DOI:
10.1039/C4RA12513D
(Paper)
RSC Adv., 2015,
5, 12755-12762
Novel screen printed potentiometric sensors for the determination of oxicams
Received
16th October 2014
, Accepted 15th January 2015
First published on 15th January 2015
Abstract
The construction and performance characteristics of new sensitive and selective sensors based on functionalized multi-walled carbon nanotubes/β-cyclodextrin nanocomposite (FMWCNTs/β-CD) was demonstrated for potentiometric determination of different anti-inflammatory agents including lornoxicam, meloxicam, piroxicam and tenoxicam. Screen printed sensors (SPEs) modified with FMWCNTs/β-CD composite, hyamine (Hy) and 2-fluorophenyl 2-nitrophenyl ether (f-PNPE) showed proper electroanalytical performances with a Nernstian compliance range between 61.2 and 52.6 mV decade−1 activity and a detection limit of 6.0 × 10−7 mol L−1 for different oxicam derivatives. Modification with carbon nanotube composite as sensing material remarkably improved the potential stability and lifetime of the fabricated sensors. The proposed sensors offer a simple analytical tool for determination of different oxicam derivatives in their pharmaceutical formulations under batch and flow injection analysis (FIA) conditions.
1. Introduction
The project that produced the novel anti-arthritic and anti-inflammatory agent piroxicam (PXM, 4-hydroxy-2-methyl-N-2-2-pyridinyl-2H-1,2-benzothiazine-3-carboxamide-1,1-dioxide, Feldene; Pfizer) began in 1962 and led to the product launching into key European markets in 1980.1 Later, other oxicam derivatives including; tenoxicam (TXM, 4-hydroxy-2-methyl-N-(pyridine-2-yl)-2H-thieno [2,3-e]-1,2-thiazine-3-carboxamide-1,1-dioxide) and meloxicam (MXM, 4-hydroxy-2-methyl-N-(5-methyl-2-thiazolyl)-2H-1,2-benzo-thiazine-3-carboxamide-1,1-di-oxide) were widely prescribed as medication. Lornoxicam (LXM 6-chloro-4-hydroxy-2-methyl-N-2-pyridyl-5H-thieno-[2,3-e]-1,2-thiazine-2-carboxamide-1,1-dioxide) is a recent non-steroidal drug of the oxicam class with analgesic, anti-inflammatory and antipyretic properties with a relatively short elimination half-life, which may be advantageous from a tolerability standpoint.2
Different official and non-official methods for analysis of oxicams (OXMs) were found in the literature.3,4 Bibliography data3 indicate that only liquid chromatographic methods with UV5 or MS detection6 have been used for LXM determination. In addition, a few spectrophotometric7 and voltammetric ones8 can also be found in the literature.
Nevertheless, most of these methods require expensive apparatus and involve several manipulation steps before the final result of analysis. Electrochemical methods are elegant approaches in analytical chemistry and an interest in developing electrochemical-sensing devices for environmental monitoring, clinical assays or process control is growing rapidly.9–11 Potentiometric methods using ion selective electrodes (ISEs) have the advantages of simplicity, short analysis time with adequate precision and accuracy.12,13 To the best of our knowledge, no ISE was reported in literature for potentiometric determination of either LXM or MXM, and only polyvinyl chloride (PVC) sensors were found for PXM and TXM.14,15 More recently, carbon paste electrode (CPE) based on multi-walled carbon nanotubes/β-cyclodextrin composite (MWCNTs/β-CD-CPE) was introduced for potentiometric determination of PXM.16
Conventional PVC and CPEs are inconvenient for large-scale routine analysis taking into account their construction, size, necessity of conditioning before measurements and the requirement for sterilization or cleaning to avoid infection or contamination. Several clinical and environmental applications would benefit from the availability of low-cost disposable sensors. Screen printing seems to be one of the most promising technologies allowing sensors to be produced on a large-scale with the advantages of optimized manufacturing repeatability, long shelf-lifetime and application the field measurements with portable small instruments.17,18
Carbon nanotubes (CNTs) are excellent sensor materials due to their high conductivity, chemical inertness and large surface area. Incorporation of CNTs in electrode matrices improves the conductivity and transduction of the chemical signal to electrical signal, which in turn improved the dynamic working range and response time.19 Moreover, defects in the graphite structure, at both end and side walls of CNTs, enables the functionalization of CNTs via either covalent or noncovalent modifications to catalyze electron transfer kinetics. Functionalization of nanotubes with guest structures will lead to new composite materials possessing the properties of each component, or even with a synergistic effect, which consequently improve the sensor performance.16,19,20
The aim of the present work is to introduce disposable screen printed electrodes (SPEs) incorporated with FMWCNTs/β-CD composite as potentiometric sensors that can be used in drug quality control. The developed methods are simple, rapid, accurate, precise and sensitive for the determination of different oxicam derivatives in various dosage forms under batch and FIA conditions.
2. Experimental
2.1. Reagents
All reagents were of the analytical grade and bidistilled water was used throughout the experiments. Cyclodextrin derivatives including; α-CD (I, Aldrich), γ-CD (II, Bio Basic Inc.), native β-CD (III, Sigma), heptakis 2,6-di-O-methyl-β-CD (IV, Aldrich), and heptakis 2,3,6-tri-O-methyl-β-CD (V, Aldrich) were used as sensing ionophores. Multi-walled carbon nanotubes (MWCNTs, Aldrich) were used for preparation of β-cyclodextrin composite (FMWCNTs/β-CD) as described in details elsewhere.16
Hyamine®1622 (Hy, Fluka), cetylpyridinium chloride (CPC, Fluka), hexadecyltrimethylammonium bromide (HTMAB, Fluka), tri-dodecyltrimethylammonium bromide (TDTMAB, Sigma), didodecyl-dimethylammonium bromide (DDMAB, Fluka) and Septonex (Sp, Hlohovec, CZ) were tested as ionic sites.
