DOI:
10.1039/C4RA12340A
(Paper)
RSC Adv., 2015,
5, 153-162
Usnic acid induces apoptosis via an ROS-dependent mitochondrial pathway in human breast cancer cells in vitro and in vivo
Received
14th October 2014
, Accepted 19th November 2014
First published on 20th November 2014
Abstract
Usnic acid (UA), an active dibenzofuran derivative mainly found in lichens, is considered an antineoplastic agent based on its activity against tumor cells. However, the exact molecular mechanism through which UA mediates this activity has yet to be elucidated. Here, we have shown that UA selectively inhibited the viability of human breast cancer MCF-7 cells in a concentration- and time-dependent manner. UA provoked the generation of reactive oxygen species (ROS), which triggered the mitochondrial/caspase apoptotic pathway in MCF-7 cells. N-Acetylcysteine (NAC) blocked the generation of ROS, which reduced the stimulation of apoptotic mechanisms including activation of c-Jun-N-terminal kinase (JNK), loss of mitochondrial membrane potential (MMP), release of cytochrome-c, and activation of the caspase–cascade. Moreover, UA markedly inhibited tumor growth in a dose-dependent manner in MCF-7 tumor-bearing mice without inducing significant toxicity. Taken together, these findings suggested that UA stimulated apoptosis through an ROS-dependent mitochondrial pathway in MCF-7 cells.
1. Introduction
Breast cancer (BC) is the most common type of cancer and the leading cause of cancer death among women worldwide.1,2 While the disease is currently treated with surgery and radiotherapy, which are frequently supported by adjuvant chemo- or hormone therapy, these treatments are not highly effective at controlling the regional and systemic disease.3,4 One reason is that tumors are often resistant to apoptosis.5 Furthermore, unregulated cellular survival mechanisms result in the promotion of tumor growth and metastasis.6 These alterations in intracellular signaling cascades can render cells resistant to standard therapies.7 Therefore, therapeutic interventions that are designed to attenuate resistance to apoptosis may sensitize tumors to conventional modalities of cancer therapy. To this end, development of novel chemopreventive and/or chemotherapeutic agents and adjuncts may improve the treatment of breast cancer.
Nature products from food occupy a very important position in the area of cancer chemotherapy.8,9 Molecules such as vincristine,10 paclitaxel,11 homoharringtonine,12 etoposide,13 and camptothecin derivatives14 are invaluable natural contributions to modern medicine. However, the quest to discover novel therapeutic nature compounds for cancer treatment and management is a never-ending venture. Lichen can be found only at high elevation and has been used in the long term as a traditional tea and medicine in China.15 Usnic acid (UA, Fig. 1A), a dibenzofuran derivative, is an active compound mainly found in lichens.16 Previous studies showed that UA exhibits several interesting properties such as anti-microbial, anti-viral, anti-inflammatory and anti-proliferative activities.17 Recently, numerous studies have described its anti-tumor activities in several cancer cells including lung, colon, liver, gastric, and ovarian.18–20 Moreover, Bačkorová et al. confirmed that UA also initiated apoptosis in breast cancer cells,21 however, the mechanism of UA-induced apoptosis in breast cancer cells is still not fully clarified.
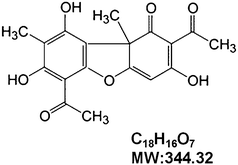 |
| Fig. 1 The chemical structure of UA. | |
Apoptosis is a process of genetically programmed cell death that consists of a cascade of molecular events in stimulated cells. To date, the extrinsic death receptor pathway and the intrinsic mitochondrial pathway are the two main apoptotic pathways.22,23 Reactive oxygen species (ROS) have been shown to induce various biological processes, including apoptosis.24,25 In apoptotic cells, excessive generation of intracellular ROS is a main cause of DNA and protein damage. ROS can directly activate the mitochondrial permeability transition and result in mitochondrial membrane potential (MMP) loss. Loss of MMP is a crucial step in apoptosis, as it subsequently triggers the release of apoptotic factors, such as cytochrome-c and apoptosis-inducing factor (AIF), from the mitochondrion into cytoplasm where these factors induce the propagation of the apoptotic cascade and the execution of cell death.26,27 The mitogen-activated protein kinases (MAPK), a family of serine/threonine kinases, are also involved in apoptosis and cell survival and have been validated as targets for anticancer drugs.28 The c-Jun N-terminal kinase (JNK), a member of the MAPK family, is induced by stress responses and cytokines, and it mediates differentiation and cell death.29,30 It has been demonstrated that MAPK signaling pathways are responsible for ROS-mediated apoptosis.31 ROS may play an important role as second messengers in signal transduction cascades, and they may lead to the activation of MAPKs.32 Based on these, the cytotoxic activity of UA toward human breast cancer cells and the underlying mechanisms involved in this process were investigated.
