples and processed using Olympus Fluoview FV 500 software (Japan). Fluorescence intensity profiling was done to determine extent of LPH internalization.
2.3 Lung injury induction and in vivo formulation efficacy studies
Animal studies were approved by the Animal Care Ethics Committee of the Mumbai Veterinary College, Mumbai, India. 30 Wistar rats weighing 180–220 g were used for the study. The animals were housed in ventilated cages and were provided food and water ad libitum. Rats were divided in five groups with six rats in each group. Rats were anesthetized with intraperitonial injection of ketamine–xylazine. E. coli lipopolysaccharide (LPS) dissolved in normal saline (5 mg per kg body weight) was administered intratracheally in 4 of the 5 groups.24 Control healthy group received normal saline (NS) intratracheally. All groups were transferred in separate static inhalation chambers and administered aerosolized dose of 200 mg phospholipid per kg bodyweight of DXP-NV, TPGS-LPH and Dual-LPH formulations (6 ml each per group) respectively in aerosolized form using jet nebulizer. Control injured group was treated with 6 ml of aerosolized NS. Animals were observed for any adverse symptoms every 30 minutes for 8 h from time of LPS instillation. After 8 h, two randomly selected rats from each group were taken for chest X-ray and for lung histopathology studies. Lung function parameters like respiratory rate, minute volume and maximum inspiratory volumes were measured for the remaining rats in Harvard double chamber plethysmograph (USA). Four rats were sacrificed and tracheal cannula was inserted in the lungs and washed with three 4 ml portion of ice cold NS. Approximately 11 ml of bronchoalveolar lavage fluid (BALF) was collected. BALF was processed and analyzed for % neutrophil, total protein and pulmonary hemorrhage. BALF lipid peroxidation was carried out as described in one of our previous research paper.18 Ray Biotech rat IL-1β, IL-6 and TNF-α ELISA kits were used to determine the concentration of respective cytokines in the collected BALF.
2.4 Statistical analysis
All the experiments, unless otherwise specified were carried out in triplicates and are represented as mean ± standard deviation. Statistical significance of the results was analyzed by one-way analysis of variance (ANOVA) at 95% confidence limit. For assessing any significant differences between groups Newman–Keul's test for statistical significance at 95% confidence limit was used.
3 Results and discussion
Controlling inflammation is extremely crucial for effective treatment for lung injury. Studies have shown that high oxidative stress reduces the functional population of PS leading to respiratory distress.6 The anatomy of the respiratory tract, presence of alveolar edema and atelectasis limits the reach and effectiveness of intratracheally administered exogenous surfactants and other localized drug therapies. Conventionally, invasive intravenous or intraperitoneal modes have been used to deliver anti-oxidant and anti-inflammatory drugs25 for controlling inflammation in lungs. Dexamethasone at high dose of 10 mg per kg body weight (intraperitoneal) alone or in combination with anti-oxidant like N-acetyl cysteine has been found to be effective in reducing lung inflammation in injured rats.26,27 Although hydrophilic polymers have shown to improve PS functions in diseased condition,15,28–30 very limited research has been done to explore use of nanoparticle based drug delivery systems for treating lung inflammation. Aerosolized surface-active nanoparticles that improved alveolar deposition, co-deliver surfactants and drugs can serve as better treatment regime for treating lung injury. We have developed lipopolymeric nanohybrid particles that can replenish the depleted surfactant pool and also act as vehicle for delivering drugs TPGS and DXP to control inflammation.
3.1 Surface pressure–area isotherm studies
Functional pulmonary surfactant lining the alveolar air–liquid interface dynamically modulates surface tension from ∼40 mN m−1 at the end of inspiration to less than 1 mN m−1 at end of exhalation. This ability of PS film to dynamically modulate surface tension to near zero value, which is very crucial for reducing work of breathing and preventing alveolar collapse, is severely impaired in acute lung injury cases. To mimic pulmonary surfactant, the developed formulation also needs to possess dynamic surface tension modulations capability similar to that of natural surfactant. Fig. 1a–d shows surface pressure–area isotherms of the mixed films of DPPC
:
POPG, TPGS-LPH, DXP-NV and Dual-LPH. Dynamic changes in the surface pressure were seen with changes in surface area. TPGS-LPH, DXP-NV and Dual-LPH reached minimum surface tensions MSTs of 0.1 ± 0.1, 0.54 ± 0.11 and 1.94 ± 1.38 mN m−1 respectively, which are comparable to that reported for PS.31 This clearly implies that these drug-loaded nanocarriers closely resemble PS in terms of modulating surface tension at air–liquid interface and are least likely to cause detrimental effect to surface tension properties of PS.