The tested electrode plasticizers were as following; o-nitrophenyl octyl ether (o-NPOE, Sigma), 2-fluorophenyl 2-nitrophenyl ether (f-PNPE, Fluka), dioctylphthalate (DOP, Sigma), dioctylsebacate (DOS, Avocado) and tricresylphosphate (TCP, Fluka). Polyvinyl chloride (PVC, relative high molecular weight, Aldrich) and graphite powder (synthetic 1–2 μm, Aldrich) were used for preparation of the printing ink.
2.2. Authentic samples
Different oxicam authentic sample were kindly provided from National Organization for Drug Control and Research, Giza, Egypt. Stock drug solutions (10−2 mol L−1) were prepared by dissolving the appropriate amount of the active ingredient in 5 × 10−2 mol L−1 NaOH solution and kept at 4 °C.
2.3. Pharmaceutical preparations
Zeficam® tablets (Eva Pharma, Egypt, 8 mg LXM/tablet), Moxen® tablets (EGDT, Egypt, 7.5 mg MXM/tablet), Tenoxil® tablet (Sigma/MPC, Egypt, 20 mg TXM/tablet) and Dispercam® tablets (MUP Cairo, Egypt, 20 mg PXM/tablet) were purchased from local drug stores. Ten tablets were weighed, grinded, and an accurate weight of the powder equivalent to one tablet was dissolved in 5 × 10−2 mol L−1 NaOH. The sample was filtered, completed to 25 mL and analyzed using the proposed method and compared with the official method for each drug.21–24
2.4. Biological samples
Aliquots of the biological fluid (2 mL urine or plasma, obtained from healthy male 12 h after an intake of one Zeficam-tablet) were spiked with standard LXM solution. Spiked samples were treated with 0.1 mL of 70% perchloric acid, diluted to 10 mL with water, vortexed for 1.0 min and centrifuged for 10 min at 13
000 rpm. Supernatants were adjusted to pH 10 with NaOH and diluted to 25 mL with water and analyzed according to the proposed protocol and official method.24
2.5. Apparatus
Potentiometric measurements were carried out using a 692-pH meter (Metrohm, Herisau, Switzerland). A single line flow injection system, composed of four channel peristaltic pump (MCP Ismatec, Zurich, Switzerland), sample injection valve (ECOM, Ventil C, Czech Republic) and continuous flow cells adapted for screen printed electrodes,25 was constructed for FIA measurements. 46-Range Digital Multimeter (Radioshack, China) with PC interface was used for potentiometric measurements in case of FIA and response time measurements.
2.6. Procedures
2.6.1. Sensor construction. The potentiometric bielectrode strips were printed on a ceramic support (dimensions 5 × 35 mm) using silver- and graphite-based inks for reference and working electrodes, respectively.26 The ion-sensing cocktail containing 1.0 mg β-CD (V), 1.0 mg Hy, 360 mg f-PNPE and 240 mg PVC dissolved in 6 mL tetrahydrofuran was printed on the surface of the graphite/PVC conducting track and left to dry at 50 °C for 24 h. In alternative electrode matrix, 20 mg of MWCNTs were added to the aforementioned matrix, sonicated for 30 min and typically printed on the conducting carbon track. For the nanocomposite based matrices, 2.0 mg of FMWCNTs/β-CD, 1.0 mg Hy, 360 mg f-PNPE and 240 mg PVC were dissolved in 6 mL THF and sonicated for 30 min before printing of the sensing membrane. All the fabricated electrodes were directly used in potentiometric measurements after preconditioning in 10−3 mol L−1 oxicam solutions for 10 min.
2.6.2. Sensor calibration. For batch measurements, the developed sensors were calibrated by immersing the bielectrode strip in different oxicam solutions covering the concentration range from 10−7 to 10−2 mol L−1 at 25 °C. The potential readings were recorded and plotted against drug concentration in logarithmic scale.Operating FIA measurements, 50 μL of freshly prepared drug solutions covering the range from 10−6 to 10−2 mol L−1, were injected in the flowing 5 × 10−2 mol L−1 NaOH stream with flow rate of 12.6 mL min−1.25 The corresponding peak heights were recorded and used to draw the calibration graphs.
2.6.3. Potentiometric determination of oxicams in pharmaceutical preparations and biological samples. Different OXM derivatives were potentiometrically determined using the developed sensors by potentiometric titration and FIA measurements. Aliquots of the sample solutions containing 2.0 to 11.0 mg of oxicam derivatives were titrated against standardized Hy solution27 using the fabricated OXM bielectrode strip as indicator electrode. Potential readings were plotted against the titrant volume to estimate the end point.Under FIA conditions, 50 μL of the sample solutions were injected in the flowing NaOH stream and the peak heights were compared to those obtained from injecting standard solutions of the same concentration. The obtained recoveries were compared with the official methods for each drug.