Here, we report that UA induced apoptosis of human breast cancer MCF-7 cells through the following processes: ROS generation, JNK activation, mitochondrial dysfunction, cytochrome-c release and caspase activation. We also evaluate anti-tumoral activity of UA in a MCF-7 tumor-bearing xenograft mouse model. Both the in vitro and in vivo experiments indicate that UA may serve as a novel agent for the treatment of BC, as it induced excessive ROS production.
2. Materials and methods
2.1 Cell culture and reagents
Human breast cancer cell lines MCF-7, MDA-MB-231, SK-BR-3 and normal human mammary epithelial cell line MCF-10A (ATCC) were cultured in Dulbecco's Modified Eagle's Medium (DMEM), 10% FBS, 100 U mL−1 penicillin, and 100 mg mL−1 streptomycin at 37 °C, 5% CO2. Usnic acid (purity > 98%), N-acetylcysteine (NAC), cyclophosphamide (CTX), 3-(4,5-dimethylthiazol-2-yl)-2,5-diphenyl-tetrazolium bromide (MTT), 3,3′-dihexyloxacarbocyanine iodide (DiOC6) and 2′7′-dichloroflurescein diacetate (DCFH-DA) were obtained from Sigma-Aldrich Inc (St. Louis, MO). DMEM high glucose medium was obtained from GIBCO. Bradford protein Assay Kit, RIPA lysis buffer, annexin V-FITC apoptotic detection kit and caspase-3 activity assay kit were obtained from Beyotime Institute of Biotechnology (Beijing, China). Fetal bovine serum (FBS), SDS, tetramethylethylenediamine, glycine, ammonium, persulfate, acrylamide, Tris, Trizol, agarose, Tween-20 and Proteinase inhibitors were purchased from Beijing Dingguo Biological Technology Co., Ltd (Beijing, China). Antibodies against JNK (rabbit, polyclonal, sc-571), p-JNK (mouse, monoclonal, sc-6254), p-c-Jun (mouse, monoclonal, sc-822), p-ERK (mouse, monoclonal, sc-7383), p-p38 (mouse, monoclonal, sc-7973), Bax (mouse, monoclonal, sc-7480), Bcl-2 (mouse, monoclonal, sc-7382), cytochrome-c (mouse, monoclonal, sc-13561), caspase-3 (rabbit, polyclonal, sc-98785), PARP (rabbit, polyclonal, sc-7150) and GAPDH (rabbit, polyclonal, sc-25778) were purchased from Santa Cruz Biotechnologies, Inc. (Santa Cruz, CA, USA). All the reagents were used without further purification. Deionized water was used in all experiments.
2.2 Cell viability assay
MCF-7, MDA-MB-231, SK-BR-3, and MCF-10A cells were plated into a 96-well culture plate at a density of 5 × 104 cells per mL and incubated overnight at 37 °C. Usnic acid was then diluted to appropriate concentrations and immediately applied to the cells. Concentration- and time-dependent cytotoxicity was assessed by exposing cells to usnic acid at concentrations of 3.125, 6.25, 12.5, 25, 50, and 100 μM for 24 and 48 hours. Viability of the cells was evaluated using the MTT reduction method. The cells were incubated with MTT for four hours, and 200 μL dimethyl sulfoxide was then added to each well to dissolve the dark blue crystal. A wavelength of 492 nm was used to measure cell viability. The results were expressed as the percentage of MTT reduction obtained in the treated cells, assuming that the absorbance of control cells was 100%. All samples were prepared in triplicate.
2.3 Apoptosis assay
Apoptosis was quantified by using annexin V-FITC apoptotic detection kit. Cells were treated with different concentrations of usnic acid for 24 hours, and annexin V staining was assessed per manufacturer's instructions. Briefly, MCF-7 cells (1 × 106) were collected and washed twice with PBS and suspended in 400 μL of binding buffer (containing 5 μL of annexin V-FITC and 10 μL of PI). Thereafter, the samples were incubated in the dark for 10 min at 4 °C and then analyzed using a FACScan flow cytometer (BD Biosciences).
2.4 Caspase-3 activity assay
MCF-7 cells were cultured in 96-well plates, and treated with different concentrations of usnic acid for 24 h. The activity of caspase-3 was determined using a caspase-3 activity kit. Cells were lysed with 80 μL reaction buffer (1% NP-40, 20 mM Tris–HCl pH 7.5, 137 mM NaCl and 10% glycerol) and then incubated with 10 μL caspase-3 substrate Ac-DEVD-pNA at 37 °C overnight. Caspase-3 activity was analyzed by measuring the levels of p-nitroanilide (pNA) cleaved from the substrate Ac-DEVD-pNA with an ELISA reader using a wavelength of 405 nm. Data were analyzed according to the manufacturer's protocol.