 |
| Fig. 1 Surface pressure–area isotherms [a] DPPC : POPG (9 : 1 w/w), [b] TPGS-LPH, [c] DXP-NV and [d] Dual-LPH mixed films at 37 °C (n = 3). All the lipid–drug mixed films reached high surface pressure of 69–70 mN m−1 at 15% of the original interfacial area. Solid and open squares represent compression and expansion curve respectively. Each curve is represents average of three different experiments. | |
3.2 Atomic force microscopy (AFM) and transmission electron microscopy (TEM) imaging
TEM imaging (Fig. 2a) of Dual-LPH formulation revealed the particles to be spherical structures made up of unilamellar phospholipid bilayer and measured 200–300 nm diametrically. Fig. 2b shows AFM image of Dual-LPH formulation. The Dual-LPH particles were found to be uniform non-agglomerating with particle size in the range of 200–260 nm (Fig. 2b and c). Particles size of the aerosolized therapeutic system plays a very crucial role in the drug deposition profile in the lungs. Particles having aerodynamic diameter of 0.5–2 μm are known to travel to greater depth in lungs and deposit more in the alveolar region.32,33 Studies have also show high deposition of 300–100 nm particles in the alveolar region.18,34 These 200–260 nm size Dual-LPH aerosolized nanoparticles are less likely to be deposited in the conducting airways and are more likely to travel to greater depth in the lungs and deposit drug in the remotest alveoli via diffusion process.
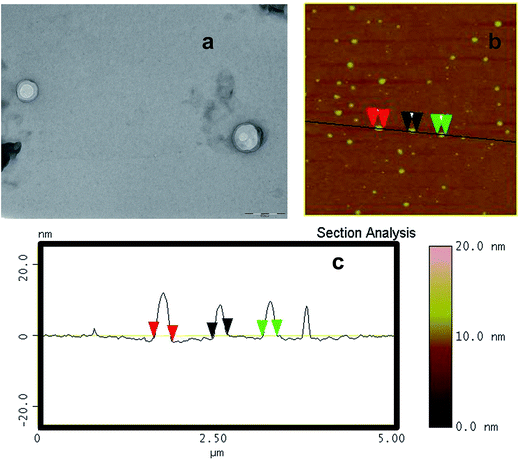 |
| Fig. 2 [a] TEM image of Dual-LPH particles. Scale bar = 500 nm [b] AFM image of Dual-LPH nanoparticles and [c] sectional dimensions of Dual-LPH nanoparticles along the black line drawn in AFM image [b]. | |
3.3 Capillary patency
TPGS-LPH, DXP-NV and Dual-LPH formulations maintained 95.3 ± 4.1, 97.2 ± 2.3 and 99.7 ± 0.1% capillary patency respectively, which is comparable to 100% maintained by innate PS. However, Dual-LPH exhibited capillary patency that closely resembles with natural PS, indicating that it has surface tension properties closely matching to that of natural PS. This formulation is least likely to occlude the airways upon deposition in the lungs as aerosol and hence was further investigated for cellular uptake studies. In addition, the high surface activity and nanosize of the Dual-LPH together would also aid in easy generation of smaller aerosol particles that are conducive for aerosol based alveolar drug delivery. Particles having aerodynamic diameter 0.5–2 μm are known to travel to greater depth in lungs and deposit more in the alveolar region32,33 and hence Dual-LPH particles are suitable for alveolar delivery of drugs.
3.4 Dual-LPH cellular uptake
Calcein is a membrane impermeable fluorophore commonly used as tracer molecule for nanoparticle cellular uptake studies.35 A549 cell line The A549 cells incubated with 0.1% w/v calcein solution showed no fluorescence (Fig. 3a) confirming the inability of dye to enter into the cell from the external medium. Fig. 3b shows the 3-D image of A549 cells treated with calcein loaded Dual-LPH formulations. The intense green fluorescence in cellular matrix at same exposure levels is due to calcein released in the A549 cell cytoplasm following LPH uptake (Fig. 3c). CLSM Z-scan fluorescence microscopy confirmed presence of the green fluorescence across various cell depths indicating that the dye is present in the cellular cytoplasm and not just on the cell surface. The cellular uptake (and consequently the fluorescence) of calcein loaded Dual-LPH was significantly reduced following incubating at 4 °C compared to incubation at 37 °C (Fig. 3d) suggesting the process to be ATP dependent. The AE2 cells synthesize pulmonary surfactant, which plays a crucial role in the normal lung breathing process and physiology. In most of the inflammatory pulmonary disease, the high oxidative stress results in inflammation of these cells or even apoptosis leading to reduction or stoppage of pulmonary surfactant production leading to alveolar collapse and hypoxia. Therefore it is of utmost importance to reduce the inflammation and also to replenish the surfactant pool in the cells. Although A549 cells are cancerous version of the normal AE2, both exhibit many similar features including lung surfactant synthesis and cellular uptake mechanism. Therefore the results of particle interaction with the A549 cells can be extended to that with AE2 cells. As A549 cells closely resemble AE2 cells, they have been extensively used as model to mimic the AE2 cells in many cellular uptake, drug deposition and efficacy studies.