3. Results and discussion
Chemically modified electrodes (CMEs), were suggested for improving sensitivity and selectivity of the electroanalytical methods through using of species capable of molecular recognition.28 Different macrocyclic molecules; such as crown ethers, calixarenes, phthalocyanins, porphyrins and cyclodextrins (CDs), have been proposed as sensing ionophores, where CDs were by far the most commonly used.29 Cyclodextrins are distinguished by the number of glucopyranose units that form the troncoconic structure, which can be 6, 7 or 8, and are referred to as α-, β- and γ-cyclodextrin.30 Hydroxyl groups of the glucopyranose subunits of the CD molecule are orientated to the exterior of the molecule; therefore the CD exterior is hydrophilic, whereas the central cavity, lined with skeletal carbon and ether oxygen atoms of the glucopyranose units, is relatively hydrophobic. The lipophilic cavity of a cyclodextrin molecule provides a micro environment which an appropriately sized nonpolar drug molecule, or more often nonpolar parts, can enter to form an inclusion complex.31
3.1. Characterization of LXM/β-CDs inclusion complexes
Phase solubility studies, on different oxicam and cyclodextrin derivatives, were performed according to Higuchi and Connors32 and the apparent stability constant (K1:1) was calculated.33 Stability constant of LXM-β-CD inclusion complex was higher than that with either γ-CD or α-CD (values were 10.959, 6.400 and 3.700 M−1 for β-CD, γ-CD and α-CD, respectively). Considering the size of α-, β- and γ-CDs, α has the smallest cavity (4.7 Å) which may be unable to include the lornoxicam molecule with the cavity, while β- and γ-CDs have larger cavities that enables them to include the LXM inside their cavities.34,35
The stability constants for different OXM derivatives with β-CDs (III–V), assuming a 1
:
1 stoichiometry are listed in Table 1. Phase solubility experiments at 25 °C showed an increase of the drug solubility in a linear form as a function of CDs concentration indicating formation of a soluble complex of the AL type. Stability constants of different oxicam derivatives were of lower values compared to finding by other investigators36–38 which may be attributed to the high pH value of the complexation medium used (5 × 10−2 mol L−1 NaOH).36
Table 1 Stability constants of different oxicam and β-CD inclusion complexes
Oxicam |
β-CD (III) |
β-CD (IV) |
β-CD (V) |
PXM |
2.897 |
4.249 |
5.904 |
TXM |
2.432 |
4.035 |
4.883 |
MXM |
5.351 |
6.807 |
7.326 |
LXM |
10.959 |
12.095 |
20.118 |
Moreover, the apparent stability constants of the four investigated oxicam derivatives with β-CDs (III–V) lie in the following order: 2,3,6-tri-O-methyl β-cyclodextrin (V) > 2,6-di-O-methyl β-cyclodextrin (IV) > native β-cyclodextrin (V). All the studied β-CD derivatives have the same cavity diameter; however, the parent β-CD has height (8 Å), therefore part of the drug could still be outside the nanocage (vide infra). Upon methylation, the height of β-CD increased (about 11 Å), which enhance more penetration of the drug inside the cyclodextrin cavity. Furthermore, the hydrophobic part provided by the methyl groups increases the hydrophobicity of the cavity.39 Such results come in accordance with other investigators where methyl β-CD and hydroxypropyl β-CD showed higher values of stability constant compared to native β-CD.40,41
In addition, different oxicam derivatives showed variant stability constants with cyclodextrin which may be related to their structures and the part of the drug molecule which enter the CD cavity. Molecular modelling studies42 show that the minimum energy configuration gives favourable interaction energy between the β-CDs and the oxicam molecule when the conjugated rings of the drugs are inside the hydrophobic bucket-like cavity of β-CDs and the third ring is exposed to the solvent.
3.2. Optimal sensor matrices compositions
3.2.1. Effect of sensing material. Various CDs derivatives (I–V) were tested as molecular recognition elements for oxicam potentiometric sensors. Lower Nernstian responses were obtained via incorporation of α- or γ-CDs as sensing ionophore, while electrodes incorporated with different β-CDs ionophores (III–V) showed reasonable Nernstian responses (Fig. 1a). Sensors incorporated with methylated β-CD (V) showed the superior performance with Nernstian slope value of 52.0 ± 1.6 mV decade−1 in the concentration range from 10−5 to 10−2 mol L−1 LXM. Such variation in sensitivity can be explained on the basis of the stability constants of LXM/β-CDs inclusion complexes and fitting of LXM in the CD cavities (see Section 3.1). Moreover, β-CD (V) contents within the electrode matrices were varied from 0.5 to 5.0 mg and incorporation of 1.0 mg of the aforementioned ionophore was sufficient to get the proper performance.
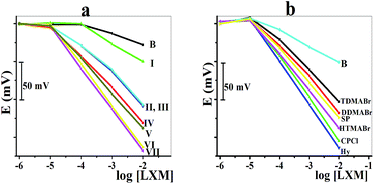 |
| Fig. 1 Effect of sensing ionophores and ionic sites nature on lornoxicam electrode performance. | |
3.2.2. Effect of ionic sites. It is well established that lipophilic ionic additives promote the interfacial ion-exchange kinetics and decrease the membrane resistance by providing mobile ionic sites in the membrane matrix.43,44 Cyclodextrins behave as neutral carrier ionophores and therefore addition of cataionic sites to their electrode matrices is required. From different ionic sites tested, Hy exhibited the best performance compared with CPC, DDMAB, HTMAB, TDMAB or Sp, which may be attributed to the difference in their lipophilicities (Fig. 1b). Furthermore, the content of Hy was changed from 0.0 to 5.0 mg and addition of 1.0 mg was the selected.