2.5 Intracellular ROS detection assay
The production of intracellular reactive oxygen species (ROS) was measured using DCFH-DA. Briefly, MCF-7 cells were seeded in 24-well plates at a density of 1 × 105 cells per well and routinely incubated overnight at 37 °C. After incubation with different concentrations of usnic acid for 24 h, cells were stained with DCFH-DA at a final concentration of 5 mM and incubated at 37 °C for 30 min. ROS production of MCF-7 cells was evaluated by both a laser scanning confocal microscope (Olympus FV1000) and FACScan flow cytometer (BD Biosciences).
2.6 Assessment of mitochondrial membrane potential (MMP)
Mitochondrial membrane potential was monitored using the fluorescent dye DiOC6. For each condition, MCF-7 cells were seeded in 6-well plates at a density of 5 × 105 cells per well and routinely incubated overnight at 37 °C. After incubation with different concentrations of usnic acid for 24 h, cells were incubated for 30 min at 37 °C in 1 mL of 40 nM DiOC6 and subsequently analyzed using a FACScan flow cytometer (BD Biosciences) with excitation and emission settings of 488 and 525 nm, respectively. Ten thousand cells were acquired for each sample. Data were analyzed with FlowJo software. Low levels of DiOC6 uptake corresponded to a loss of MMP.
2.7 Western blot analysis
MCF-7 cells (1 × 106) were plated in a 25 cm2 cell culture flask. After 24 h, the cells were treated with PBS vehicle alone, usnic acid or usnic acid plus NAC for 24 h, and then, the cells were collected and lysed with 0.1 mL of cold RIPA lysis buffer containing 0.02% PMSF. The cell lysate was centrifuged at 12
000g for 30 min at 4 °C. Protein concentrations were determined with a Bradford protein Assay Kit using bovine serum albumin as the standard. The proteins were separated by SDS-PAGE and transferred to a polyvinylidene difluoride (PVDF) membrane. After nonspecific binding sites were blocked with 5% nonfat dry milk in PBS for 60 min, the transferred membrane was incubated at 4 °C overnight with primary antibodies (1
:
500 dilutions). Following repeated washings with TBST, the membranes were incubated with the HRP-conjugated mouse anti-rabbit secondary antibody or HRP-conjugated rabbit anti-mouse secondary antibody (1
:
1000 dilutions) (Santa Cruz Biotechnology, Inc.) for 1 h at room temperature prior to additional washes with TBST. Antibody binding was detected with the enhanced chemiluminescence kit (Amersham Pharmacia Biotech, Amersham, UK). Equal loading was verified using the antibodies against GAPDH. All western blot analyses were repeated three times.
2.8 In vivo tumor xenograft study
To determine the in vivo antitumor activity of UA, MCF-7 cells (2 × 106) were collected in 70 μL PBS and mixed with 70 μL Matrigel Matrix (Becton Dickinson Biosciences). The mixture was injected subcutaneously to one side of the dorsal flank of 6- to 8-week-old female BALB/c nu/nu mice (Vital River Laboratories, Beijing, China). When the tumor reached 100 mm3, mice were randomly divided into five groups (n = 6). The mice in the test group first received intraperitoneal (i.p.) injections of UA (25, 50 or 100 mg kg−1 body weight in sodium chloride injection containing 2.5% Tween-80 in a volume of 200 μL) at 2 day intervals for 18 days. The control group was treated with an equal volume of vehicle. The positive group received i.p. injections of cyclophosphamide (CTX) (25 mg kg−1). The body weights and tumor sizes were accurately recorded once every three days, and the tumor volume was calculated according to the formula: volume [mm3] = π/6 × length × width × width. After 21 days, mice were sacrificed and tumors were removed, weighed, fixed with formaldehyde and embedded in paraffin. Then, 4 μm tissue sections were stained with TUNEL assay. Terminal deoxynucleotidyl transferase-mediated dUTP nick end labeling (TUNEL) assay for apoptosis was conducted according to the manufacturer's instructions (Dead End Fluorometric TUNEL System; Promega). Cells that stained positive for TUNEL were counted at 400× magnification in at least 5 different fields. Experimental animal protocols were approved by the Ethics Committee for the Use of Experimental Animals of Jilin University.
2.9 Statistical analysis
All experiments were completed at least three times unless otherwise indicated. Data are expressed as the means ± standard deviations. The differences between groups were assessed using the SPSS software. Differences with P < 0.05 were considered to be significantly significant.