 |
| Fig. 3 [a] Fluorescence microscopy image of A549 cells incubated with 0.1% w/v calcein at 37 °C. [b] 3D reconstruction of calcein-Dual-LPH internalized A549 cells. Fluorescence microscopy images and intensity profile of A549 cell incubated with calcein-Dual-LPH formulation at [c] 37 °C and [d] 4 °C. Scale bar represents fluorescence intensity of the calcein-Dual-LPH internalized A549 cells. | |
Dexamethasone exerts anti-inflammatory action by binding to the glucocorticoid receptor in cell cytoplasm causing induction of mitogen-activated protein kinase (MAPK) phosphatase and production of annexin I thereby down regulating cytosolic phospholipase A2α expression and lowering production inflammatory mediators like leukotrienes. It also down regulates NF-κB transcription reducing production of proinflammatory cytokines like IL-1β, IL-6 and TNF-α and cell adhesion proteins like ICAM-1.27,36 TPGS is a conjugate of vitamin E and PEG 1000. It primarily acts as an anti-oxidant in the alveolar milieu by scavenging free radicals. It also inserts into the lipid membrane and protects the unsaturated phospholipids from free radical mediated lipid peroxidation. Vitamin E also plays a crucial role in lung surfactant biosynthesis.37 The cellular uptake of these drugs is important for reducing production of inflammatory mediators and for protecting the innate surfactant pool in the cells. Dual-LPH contains phospholipids like DPPC, POPG that are also present in the naturally occurring functional PS. Thus, the active cellular uptake of Dual-LPH nanohybrids by A549 cells suggests that in addition to increasing local drug concentration in alveolar hypophase, they can potentially serve as an excellent vehicle to directly deliver drugs into the inflamed cells, reduce oxidative load and most crucially concomitantly replenish the depleted surfactant pool resulting in better inflammation control.
3.5 In vivo studies
3.5.1 Chest X-ray and histopathological studies. LPS induced lung injury has been extensively used as model for inflammation and sepsis lung condition in respiratory infection and lung injury.3,24,38 LPS are glycolipids made up of repeating disaccharide group attached to polar lipid head group that is primarily responsible for immunological responses. The antigenic polar lipid head group activates alveolar macrophages that release various inflammatory mediators like TNF-α, IL-1β and chemokine monocyte attractant protein-1 that modulate neutrophil recruitment in the lungs.39 Chest X-ray of rats from different treatment groups is shown in Fig. 4a–c. In comparison to control group (Fig. 4a), LPS + NS showed the presence of irregular hazy patch in all quadrants of the chest region because of fluid accumulation in the lungs (Fig. 4b). The chest regions were distinctively clearer in case of LPS + NS treated with Dual-LPH formulations (Fig. 4c) indicative of marked reduction in fluid accumulation in the lungs. Histopathological changes in lungs as a result of LPS mediated lung injury and treatment with Dual-LPH aerosol are depicted in Fig. 4d–f. The normal lung alveolar architecture as seen in control group (Fig. 4d) is significantly damaged in LPS + NS group, and exhibited high leukocyte infiltration, multifocal hemorrhage and diffused distribution of edematous fluid compared to normal lung tissue (Fig. 4e). LPS injured rats treated with Dual-LPH exhibited markedly reduced alveolar tissue damage, lesser leukocyte infiltration and fewer hemorrhagic areas (Fig. 4f), indicating aerosol therapy helps in reducing extent of LPS mediated lung injury. High neutrophil migration and diffused edematous patches in lung tissue of LPS injured rats treated with either TPGS-LPH and DXP-NV aerosol (see ESI) amply proved the benefits of using Dual-LPH over single drug therapy. The activated neutrophils in turn release reactive oxygen species (ROS) that cause lipid peroxidation of functional surfactant and damage lung tissue. LPS causes apoptosis of capillary endothelium3 leading to extensive pulmonary hemorrhage and diffused edematous fluid patches which is also observed in chest X-ray and histological section of lung tissue derived from LPS injured group.