3.2.3. Effect of membrane plasticizer. Nature of the membrane plasticizer has crucial roles on the electrode performance through its affect on the mobility of ionophore molecules and the state of the formed inclusion complexes between the sensing ionophore and analyte.45,46 In the present work, five membrane plasticizers with different dielectrical contestants, were tested namely; f-PNPE, o-NPOE, TCP, DOS and DOP (ε = 50, 24, 17.6, 5.2 and 4.7, respectively). Application of less polar plasticizers lowered the Nernstian responses compared with reasonable sensitivity observed for electrodes containing high polar plasticizer. Sensors fabricated using f-PNPE showed the highest slope value (57.9 ± 1.2 mV decade−1) in the concentration range from 10−6 to 10−2 mol L−1 LXM.
3.2.4. Nanomaterial and nanocomposite based electrodes. It has been shown experimentally that the introduction of CNTs into a polymer matrix improves the electric conductivity which in turn improved the dynamic working range and response time.19,47,48 For sake of more sensitivity, different quantities of MWCNTs (ranging from 0 to 50 mg) were incorporated into the electrode matrix containing β-CD (V) as sensing material. Improved sensitivity and potential stability were achieved via addition of 20 mg MWCNTs (slope value was 58.1 ± 0.8 mV decade−1).Moreover, the FMWCNT-β-CD composite was used as a sensing ionophore instead of free β-CD (V) or MWCNTs. The effect of the nanocomposite contents within the electrode matrix was tested in the concentration range from 0.5 to 10 mg and addition of 2.0 mg was sufficient to get Nernstian slope 60.5 ± 0.6 mV decade−1 in the concentration range from 10−6 to 10−2 mol L−1 of LXM.
3.3. Sensor performances
The potentiometric response characteristics of the developed sensors towards different oxicam derivatives were evaluated according to the IUPAC recommendation49 (Table 2 and Fig. 2a). Data recommended application of FMWCNTs/β-CD nanocomposite as sensing material compared with those incorporated with free β-CD (V) or carbon nanotube which can be explained on the basis of the synergistic effect between MWCNTs and β-CD within the composite structure.16 From tested oxicam derivatives, sensors showed higher sensitivity towards lornoxicam which may be explained on the basis of the stability constants of β-CD (V) with oxicam derivatives (Table 1, Fig. 2b).
Table 2 Analytical performancesa of different oxicam screen printed sensors
Sensors |
LXM |
MXM |
TXM |
PXM |
Free β-CD |
CNT-β-CD |
FMWCNT/β-CD |
FMWCNT/β-CD |
FMWCNT/β-CD |
FMWCNT/β-CD |
Results are average of five different calibrations. |
Concentration range (mol L−1) |
10−6 to 10−2 |
10−6 to 10−2 |
10−6 to 10−2 |
10−6 to 10−2 |
10−5 to 10−2 |
10−5 to 10−2 |
Slope (mV decade−1)a |
57.9 ± 1.2 |
58.1 ± 0.8 |
60.5 ± 0.6 |
54.5 ± 0.9 |
53.5 ± 2.4 |
52.6 ± 3.2 |
R |
0.99973 |
0.99973 |
0.99987 |
0.9996 |
0.998 |
0.9964 |
Limit of detection (LOD, mol L−1) |
1.0 × 10−6 |
1.0 × 10−6 |
6.0 × 10−7 |
1.0 × 10−6 |
5.0 × 10−6 |
4.0 × 10−6 |
Limit of quantification (LOQ mol L−1) |
1.0 × 10−5 |
7.0 × 10−6 |
3.0 × 10−6 |
1.0 × 10−5 |
5.0 × 10−5 |
4.0 × 10−5 |
Response time (s) |
4 |
3 |
2 |
2 |
2 |
2 |
Lifetime (week) |
12 |
12 |
16 |
16 |
16 |
16 |
Reproducibility (mV per day) |
±0.6 |
±0.5 |
±0.3 |
±0.8 |
±0.8 |
±0.7 |
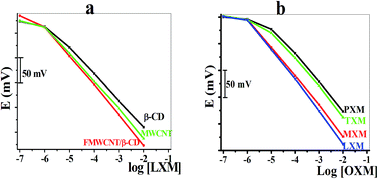 |
| Fig. 2 Electrochemical performances of oxicam screen printed sensors. | |
Screen printing technology offers the advantages of high fabrication reproducibility. The average Nernstian slope values for ten printed electrodes within the same batch were 59.0 ± 0.5 mV decade−1 with standard potential of −246.6 ± 1.8 mV.
Operational lifetimes of the fabricated electrodes were tested by performing day-to-day calibration with storage of electrodes at 4 °C when not used. SPEs showed useful shelf lifetime for 16 weeks during which the Nernstian slopes did not change significantly (±1 mV decade−1). Shorter lifetimes (12 weeks) were noticed in case of sensor incorporated with free ionophores due to leakage of the sensing material to the measuring solution.
One disturbing drawbacks of solid contact electrodes was the potential drift and the poor adhesion of the sensing membrane to the metal substrate50 due to penetration of water and ions through the sensing membrane with formation of undefined layer between sensing membrane and conductor.
In the proposed sensor fabrication protocol, the sensing layer contain the same polymer matrix of the conducting track, and co-polymerization of both PVC matrices (sensitive membrane and conducting track) during electrode fabrication will hinder formation of such internal water layer and improve the electrode potential stability. Thus, the fabricated electrodes can directly be used in measurements after preconditioning in 10−3 mol L−1 OXM solutions for 10 min. In addition, incorporation of MWCNTs enhances the hydrophobicity of the membrane, which contributes to the more stable potential signal by elimination of undesirable water layer at the interface.51 Even though the use of the SPEs allows a single use of the sensor, it can be reliably applied up to ten times without significant losses of the sensitivity.