3. Results
3.1 UA selectively inhibited proliferative activity of breast cancer cell lines
The anti-proliferative activity of UA toward four human breast cell lines, including the cancer cell lines MCF-7, MDA-MB-231, SK-BR-3 and the non-neoplastic cell line MCF-10A, was detected by the MTT assay. As shown in Fig. 2A, estrogen receptor (ER) positive breast cancer MCF-7 cells exhibited time- and concentration-dependent sensitivity to usnic acid, with IC50 values (the concentration of drug inhibiting 50% of cells) of approximately 34.12 ± 1.25 μM at 24 h. In addition, UA also inhibited the growth of other (ER) negative breast cancer cell lines such as MDA-MB-231 and SK-BR-3 cells in a time- and dose-dependent manner (Fig. 2B and C) with IC50 values of 38.41 ± 1.64 μM and 48.07 ± 1.52 μM, respectively after 24 h. However, UA did not affect the proliferation of normal human mammary epithelial cell line MCF-10A at concentrations up to 25 μM (Fig. 2D). Taken together, these results suggest that UA may preferentially kill breast cancer cells over normal mammary epithelial cells, and anti-proliferative effect of UA on breast cancer cells are ER-independent.
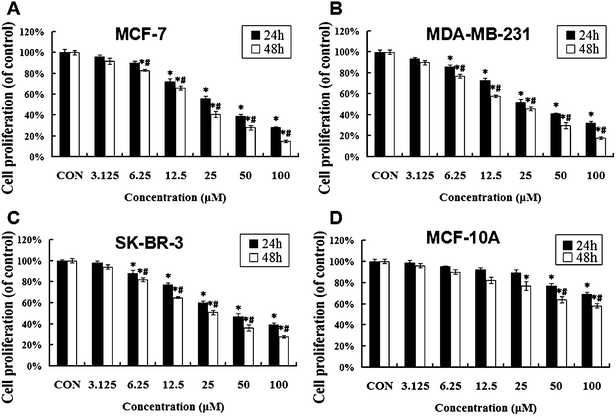 |
| Fig. 2 Effect of UA on the growth of four human breast cell lines. The human breast cancer cell lines MCF-7 (A), MDA-MB-231 (B), and SK-BR-3 (C), and normal human mammary epithelial cell line MCF-10A (D) were treated with different concentrations of UA for 24 and 48 h. The cytotoxicity was analyzed by MTT assay. Each bar represents the mean ± SD of three independent experiments. P* < 0.05 vs. control group. | |
3.2 UA-induced activation of a mitochondrial apoptotic pathway in MCF-7 cells
To investigate whether UA-mediated inhibition of proliferation at 24 h was caused by apoptosis in MCF-7 cells, the number of apoptotic cells was measured using annexin V-FITC/PI staining after treatment with UA for 24 h. As shown in Fig. 3A, there were only small percentages (5.27% ± 0.36%) of cells binding annexin V-FITC in groups of cells that were not treated with UA. On the contrary, the percentages of apoptotic cells were significantly increased from 23.30% ± 1.23% to 45.39% ± 1.82% and 62.56% ± 1.55% in a concentration-dependent manner after treatment with 12.5–50 μM UA. To assess the role of mitochondria in UA-induced cell death, we tested whether UA caused a loss of mitochondrial membrane potential by flow cytometry. As shown in Fig. 3B, UA caused an obvious decrease of mitochondrial membrane potential in MCF-7 cells in a concentration-dependent manner. To evaluate whether UA-induced apoptosis involved the activation of caspase-3, we investigated the caspase-3 activity using a caspase-3 Activity Assay. The results shown in Fig. 3H indicate that UA stimulated caspase-3 activity in a dose-dependent manner at 24 h. Taken together, these results suggested that UA induced apoptosis in MCF-7 cells by stimulating the mitochondrial apoptotic pathway.
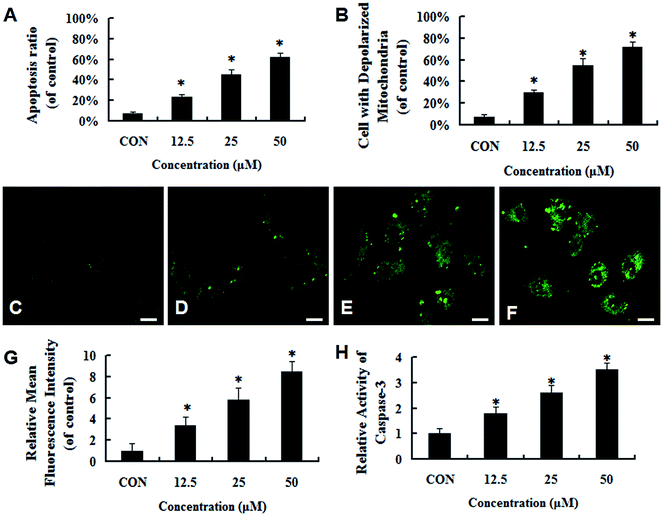 |
| Fig. 3 UA-induced a mitochondrial apoptotic pathway in MCF-7 cells. (A) MCF-7 cells were treated with 12.5–50 μM UA for 24 h, then apoptosis was assessed by annexin V-FITC/PI binding and measured by flow cytometry analysis. (B) Disruption of the mitochondrial membrane potential in cells was monitored by DiOC6 staining and measured by flow cytometry analysis. Cells were treated with UA at concentrations of 0 (C), 12.5 (D), 25 (E), and 50 (F) μM for 24 h, and the intracellular ROS were stained with DCFH-DA and measured by both laser scanning confocal microscopy and flow cytometry (G). The scale bar represents 10 μm. (H) Caspase-3 activation in cells was detected by a caspase-3 activity assay. Each bar represents the mean ± SD of three independent experiments. P* < 0.05 vs. control group. | |