 |
| Fig. 4 Chest X-rays of rat lungs from [a] NS + NS, [b] LPS + NS and [c] LPS + Dual-LPH treated groups. Hazy consolidated patches (White arrow) in image [b] are as a result of collection of edematous fluid in the LPS injured rats. Minimal chest congestion and fluid collection was seen in lungs of LPS injured rats treated with Dual-LPH aerosol. Hematoxylin and eosin stained histopathological section of rat lung tissues from [d] NS + NS, [e] LPS + NS and [f] LPS + Dual-LPH treated groups. LPS injured group [e] showed extensive neutrophil accumulation, diffused pulmonary hemorrhage and total destruction of alveolar network compared to control group [d]. Occasional hemorrhagic patches, retention of alveolar network and significantly lesser neutrophil migration into the alveolar tissue is seen in LPS injured rats treated with Dual-LPH aerosol [f]. | |
3.5.2 BALF total protein and pulmonary haemorrhage estimation. BALF from LPS + NS group contained 0.67 ± 0.09 g per dl of protein which is significantly higher than in control NS + NS group (0.225 ± 0.05 g dl−1). The total protein content in BALF of LPS injured groups treated with Dual-LPH, TPGS-LPH and DXP-NV aerosol were 0.275 ± 0.09, 0.25 ± 0.1 and 0.3 ± 0.17 g dl−1 respectively which were significantly lower than LPS + NS group (ANOVA, p < 0.05) (Fig. 5a). Pulmonary hemorrhage was determined by measuring optical density (OD) of BALF at 412 nm. NS + NS and LPS + NS groups BALF ODs are 0.333 ± 0.047 and 0.511 ± 0.09 respectively indicating significant pulmonary hemorrhage in LPS injured group (ANOVA, p < 0.05). BALF ODs for LPS groups treated with aerosolized Dual-LPH, TPGS-LPH and DXP-NV were 0.346 ± 0.034, 0.363 ± 0.033 and 0.423 ± 0.019 respectively which were significantly lower than LPS injured group (ANOVA, p < 0.05) (Fig. 5b). Thus all the three formulation helped in reducing pulmonary hemorrhage and total protein content in lung fluid in LPS injured lungs.
 |
| Fig. 5 [a] Total protein and [b] pulmonary hemorrhage in terms of optical density (O.D.) of BALF and [c] BALF capillary patency, from various groups (N = 4). *, ** and *** represent significant difference at p < 0.05, p < 0.01 and p < 0.001 respectively by Newman–Keuls analysis following ANOVA at 95% confidence limit. | |
3.5.3 Capillary patency studies. BALF from NS + NS group maintained 88.2 ± 1.9% capillary patency compared to 0.05 ± 0.1% by LPS + NS group (Fig. 5c). BALF collected from LPS injured group treated with Dual-LPH and TPGS-LPH formulations showed significantly higher capillary patency of 90.6 ± 2.8 and 83.7 ± 5.2% respectively (ANOVA, p < 0.05) in comparison to LPS + NS group. BALF collected for LPS injured group treated with DXP-NV maintained 0.125 ± 0.1% capillary patency indicating that dexamethasone alone did not keep airways patent upon LPS mediated lung injury. BALF from Dual-LPH treated group maintained highest airway patency (By Newman–Keuls analysis), indicating concomitant addition of TPGS and DXP aided in improving surface activity and helped to maintain capillary patent in LPS injured condition. Proteins like albumin, which are present in edematous fluid, are known to reduce capillary patency by competitive inhibition of PS.14 Treating LPS injured rats especially with Dual-LPH helped to replenish level of surfactant in lungs, which, along with reduced protein content in BALF leads to improved capillary patency to ∼93%.