The dynamic response times of the fabricated sensors were tested by measuring the time required to achieve a steady state potential (within ±1 mV) after sudden increase in the oxicam concentration from 1 × 10−6 to 1 × 10−3 mol L−1. Sensors incorporated with FMWCNTs/β-CD nanocomposite showed fast response time (about 2 s) compared with 3 and 4 s for those contain free ionophore or free MWCNTs.
The influence of pH on the electrode response was checked by recording the electrode potential readings for oxicam solutions containing 10−4 to 10−2 mol L−1 at different pH values (pH 6–12). The electrode responses were pH independent in the range from 8–11. The better performances at higher pH value may be explained on the bases of the stability constants of OXM tautomeric forms with β-CD. Oxicams usually presents in neutral, zwitterionic, anionic (deprotonated) and cationic (protonated) forms. The optimized structures of the inclusion complexes reveal an overall affinity ranking for the OXM guest molecule in the following order: deprotonated form > enolic form > zwitterionic form > protonated form.36,52
In pharmaceutical analysis, it is important to test the selectivity of the method towards the excipients which are usually added to the pharmaceutical preparations, such as glucose, starch, talc, lactose, sucrose. Selectivity of the prepared potentiometric sensors towards different species was tested applying Matched Potential Method (MPM).53 Results revealed high selectivity toward LXM in the presence of other interferents, additives and fillers introduced in pharmaceutical formulations (Table 3). Some metal cations such as Fe(III), Cr(III), Cd(II), Cu(II) and U which form organometallic complexes with oxicams54 are expected to interfere, but they are not present in the aforementioned drug formulations.
Table 3 Potentiometric selectivity coefficients of lornoxicam – screen printed sensors under batch and FIA conditionsa
Interferent |
−log KA,B |
Batch |
FIA |
|
Batch |
Average of five measurements. |
Li+ |
3.20 |
3.30 |
Maltose |
3.60 |
NH4+ |
3.40 |
3.50 |
Starch |
3.80 |
Ca2+ |
2.80 |
3.00 |
Sucrose |
3.10 |
Mg2+ |
3.50 |
3.70 |
Glucose |
2.00 |
Ni2+ |
2.10 |
2.45 |
Fructose |
2.20 |
Co2+ |
1.80 |
2.00 |
Glycine |
2.40 |
Phosphate |
3.80 |
4.00 |
Caffeine |
3.30 |
Citrate |
2.95 |
3.10 |
Cysteine |
2.80 |
3.4. Analytical applications
3.4.1. Potentiometric titration. Different oxicam derivatives were titrated against standardized hyamine solution27 using sensors incorporated with FMWCNT/β-CD composite (Fig. 3). It can be noticed that the best titration curve was obtained in the case of LXM compared with other oxicam derivatives (the total potential change values were 220, 204, 172 and 155 mV for LXM, MXM, TXM and PXM, respectively). Under the optimum conditions, titration curves were symmetrical with well-defined potential jumps (ΔE ranged from 120 to 220 mV) allowing the determination of less 0.8 mg LXM.
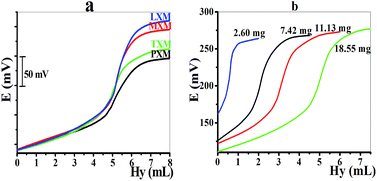 |
| Fig. 3 Potentiometric titration of, (a) 5 mL 10−2 mol L−1 of different OXM derivatives, and (b) different LXM concentrations with 10−2 mol L−1 Hy using FMWCNT/β-CD based screen printed sensors as indicator electrode. | |
3.4.2. Electrode response under FIA conditions. One of the promising advantages of ISEs is their incorporation in flow injection systems for sake of automation and high sampling frequency.55 Fig. 4 showed flow injection peaks from the LXM electrode when 50 μL of LXM solutions covering the concentrations range from 10−6 to 10−2 mol L−1 were injected in 5 × 10−2 mol L−1 NaOH flowing stream (12.6 mL min−1). Calibration graphs were linear in the concentration range from 10−6 to 10−2 mol L−1 with Nernstian slopes of 61.2 ± 0.7 mV decade−1. Reproducibility was evaluated from repeated 10 injections of 50 μL LXM solution (10−3 mol L−1); the average peak heights were found to be −70.2 ± 1.2 mV. The fabricated electrodes showed fast response time (2 s) and stable potential readings which improve the residence time (ranged between 10 and 25 s) and the sampling output (100 sample h−1).