3.3 Generation of ROS triggered UA-induced apoptosis in MCF-7 cells
The generation of intracellular ROS is always associated with mitochondrial membrane potential disruption and cell apoptosis. To investigate the upstream regulatory mechanisms leading to UA-induced mitochondrial dysfunction, we analyzed changes of intracellular ROS in UA-treated and untreated MCF-7 cells by laser scanning confocal microscope and flow cytometry. As shown in Fig. 3C–F, UA induced ROS generation in MCF-7 cells and increased intracellular ROS levels in a concentration-dependent manner. To further validate that ROS was involved in UA-induced apoptosis of MCF-7 cells, the cells were pretreated with 5 mM NAC, a ROS scavenger which had no effect on proliferation of MCF-7 cells. As expected, the presence of NAC significantly reduced the accumulation of intracellular ROS caused by UA (Fig. 4A–E). In addition, NAC inhibited UA-induced apoptosis as evidenced by a reduction in the loss of mitochondrial membrane potential (Fig. 4F), the number of apoptotic cells (Fig. 5C) and the increase in caspase-3 activity (Fig. 5D) after UA treatment. Moreover, NAC abrogated UA-induced loss of MCF-7 cell viability (Fig. 5A and B), indicating that UA exerts cytotoxic effect through ROS generation. Taken together, these results demonstrated that UA-elicited ROS triggered the intrinsic mitochondrial pathway of apoptosis in MCF-7 cells.
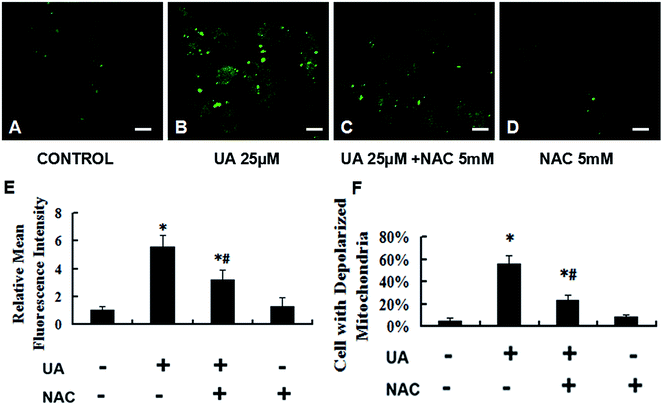 |
| Fig. 4 Role of ROS generation in UA-induced mitochondrial membrane potential dysfunction in MCF-7 cells. MCF-7 cells were treated with 25 μM UA for 24 h in the presence or absence of 5 mM N-acetylcysteine (NAC). Intracellular ROS were stained with DCFH-DA, and ROS levels were measured by both laser scanning confocal microscopy (A–D) and flow cytometry (E). The scale bar represents 10 μm. (F) Mitochondrial membrane potential disruption in cells. Each bar represents the mean ± SD of three independent experiments. P* < 0.05 vs. control group, P# < 0.05 vs. UA group. | |
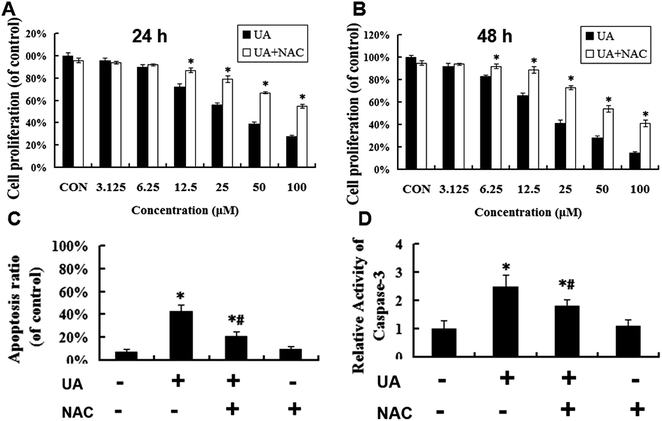 |
| Fig. 5 Role of ROS generation in UA-induced apoptosis in MCF-7 cells. MCF-7 cells were treated with different concentrations of UA for 24 (A) and 48 (B) h in the presence or absence of 5 mM NAC. The cytotoxicity was analyzed by MTT assay. Each bar represents the mean ± SD of three independent experiments. P* < 0.05 vs. UA group. MCF-7 cells were treated with 25 μM UA for 24 h in the presence or absence of 5 mM N-acetylcysteine (NAC). Apoptosis in cells (C) and caspase-3 activation (D) were measured. Each bar represents the mean ± SD of three independent experiments. P* < 0.05 vs. control group, P# < 0.05 vs. UA group. | |
3.4 UA-induced apoptosis is mediated via activation of the ROS-JNK pathway
To investigate the possible role of MAPK pathways in UA-induced apoptosis, the phosphorylation state of JNK, c-Jun, ERK and p38 was evaluated by Western blot analysis. As shown in Fig. 6, UA induced a robust and sustained activation of JNK, c-Jun and ERK, but not p38, suggesting that JNK and ERK may be specifically activated in UA-induced apoptotic pathway. It is known that ROS activates multiple signaling pathways, including the MAPK and Akt signal transduction cascades. Thus, we examined the effect of antioxidants on UA-induced phosphorylation of JNK, c-Jun and ERK. Pretreatment with NAC effectively blocked UA-induced phosphorylation of JNK but not ERK, suggesting that JNK may be a target of ROS, and the ROS-JNK pathway is involved in UA-induced apoptosis.