3.5.4 BALF percent neutrophil and lipid peroxidation studies. The number of neutrophils present in BALF was represented as percentage of the total leucocytes population counted in the BALF samples. BALF from LPS injured group had significantly high percent of neutrophil accumulation (72 ± 3%) in comparison to NS + NS group (52 ± 6%) (Fig. 6a). BALF of Dual-LPH, TPGS-LPH and DXP-NV treated group contained 40 ± 12, 78 ± 2 and 68 ± 10% neutrophils respectively. Marked reduction in neutrophil recruitment is seen in Dual-LPH treated group (ANOVA, p < 0.05) and comparable to that found in NS + NS group. Oxidative stress was measured as thiobarbituric acid reactive substance (TBARS). A higher value of TBARS would imply higher oxidative stress. BALF from LPS + NS group has significantly higher TBARS of 1.40 ± 0.21 μM in comparison to 1.00 ± 0.03 μM for NS + NS group (Fig. 6b). The high capillary permeability in injured group results in higher recruitment and activation of neutrophils in the alveoli that further increases oxidative stress in the lung milieu and cause lung surfactant dysfunction. Neutrophil recruitment in lungs was reduced in Dual-LPH treated groups along with associated lower TBARS values in BALF pointing towards reduced inflammation and lesser oxidative stress in lungs. BALF of Dual-LPH, TPGS-LPH and DXP-NV formulation treated groups have significantly reduced TBARS of 0.76 ± 0.08, 0.80 ± 0.09 and 0.99 ± 0.13 μM respectively in comparison to LPS injured group (ANOVA, p < 0.05) suggesting that the formulations helped to reduce lipid peroxidation of PS and oxidative stress in the LPS injured rat lungs. The reduced neutrophil recruitment helps to lower oxidative stress in lungs.
 |
| Fig. 6 [a] % neutrophils in BALF from different treatment groups [b] lipid peroxidation measured as TBARS (N = 4). *, ** and *** represent significant difference at p < 0.05, p < 0.01 and p < 0.001 respectively by Newman–Keuls analysis following ANOVA at 95% confidence limit. NS = not significant. | |
3.5.5 BALF cytokine levels. IL-1β levels (922.4 ± 87.0 pg ml−1) in LPS injured group were significantly higher than control group (71.7 ± 26.4 pg ml−1) (Fig. 7a). BALF from groups treated with Dual-LPH, TPGS-LPH and DXP-NV aerosol showed significantly lower IL-1β concentrations of 592.3 ± 73.5, 545.6 ± 191.2 and 569.3 ± 61.3 pg ml−1 respectively in comparison to injured group (ANOVA, p < 0.05). IL-6 levels were also significantly higher in LPS + NS groups (4360.0 ± 866.3 pg ml−1) compared to NS + NS group (77.8 ± 10.6 pg ml−1) (ANOVA, p < 0.05). IL-6 levels in BALF from Dual-LPH, TPGS-LPH and DXP-NV treated groups were 2018.9 ± 659.0, 2685.5 ± 636.9 and 2639.9 ± 1381.0 pg ml−1 respectively. The IL-6 levels were significantly reduced in Dual-LPH treated rats (ANOVA, p < 0.05, Newman–Keuls analysis) compared to the LPS + NS group (Fig. 7b). TNF-α levels were significantly higher in LPS + NS group (3221.4 ± 82.9 pg ml−1) compared to NS + NS group (62.5 ± 10.7 pg ml−1) (ANOVA, p < 0.05). BALF from Dual-LPH and DXP-NV aerosol treated group had 1764.1 ± 1179.1 and 1382.7 ± 657.6 pg ml−1 of TNF-α, which were significantly less in comparison to LPS + NS group (ANOVA, p < 0.05, Newman–Keuls analysis) (Fig. 7c). TPGS-LPH treated group did not significantly lower TNF-α level in comparison to injured group. Thus it can be seen from the above results, that amongst the three developed formulations, Dual-LPH is most effective in reducing the expression of inflammatory cytokines and thus can potentially help to reduce inflammation in the injured lungs. This might be because of the synergistic effect of combination of TPGS and DXP. TPGS contains vitamin E that helps in reducing the local reactive oxygen species load in the lungs through its free radical scavenging property. Apart from its antioxidant properties, vitamin E is known to attenuate macrophage inflammatory protein-1-alpha and macrophage chemo attractant protein-1, which are involved in the process of acute lung injury.40 Vitamin E reduces trans endothelial membrane migration of neutrophils in the alveoli,41 resulting in reduced oxidative stress in the lungs. Addition of DXP on the other hand helped in modulation of macrophage activity thereby controlling release of inflammatory mediators.42 The beneficial effects of combined TPGS and DXP therapy is clearly seen in the form of marked reduction in levels of inflammatory cytokines like TNF-α and IL-6 that were not significantly reduced with single drug formulation aerosol therapy. Conventionally, invasive intravenous or intraperitoneal modes have been used to deliver anti-oxidant and anti-inflammatory drugs25 for controlling inflammation in lungs. Dexamethasone at high dose of 10 mg per kg body weight (intraperitoneal) alone or in combination with anti oxidant like N-acetyl cysteine has been found to be effective in reducing lung inflammation in injured rats.26,27 In the current study, aerosolized nanoparticles not only helps in directly co-delivering anti-oxidant and anti-inflammatory drugs non-invasively into the lungs, but also, significantly reduce the effective dexamethasone dose to 2 mg per kg body weight to control lung inflammation.