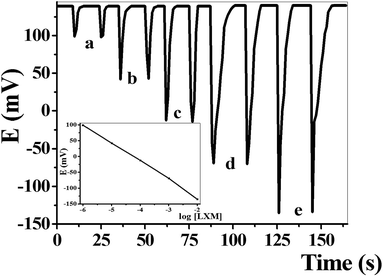 |
| Fig. 4 FIA potentiometric determination of lornoxicam using FMWCNT/β-CD-SPE: (a) 1 × 10−6, (b) 1 × 10−5, (c) 1 × 10−4, (d) 1 × 10−3, and (e) 1 × 10−2 mol L−1 via injection of 50 μL sample at flow rate 12.6 mL min−1. | |
3.4.3. Potentiometric determination of oxicam derivatives in pharmaceutical preparations and biological fluids. The obtained satisfactory sensitivity and selectivity of the fabricated sensors made the proposed methods suitable for the routine quality control analysis of different oxicam derivatives in their pharmaceutical formulations and biological fluids under FIA and potentiometric titration modes. Results (Table 4) clearly indicated satisfactory agreement between the drug contents in pharmaceutical samples determined by the developed sensor and official methods.21–24 The time required for sample analysis was short in case of FIA (about 2 min) with the advantage of accuracy and automation feasibility. Lornoxicam is absorbed from gastrointestinal tract and is characterized by a rapid rate. The peak blood concentration is reached after approximately 1–2 h.56 Lornoxicam is found in the plasma in unchanged form and as its metabolite. 5-Hydroxylation is the main metabolic pathway, which accounts for up to 95% of total intrinsic lornoxicam clearance. Preliminary tests showed that hydroxylation of the pyridyl ring of lornoxicam molecules does not affect the complexation with cyclodextrin.56
Table 4 Potentiometric determination of oxicam derivatives their pharmaceutical preparations and biological fluids
Sample |
Potentiometric titration |
FIA |
Official (mg) |
Proposed (mg) |
Recoverya (%) |
RSD |
Official (μg) |
Proposed (mg) |
Recoverya (%) |
RSD |
Mean recovery and relative standard deviations of five replicate of the same concentration. Average recoveries were calculated according to the official method for each drug.21–24 |
Zeficam |
2.10 |
2.07 |
98.6 |
1.5 |
0.74 |
0.73 |
99.1 |
1.1 |
6.30 |
6.25 |
99.2 |
1.4 |
7.44 |
7.43 |
99.9 |
1.3 |
10.50 |
10.46 |
99.6 |
1.7 |
74.36 |
75.48 |
101.5 |
1.4 |
Spiked urine |
2.10 |
2.05 |
97.6 |
2.0 |
0.74 |
0.73 |
98.2 |
1.9 |
6.30 |
6.21 |
98.5 |
1.9 |
7.44 |
7.34 |
98.7 |
1.6 |
Spiked plasma |
2.10 |
2.06 |
98.1 |
2.2 |
0.74 |
0.72 |
97.9 |
1.7 |
6.30 |
6.22 |
98.8 |
2.0 |
7.44 |
7.33 |
98.6 |
1.4 |
Moxen |
2.11 |
2.07 |
98.0 |
1.2 |
0.70 |
0.69 |
98.7 |
1.0 |
6.33 |
6.25 |
98.7 |
1.6 |
7.03 |
6.97 |
99.1 |
1.2 |
10.55 |
10.57 |
100.2 |
1.7 |
70.28 |
70.06 |
99.7 |
1.3 |
Tenoxil |
3.20 |
3.17 |
99.0 |
1.3 |
6.74 |
6.68 |
99.1 |
1.0 |
6.40 |
6.39 |
99.8 |
1.4 |
67.40 |
66.66 |
98.9 |
1.3 |
11.00 |
10.91 |
99.2 |
1.6 |
|
|
|
|
Dispercam |
3.30 |
3.24 |
98.2 |
1.7 |
6.62 |
6.53 |
98.7 |
1.0 |
6.60 |
6.53 |
99.0 |
1.8 |
66.20 |
65.87 |
99.5 |
1.1 |
9.90 |
9.86 |
99.6 |
1.5 |
|
|
|
|
3.5. Method validation
Validation of electroanalytical methods is used to confirm the applicability of the proposed procedure for a specific test like other analytical methods.57 Accuracy, precision, linearity, specificity, limits of detection (LOD) and quantification (LOQ) were achieved using a standard LXM stock solution.
The accuracy of the proposed method using sensors incorporated with FMWCNT/β-CD composite was investigated by the determination of LXM in spiked samples prepared from serial concentrations of LXM reference standards. The results summarized in Table 5 show high accuracy of the proposed method, as indicated by the percentage recovery values. The statistical analysis of the results using Student's t-test and F-test showed no significant differences between them regarding accuracy and precision (Table 4).
Table 5 Evaluation of accuracy and precision of lornoxicam – screen printed sensors
Sample |
Officiala (mg) |
Intra-day |
Inter-day |
Proposed (mg) |
Recovery (%) |
SD |
RSD |
Proposed (mg) |
Recovery |
SD |
RSD |
Mean recovery and relative standard deviations of five replicate of the same concentration. Average recoveries were calculated according to the official method for each drug.24 |
LXM |
2.10 |
2.07 |
98.6 |
0.09 |
1.52 |
2.02 |
96.4 |
0.12 |
1.65 |
6.30 |
6.25 |
99.2 |
0.06 |
1.25 |
6.19 |
98.2 |
0.0.9 |
1.40 |
10.50 |
10.46 |
99.6 |
0.04 |
1.00 |
10.66 |
101.5 |
0.08 |
1.35 |
Zeficam |
2.11 |
2.07 |
98.0 |
0.10 |
1.42 |
2.08 |
98.7 |
0.15 |
1.78 |
6.33 |
6.25 |
98.7 |
0.08 |
1.36 |
6.27 |
99.1 |
0.11 |
1.65 |
10.55 |
10.57 |
100.2 |
0.07 |
1.17 |
10.51 |
99.7 |
0.09 |
1.33 |
Intra-day and inter-day precisions were assessed using three concentrations and five replicates of each concentration. The relative standard deviations were found to be very small indicating reasonable repeatability and reproducibility of the proposed method as shown in Table 5.