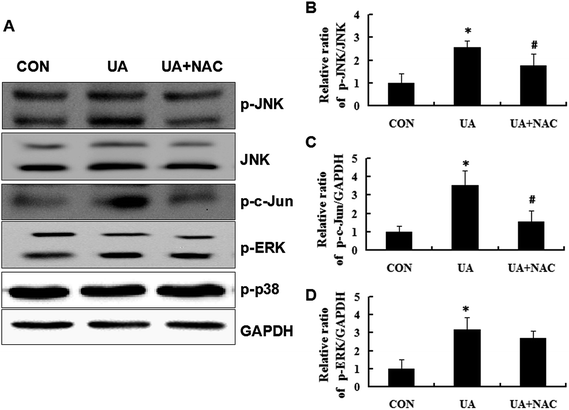 |
| Fig. 6 Roles of MAPK subfamilies in UA-induced apoptosis in MCF-7 cells. (A) Cells were treated with 25 μM UA for 24 h in the presence or absence of 5 mM NAC, then whole-cell lysates were collected and immunoblotted with antibodies against MAPK subfamily proteins, such as JNK, p-JNK (B), p-c-Jun (C), p-ERK (D), and p-p38. GAPDH was used to ensure equal loading of lysates. Each bar represents the mean ± SD of three independent experiments. P* < 0.05 vs. control group, P# < 0.05 vs. UA group. | |
3.5 ROS regulated apoptotic proteins in UA-treated MCF-7 cells
Because Bcl-2 family members play a vital role in the regulation of the mitochondrial apoptotic pathway, the levels of Bax and Bcl-2 were measured by Western blot analysis. As shown in Fig. 7A and B, UA treatment of cells suppressed Bcl-2 expression and upregulated Bax expression compared to control cells, which was most vividly illustrated as a marked increase in Bax/Bcl-2 expression ratio. This effect was inhibited by NAC. It is known that the release of cytochrome-c from mitochondria can activate the caspase cascade. Caspases are a family of proteins related to cysteine proteases and are the effectors of the apoptotic process downstream of both the death receptor and mitochondrial apoptotic pathways. Thus, we investigated the involvement of cytochrome-c, caspase-3 and PARP in UA-induced apoptosis by Western blot analysis. As shown in Fig. 7A, C and D, UA treatment increased the release of cytochrome-c in the cytosolic fraction, activation of caspase-3 and cleavage of PARP. In contrast, NAC reduced UA-induced release of cytochrome-c, activation of caspase-3, and cleavage of PARP. Taken together, these observations suggested that UA-induced ROS generation is an early event that triggers apoptosis through a caspase-dependent mechanism in MCF-7 cells.