 |
| Fig. 7 [a] IL-1β, [b] IL-6 and [c] TNF-α levels in BALF of various treated groups (N = 3). *and ** represents significant difference at p < 0.05 and p < 0.01 respectively by Newman–Keuls analysis following ANOVA at 95% confidence limit. | |
3.5.6 Lung function parameters. LPS injured saline treated group (LPS + NS) had significantly higher per minute respiratory rate of 129 ± 12 compared to 82 ± 5 min−1 for control group (NS + NS) indicative of respiratory distress (ANOVA, p < 0.05). Respiratory rates in groups treated with aerosolized Dual-LPH, TPGS-LPH and DXP-NV formulations were 77 ± 12, 90 ± 5 and 94 ± 15 min−1 respectively (Fig. 8a), which were significantly less in comparison to that of LPS + NS group (ANOVA, p < 0.05). LPS treated group had significantly higher minute volume of 164.1 ± 33.5 ml compared to 63.4 ± 7.9 ml in control group, indicating hyperventilation in LPS injured rats (Fig. 8c). Dual-LPH, TPGS-LPH and DXP-NV aerosol treated groups had significantly low minute volumes of 99.1 ± 23.9, 89.2 ± 39.2 and 100.2 ± 2.4 ml respectively in comparison to LPS injured group (ANOVA, p < 0.05). Control and LPS injured groups treated with Dual-LPH, TPGS-LPH and DXP-NV aerosol had maximum inspiratory flow of 4.25 ± 0.6, 5.7 ± 1.5, 6.8 ± 3.0 and 5.8 ± 0.4 ml s−1 respectively which were significantly different compared to 11.3 ± 4.9 ml s−1 of LPS + NS group (ANOVA, p < 0.05) (Fig. 8b). Neman Keuls analysis revealed that all the three surface-active nanoparticle aerosols were equally effective in reducing respiratory distress. High respiratory rates, minute volume and maximum inspiratory flow seen in LPS injured rats pointed toward hyperventilation and respiratory distress. The labored breathing was significantly reduced with the aerosol therapy of the developed formulations.
 |
| Fig. 8 [a] Respiratory rate, [b] maximum inspiratory flow (ml s−1) and [c] minute volume of rats (ml) from different treatment groups. N = 4. *, ** and *** represent significant difference at p < 0.05, p < 0.01 and p < 0.001 respectively by Newman–Keuls analysis following ANOVA at 95% confidence limit. | |
3.6 Role of nanotechnology, combination therapy and its implications
A lot of research is currently been done in developing “smart” drug delivery systems that are much more target specific, reduce unwanted side effects and improve therapeutic efficacy. Treating inflammation in injured lungs would require lowering the oxidative stress, neutralizing the causative agents for lung injury, restoration of normal lung function and aiding repair process. Lipid or polymer based drug delivery systems have been previously evaluated for delivering drugs ranging from antibiotics to corticosteroids as aerosols,43 but till date there are no reports of any self-assembling amphiphilic polymer–lipid hybrid nanosystems like the one discussed in this paper been used as aerosol. The lipid component of the developed lipopolymeric nanohybrid imparts more PS-like attributes to the particles whereas the anti-oxidant polymeric component provides higher resistance against free radicals and surfactant inactivation. In the current study, aerosolized nanoparticles not only helps in non-invasively co-delivering anti-oxidant and anti-inflammatory drugs directly into the lungs, but also, significantly reduce the effective dexamethasone dose to 2 mg per kg body weight to control lung inflammation compared to 10 mg per kg body weight intra peritoneal injection as reported in literature.27 This can be probably attributed to use of combination of TPGS and DXP that have different mechanism of action thereby providing synergistic effect in controlling lung inflammation.
4 Conclusions
In this paper we have shown LPS mediated lung injury in rats can be controlled by non-invasive aerosol therapy of surface-active nanoparticles. Due to surface-activity, nanosize, and ability to concomitantly deliver two drugs within alveolar cells, the Dual-LPH aerosol therapy helped to reduced inflammation and oxidative stress in the injured lungs. Dual anti-inflammatory and anti-oxidant loaded surfactant nanoparticle aerosols may serve as a promising treatment modality in mitigating endotoxin mediated lung injury. Improved drug penetration and distribution can be achieved by drug loaded nanoparticles, providing new opportunities of using drugs like TPGS, that have to the best of our knowledge, never been attempted to be delivered as aerosol. Such nanoparticles can also serve as platform technology to deliver conventionally used anti-inflammatory drugs like steroids locally into the lungs without causing extensive systemic side effects. In vivo efficacy studies in different lung injury models using different injury causing agents will be highly crucial in establishing the true potential of such drug delivery systems in treatment of lung injury cases.