The specificity of the method was examined by observing the interference caused by the common excipients of the pharmaceutical formulation. It was found that these compounds did not interfere with the results of the proposed method as shown in Table 3.
Linear relationship was present between the electrode potential (mV) and the log[LXM]. The regression data, correlation coefficients (r) and other statistical parameters are presented in Table 2. The values of LOD that are presented in Table 2 indicate that the sensors under investigation are highly sensitive, selective and can be applied in determination of small amounts of LXM. The LOQ was determined by establishing the least concentration that can be measured according to ICHQ2 (R1) recommendations,58 below which the calibration range is non-linear, and was found to be 5.0 × 10−6 mol L−1.
4. Conclusions
The present work describes the fabrication of novel disposable screen printed sensor based FMWCNT/β-CD for potentiometric determination of different anti-inflammatory agents including lornoxicam, meloxicam, piroxicam and tenoxicam. Sensors showed Nernstian compliance in the concentration range from 10−6 to 10−2 mol L−1 with fast response time (2 s) and long operational lifetime (16 weeks). Improved sensitivity and selectivity were achieved by incorporation of carbon nanotubes/β-CD composite and carbon nanotube within the electrode matrix. The fabricated sensors showed better performance compared with previously published oxicam sensors14–16 in term of sensitivity, operational lifetime and the simple fabrication protocol for mass production. The proposed method is suitable for routine analysis of different oxicam derivatives in pure and four different dosage forms with average recoveries comparable to the official methods. These results may be the base for further research leading to improvement of the analytical parameters for preparation of simple drug potentiometric sensors with the possibility of commercialization of such disposable sensors.
Acknowledgements
Authors acknowledge the support from the project 9030104 NRC.
Notes and references
- J. G. Lombardino and J. A. Lowe, Nat. Rev. Drug Discovery, 2004, 3, 853 CrossRef CAS PubMed.
- J. A. Balfour, A. Fitton and L. B. Barradell, Drugs, 1996, 51, 639 CrossRef CAS PubMed.
- M. Starek and J. Krzek, Talanta, 2009, 77, 925 CrossRef CAS PubMed.
- A. A. Gouda, M. I. El-Sayed, A. S. Amin and R. El-Sheikh, Arabian J. Chem., 2013, 6, 145 CrossRef CAS PubMed.
- A. S. Jagtap, S. S. Yadav and J. R. Rao, Pharm. Globale, 2011, 2, 1 Search PubMed.
- Y. H. Kim, H. Y. Ji, E. S. Park, S. W. Chae and H. S. Lee, Arch. Pharmacal Res., 2007, 30, 905 CrossRef CAS.
- P. Prajesh, V. Vipul, R. Deepak, P. Harshad, K. Jasmin and B. Dharmendra, J. Pharm. Anal., 2012, 2, 306 CrossRef PubMed.
- M. M. Ghoneim, A. M. Beltagi and A. Radi, Anal. Sci., 2002, 18, 183 CrossRef CAS.
- Y. Wang, H. Xu, J. Zhang and G. Li, Sensors, 2008, 8, 2043 CrossRef CAS PubMed.
- D. W. Kimmel, G. LeBlanc, M. E. Meschievitz and D. E. Cliffel, Anal. Chem., 2012, 84, 685 CrossRef CAS PubMed.
- S. A. Ozkan, B. Uslu and H. Y. Aboul-Enein, Crit. Rev. Anal. Chem., 2003, 33, 155 CrossRef.
- V. V. Cosofret and R. P. Buck, Pharmaceutical Applications of Membrane Sensors, CRC Press, Boca Raton, FL, 1992 Search PubMed.
- K. Vytras, Advanced Instrumental Methods of Chemical Analysis, Ellis Horwood, Chichester, UK, 1st edn, 1993 Search PubMed.
- S. Khalil, N. Borham and M. A. El-Ries, Anal. Chim. Acta, 2000, 414, 215 CrossRef CAS.
- Z. Kormosh, I. Hunka, Y. Bazel and O. Matviychuk, Mater. Sci. Eng., C, 2010, 30, 997 CrossRef CAS PubMed.
- E. Khaled, M. S. Kamel, H. N. A. Hassan, A. A. Haroun, A. M. Youssef and H. Y. Aboul-Enein, Talanta, 2012, 97, 96 CrossRef CAS PubMed.
- O. D. Renedo, M. A. Alonso-Lomillo and M. J. Martinez, Talanta, 2007, 73, 202 CrossRef CAS PubMed.
- M. Li, Y. Li, D. Li and Y. Long, Anal. Chim. Acta, 2012, 734, 31 CrossRef CAS PubMed.
- Z. Spitalsky, D. Tasis, K. Papagelis and C. Galiotis, Prog. Polym. Sci., 2010, 35, 357 CrossRef CAS PubMed.
- Q. Yu, Y. Liu, X. Liu, X. Zeng, S. Luo and W. Wei, Electroanalysis, 2010, 22, 1012 CrossRef CAS.
- European Pharmacopoeia, 6th edn (Suppl. 6.2), Council of Europe, Strasbourg, France, 2007.
- United States Pharmacopeia, 24th Review, The National Formulary, 19th Review, The United States Pharmacopeial convention, Rockville, MD, 2000.
- British Pharmacopeia, Stationary Office, London, 7th edn, 2014, vol. II.
- P. Prajapati, V. Vaghela, D. Rawtani, H. Patel, J. Kubavat and D. Baraiya, J. Pharm. Anal., 2012, 2, 306 CrossRef CAS PubMed.