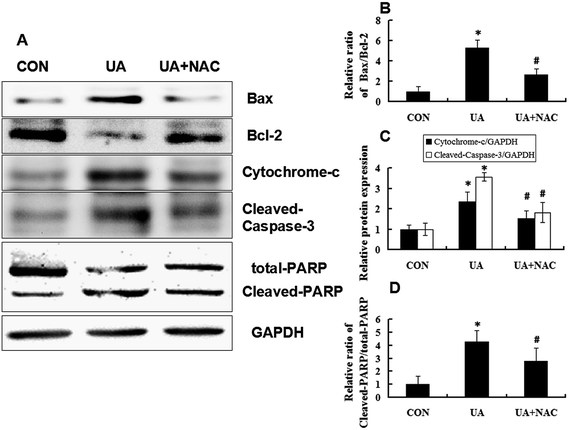 |
| Fig. 7 Effect of UA on proteins involved in the mitochondria- and caspase-dependent apoptotic pathway in MCF-7 cells. (A) Cells were treated with 25 μM UA for 24 h in the presence or absence of 5 mM NAC, then whole-cell lysates were collected and immunoblotted with antibodies against apoptotic proteins, such as Bax, Bcl-2 (B), cytochrome-c, cleaved caspase-3 (C), and cleaved PARP (D). GAPDH was used to ensure equal loading of lysates. Each bar represents the mean ± SD of three independent experiments. P* < 0.05 vs. control group, P# < 0.05 vs. UA group. | |
3.6 UA inhibited MCF-7 xenograft tumor growth by increasing apoptotic cell death
To further evaluate whether UA could inhibit tumor growth in vivo, we examined the effect of UA on the growth of MCF-7 cells in nude mice. As shown in Fig. 8A, UA significantly reduced tumor volume compared with the control group, and the percentage of tumor volume inhibition at day 21 resulting from 25, 50, and 100 mg kg−1 treatments of UA were 21.7, 45.7, and 66.3%, respectively. The percentage of tumor volume inhibition in the cyclophosphamide (CTX) group was 53.1%. The mean tumor weight of mice from UA-treated groups (25, 50 and 100 mg kg−1) were 0.78 ± 0.08, 0.46 ± 0.07 and 0.23 ± 0.06 g, respectively, compared with 0.93 ± 0.09 g for the control group and 0.37 ± 0.05 g for the CTX-treated group (Fig. 8E). In addition, during the experiment no sign of toxicity, which was measured by parallel monitoring of body weight, was observed in UA-treated mice (Fig. 8B), however, the CTX-treated group showed a remarkable decrease in body weight following drug administrations. Furthermore, immunohistochemical analysis by TUNEL showed that UA induced significant apoptosis within the tumor, whereas the tumors from control mice contained minimal signs of apoptosis (Fig. 8C and D). The results revealed obvious features of apoptosis, which strongly suggested that UA-mediated inhibition of tumor growth in breast tumor-bearing mice is closely correlated with the enhanced apoptosis in cancer cells. Taken together, these results revealed that UA was an effective antitumor lead compound with minimal toxic side effects.
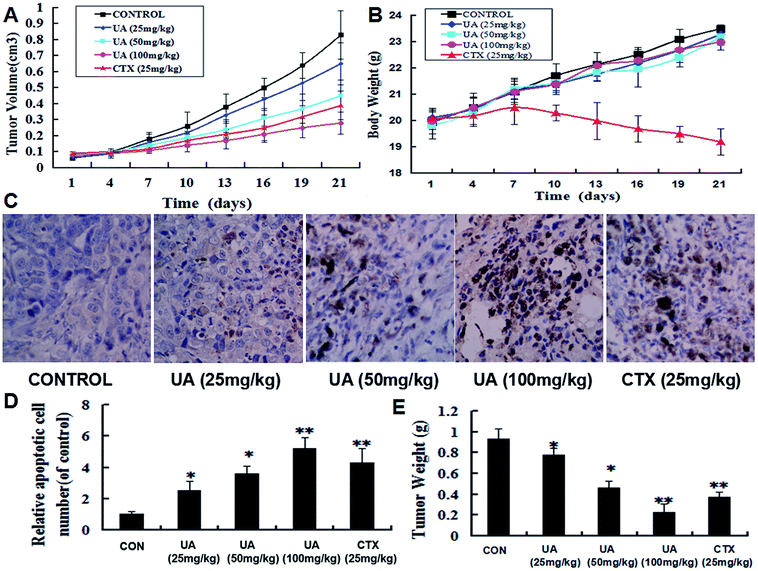 |
| Fig. 8 UA suppressed tumor growth and induced apoptosis in an MCF-7 xenograft model. Nude mice with MCF-7 tumors (n = 6) were treated i.p. with 25, 50, or 100 mg kg−1 UA every 2 days for 21 days. Changes in tumor volume (A) and body weight (B) over time were measured. (C and D) TUNEL assay of tumor. Apoptosis within the tumors was determined by using a TUNEL assay kit. (E) Quantification of tumor weight. P* < 0.05, P** < 0.01 vs. control group. | |
4. Discussion
There is an urgent need to develop innovative ways to treat breast cancer that has become resistant to established therapies. Nature products shave been a main source of highly effective conventional drugs for the treatment of many forms of cancer. While the compounds isolated from fungi often may not serve as drugs, they provide leads for the development of potential novel agents. We sought to identify novel agents by examining natural products with validated anticancer properties. We focused our study on the usnic acid (UA), which is the most common and abundant lichenic secondary metabolite with potential therapeutic application.16 Antitumoral properties have already been reported and UA-enriched extracts are widely used to treat several diseases in non-conventional medicine.18–21 In the present study, we first explored the molecular mechanisms by which UA induced apoptosis in breast cancer cells lines and then examined the involvement of ROS and MAPK in UA-induced apoptosis through the mitochondrial pathway.