Acknowledgements
The authors acknowledge Department of Biotechnology, Government of India for funding the project and Indian Institute of Technology Bombay (IIT Bombay) for research facilities and Dr Gaikwad of Nuclear Medicine, Mumbai Veterinary College for providing facilities for animal study. Shah and Banerjee acknowledge Industrial Research and Consultancy Centre, IIT Bombay for TEM and Sophisticated Analytical Instrument Facility, IIT Bombay for AFM imaging.
References
- S. Bakand, A. Hayes and F. Dechsakulthorn, Inhalation Toxicol., 2012, 24, 125–135 CrossRef CAS PubMed.
- A. K. Madl and K. E. Pinkerton, Crit. Rev. Toxicol., 2009, 39, 629–658 CrossRef CAS PubMed.
- C. Y. Chuang, T. L. Chen, Y. G. Cherng, Y. T. Tai, T. G. Chen and R. M. Chen, Arch. Toxicol., 2011, 85, 209–218 CrossRef CAS PubMed.
- C. R. Bernard, Am. J. Respir. Crit. Care Med., 2005, 172, 798–806 CrossRef PubMed.
- A. S. Kaviratna, A. R. Shah, S. Rao and R. Banerjee, Nanotechnology I. Dynamic Biochemistry, Process Biotechnology and Molecular Biology 3, 2009, vol. 3, pp. 21–32 Search PubMed.
- S. Andersson, A. Kheiter and T. A. Merritt, Lung, 1999, 177, 179–189 CrossRef CAS.
- D. Annane, Chest, 2007, 131, 945–946 CrossRef PubMed.
- M. Y. Yang, J. G. Y. Chan and H.-K. Chan, J. Controlled Release, 2014, 193, 228–240 CrossRef CAS PubMed.
- A. J. Hickey, J. Controlled Release, 2014, 190, 182–188 CrossRef CAS PubMed.
- N. R. Labiris and M. B. Dolovich, Br. J. Clin. Pharmacol., 2003, 56, 588–599 CrossRef CAS.
- L. R. Wiseman and H. M. Bryson, Drugs, 1994, 48, 386–403 CrossRef CAS PubMed.
- Y. Sun, Y. Q. Wang, R. Yang, J. J. Zhu, Y. Y. Le, J. G. Zhong and J. Lu, Pulm. Pharmacol. Ther., 2009, 22, 253–259 CrossRef CAS PubMed.
- V. J. Erpenbeck, A. Hagenberg, Y. Dulkys, J. Elsner, R. Bälder, H. Krentel, M. Discher, A. Braun, N. Krug and J. M. Hohlfeld, Am. J. Respir. Crit. Care Med., 2004, 169, 578–586 CrossRef PubMed.
- G. Enhorning, Chest, 2008, 133, 975–980 CrossRef CAS PubMed.
- J. J. Lu, W. W. Y. Cheung, L. M. Y. Yu, Z. Policova, D. Li, M. L. Hair and A. W. Neumann, Respir. Physiol. Neurobiol., 2002, 130, 169–179 CrossRef CAS.
- K. W. Lu, H. William Taeusch, B. Robertson, J. Goerke and J. A. Clements, Am. J. Respir. Crit. Care Med., 2001, 164, 1531–1536 CrossRef CAS PubMed.
- A. R. Shah and R. Banerjee, Colloids Surf., B, 2011, 85, 116–124 CrossRef CAS PubMed.
- A. R. Shah and R. Banerjee, Soft Matter, 2012, 8, 11911–11922 RSC.
- T. Wiedmann, A. Salmon and V. Wong, Biochim. Biophys. Acta, Lipids Lipid Metab., 1993, 1167, 114–120 CrossRef CAS.
- E. C. O'Leary and S. H. Zuckerman, Am. J. Respir. Cell Mol. Biol., 1997, 16, 267–274 CrossRef PubMed.
- H. Chen, C. Bai and X. Wang, Expert Rev. Respir. Med., 2010, 4, 773–783 CrossRef CAS PubMed.