- E. Khaled, H. N. A. Hassan, G. G. Mohamed and A. A. Seleim, Drug Test. Anal., 2010, 2, 424 CrossRef CAS PubMed.
- E. Khaled, M. S. Kamel, H. N. A. Hassan, H. Abdel-Gawad and H. Y. Aboul-Enein, Talanta, 2013, 119, 467 CrossRef PubMed.
- K. Vytras, Ion-Sel. Electrode Rev., 1985, 7, 77 CAS.
- J. Zen, A. S. Kumar and D. Tasi, Electroanalysis, 2003, 15, 1073 CrossRef CAS.
- T. Ogoshi and A. Harada, Sensors, 2008, 8, 4961 CrossRef CAS PubMed.
- G. Astray, C. Gonzalez-Barreiro, J. C. Mejuto, R. Rial-Otero and J. Simal-Gandara, Food Hydrocolloids, 2009, 23, 1631 CrossRef CAS PubMed.
- K. H. Fromming and J. Szejtli, Cyclodextrins in Pharmacy, Kluwer Academic Publishers, Dordrecht, 1994 Search PubMed.
- T. Higuchi and K. Connors, Adv. Anal. Chem. Instrum., 1995, 4, 117 Search PubMed.
- T. Loftsson, M. Masson and M. E. Brewater, J. Pharm. Sci., 2004, 93, 1091 CrossRef CAS PubMed.
- A. Rawashdeh, S. Mizyed, S. Mahmoud and D. Marji, Spectrochim. Acta, Part A, 2008, 71, 562 CrossRef PubMed.
- N. B. Naidu, K. P. R. Chowdary, K. V. R. Murthy, V. Satyanarayana, A. R. Hayman and G. Becket, J. Pharm. Biomed. Anal., 2004, 35, 75 CrossRef CAS PubMed.
- M. E. Dalmora and A. G. Oliveira, Int. J. Pharm., 1999, 184, 157 CrossRef CAS.
- S. Senel, O. Cakoglu, M. Sumu, D. Duchene and A. Hincal, J. Inclusion Phenom. Macrocyclic Chem., 1992, 14, 171 CrossRef CAS.
- N. Yadav, G. Chabra and K. Pathak, Int. J. Pharm. Pharm. Sci., 2012, 4, 395 CAS.
- C. J. Easton and S. F. Lincoln, Modified Cyclodextrins, Scaffolds and Templates for Supramolecular Chemistry, Imperial College Press, London, 1999 Search PubMed.
- E. Larrucea, A. Arellano, S. Santoyo and P. Ygartua, Drug Dev. Ind. Pharm., 2002, 28, 245 CrossRef CAS PubMed.
- H. O. Ammar, M. Ghorab, A. A. Mahmoud, T. S. Makram and S. H. Noshi, J. Inclusion Phenom. Macrocyclic Chem., 2012, 73, 161 CrossRef CAS.
- R. Banerjee, H. Chakraborty and M. Sarkar, Biopolymers, 2004, 75, 355 CrossRef CAS PubMed.
- E. Bakker, P. Buhlmann and E. Pretsch, Electroanalysis, 1999, 11, 915 CrossRef CAS.
- M. Telting-Diaz and E. Bakker, Anal. Chem., 2001, 73, 5582 CrossRef CAS.
- E. Bakker, P. Buhlmann and E. Pretsch, Electroanalysis, 1999, 11, 915 CrossRef CAS.
- W. E. Morf, The Principles of Ion-Selective Electrodes and Membrane Transport, Elsevier, New York, 1981 Search PubMed.
- C. Lai, M. A. Fierke, A. Stein and P. Buhlmann, Anal. Chem., 2007, 79, 4621 CrossRef CAS PubMed.
- J. Zhu, Y. Qin and Y. Zhang, Electrochem. Commun., 2009, 11, 1684 CrossRef CAS PubMed.
- R. P. Buck and E. Lindner, Pure Appl. Chem., 1994, 66, 2527 CrossRef CAS.
- B. P. Nikolskii and E. A. Materova, Ion-Sel. Electrode Rev., 1985, 7, 3 CAS.
- J. Bobacka, T. Lindfords, M. McCarrick, A. Ivaska and A. Lewenstam, Anal. Chem., 1995, 67, 3819 CrossRef CAS.
- W. Snor, E. Liedl, P. Weiss-Greiler, H. Viernsteinb and P. Wolschann, Int. J. Pharm., 2009, 381, 146 CrossRef CAS PubMed.
- Y. Umezawa, P. Buhlmann, K. Umezawa, K. Tohda and S. Amemiya, Pure Appl. Chem., 2000, 72, 1851 CrossRef CAS.
- H. A. Mohamed, H. M. A. Wadood and O. A. Farghaly, J. Pharm. Biomed. Anal., 2002, 28, 819 CrossRef CAS.
- M. Trojanowicz, M. Szewcznska and M. Wcislo, Electroanalysis, 2003, 15, 347 CrossRef CAS.
- O. M. Ahmed and A. A. Al-Badr, Lornoxicam, Comprehensive Profile, Profiles of Drug Substances, Excipients and Related Methodology, ed. H. G. Brittain, 2011, vol. 36, pp. 205–237 Search PubMed.
- M. Gumustas and A. S. Ozkan, Open Anal. Chem. J., 2011, 5, 1 CrossRef CAS.
- ICH Harmonized Tripartite Guideline: Validation of Analytical Procedures. Text and Methodology, 2005, Q2 (R1).
|
This journal is © The Royal Society of Chemistry 2015 |