- M. Lieber, B. Smith and A. Szakal, Int. J. Cancer, 1976, 17, 62–70 CrossRef CAS.
- J. Zhang, X. G. Chen, W. B. Peng and C. S. Liu, J. Nanomed. Nanotechnol., 2008, 4, 208–214 CrossRef CAS PubMed.
- A. H. Jansson, C. Eriksson and X. Wang, Lung, 2004, 182, 163–171 CrossRef CAS PubMed.
- S. J. Kao, D. Wang, H. I. Lin and H. I. Chen, Clin. Exp. Pharmacol. Physiol., 2006, 33, 33–40 CrossRef CAS PubMed.
- E. Wigenstam, D. Rocksn, B. Ekstrand-Hammarstrm and A. Bucht, Inhalation Toxicol., 2009, 21, 958–964 CrossRef CAS PubMed.
- D. Rocksén, B. Lilliehöök, R. Larsson, T. Johansson and A. Bucht, Clin. Exp. Immunol., 2000, 122, 249–256 CrossRef.
- N. Kang, Z. Policova, G. Bankian, M. L. Hair, Y. Y. Zuo, A. W. Neumann and E. J. Acosta, Biochim. Biophys. Acta, Biomembr., 2008, 1778, 291–302 CrossRef CAS PubMed.
- I. Minkov, K. Mircheva, N. Grozev, I. Tz and I. Panaiotov, Colloids Surf., B, 2013, 101, 135–142 CrossRef CAS PubMed.
- H. W. Taeusch, E. Dybbro and K. W. Lu, Colloids Surf., B, 2008, 62, 243–249 CrossRef CAS PubMed.
- H. Zhang, Y. E. Wang, Q. Fan and Y. Y. Zuo, Langmuir, 2011, 27, 8351–8358 CrossRef CAS PubMed.
- J. S. Patton and P. R. Byron, Nat. Rev. Drug Discovery, 2007, 6, 67–74 CrossRef CAS PubMed.
- P. J. Kuehl, T. L. Anderson, G. Candelaria, B. Gershman, K. Harlin, J. Y. Hesterman, T. Holmes, J. Hoppin, C. Lackas, J. P. Norenberg, H. Yu and J. D. McDonald, Inhalation Toxicol., 2012, 24, 27–35 CrossRef CAS PubMed.
- M. Geiser and W. G. Kreyling, Part. Fibre Toxicol., 2010, 7, 2 CrossRef PubMed.
- Y. Hu, T. Litwin, A. R. Nagaraja, B. Kwong, J. Katz, N. Watson and D. J. Irvine, Nano Lett., 2007, 7, 3056–3064 CrossRef CAS PubMed.
- T. Rhen and J. A. Cidlowski, N. Engl. J. Med., 2005, 353, 1711–1723 CrossRef CAS PubMed.
- F. Guthmann, I. Kolleck, C. Schachtrup, M. Schlame, F. Spener and B. Rüstow, Free Radical Biol. Med., 2003, 34, 663–673 CrossRef CAS.
- G. Matute-Bello, C. W. Frevert and T. R. Martin, Am. J. Physiol., 2008, 295, L379–L399 CAS.
- B. Beck-Schimmer, R. Schwendener, T. Pasch, L. Reyes, C. Booy and R. C. Schimmer, Respir. Res., 2005, 6, 61 CrossRef PubMed.
- K. Uchiyama, H. Takano, R. Yanagisawa, K.-i. Inoue, Y. Naito, N. Yoshida, S. Yoshino, H. Murase, T. Ichinose and T. Yoshikawa, Clin. Exp. Pharmacol. Physiol., 2004, 31, 226–230 CrossRef CAS PubMed.
- D. Rocksén, B. Ekstrand-Hammarström, L. Johansson and A. Bucht, Am. J. Respir. Cell Mol. Biol., 2003, 28, 199–207 CrossRef PubMed.
- Y. Nakamura, T. Murai and Y. Ogawa, Eur. Respir. J., 1996, 9, 301–306 CrossRef CAS.
- P. Muralidharan, E. Mallory, M. Malapit, H. Don Jr and H. M. Mansour, Pharmaceutics, 2014, 6, 333–353 CrossRef CAS PubMed.
Footnotes |
† Electronic supplementary information (ESI) available. See DOI: 10.1039/c4ra13558j |
‡ Current work address: Department of Oncology and The Institute of Biomedical Engineering, University of Oxford, Old Road Campus Research Building, Roosevelt Drive, Oxford, OX3 7DQ, UK. |
|
This journal is © The Royal Society of Chemistry 2015 |