We found that UA inhibited the survival of three breast cancer cell lines (MCF-7, MDA-MB-231 and SK-BR-3) and induced apoptosis in a concentration-dependent manner. Moreover, at these concentrations, UA had little effect on MCF-10A cells, non-tumorigenic mammary cells, suggesting a selective antitumoral action of UA. These data enhance the desirability of UA as an anticancer agent because they suggest that UA preferentially kills breast cancer cells, while minimizing damage to normal breast tissue. Accumulating evidence indicates that many chemotherapeutic agents may be selectively toxic to tumor cells because they increase oxidant stress and drive these already stressed cells beyond their limit.33 Therefore, the detailed mechanisms behind the selective toxicity of UA on breast cancer cells require further investigation.
Recent studies have indicated that cancer chemo-preventive agents induce apoptosis in part by generation of ROS and the disruption of redox homeostasis.34,35 Porcine polysaccharide, extracted from porcine cartilage, triggers apoptosis of through a ROS-mediated mitochondrial pathway.36 In the present study, a dramatic increase in ROS was observed in UA-treated MCF-7 cells, and the ROS inhibitor NAC significantly reduced UA-induced ROS production and apoptosis, suggesting that ROS generation is involved in the mechanism of UA-induced apoptosis in MCF-7 cells. There is a close relationship between cellular signaling and the cellular redox state. MAPKs, including JNK, ERK, and p38, are mediators of cellular responses to extracellular signals. The elucidation of MAPK signaling in apoptotic processes may provide the explanation for ROS action. JNK and p38 MAPKs are induced by stress responses and are closely associated with cell death. In contrast, ERK is generally associated with proliferation and growth.37 There are very few reports that relate MAPKs to UA effects. To elucidate the precise mechanism involved in the UA-induced cell death, the effects of UA on MAPK activation were examined. The present study showed that UA treatment resulted in the activation of JNK, c-Jun, and ERK, but not p38. Moreover, UA induced the activation of JNK through a ROS-dependent mechanism as evidenced by loss of JNK phosphorylation by the ROS inhibitor NAC. However, NAC did not affect the phosphorylation of ERK. These data suggest that JNK, but not p38 or ERK, participates in ROS-dependent UA-induced apoptosis.
Mitochondria are thought to be the major pathway for apoptosis, and therefore, targeting the mitochondria is a novel strategy for cancer therapy. In mammalian cells, increased cellular ROS production has been suggested to be responsible for mitochondrial dysfunctions and subsequent cell death. Mitochondrial dysfunctions including the loss of MMP, permeability transition, and release of cytochrome-c from the mitochondrion into the cytosol are associated with apoptosis. Bcl-2 is a thiol-containing protein that prevents apoptosis through a negative regulation on the mitochondrial translocation of Bax.38 This latter protein is known to trigger the release of cytochrome c from the intermembrane space. Once cytochrome-c binds Apaf-1 and procaspase-9, procaspase-9 is cleaved and activates procaspase-3. PARP, known as a poly (ADP-ribose) synthetase and poly (ADP-ribose) transferase, is generally cleaved as a substrate by caspase-3 in apoptosis.39 It catalyzes the transfer of the ADP-ribose moiety from the respiratory co-enzyme NAD+ to a limited number of acceptor proteins involved in chromatin architectures when cells are stimulated by DNA strand-breaks. In this study, the rapid loss of MMP, release of cytochrome-c, increase in Bax-to-Bcl-2 expression ratio, activation of caspase-3 and cleavage of PARP were observed in UA-treated cells, and these events were blocked by NAC. From these results, we demonstrated that UA induced apoptosis through ROS-mediated activation of the mitochondrial apoptotic pathway.
Here, we have also demonstrated that UA visibly reduced volume and weight of subcutaneous tumor masses. After a 21 day treatment with the highest dosage of UA (100 mg kg−1), tumor volume and weight decreased nearly 60% and 70%, respectively, and highest dosage of UA had anti-tumor effects that were comparable to those of CTX, a first-line drug for breast cancer.40 To detect whether UA could also induce apoptotic cell death in vivo, TUNEL staining was performed on subcutaneous tumor tissue sections, and this assay demonstrated that UA induced apoptosis in tumor masses in a dose-dependent manner. Together, these results indicate that UA has the capability of inhibiting human breast cancer MCF-7 cell growth inducing apoptosis in vivo. As many antitumor agents lack tumor selectivity, a large dose is needed to achieve a therapeutic effect. However, a high dose often causes toxic side effects, which limits the use of the agent. Natural products for the treatment or prevention cancer are attractive because of their very low clinical toxicity compared with synthetic antitumor drugs.41 In this study, the toxicity of UA and CTX was evaluated based on body weight changes in nude mice. These results showed that CTX treatment resulted in marked decreases in the body weight of mice, while the highest dosage of UA resulted in no obvious change in body weight, which is consistent with preferential toxicity towards breast cancer cells. It is worth noting that UA has been associated with clinical hepatotoxicity leading to liver failure in humans.42 Although no significant changes in mice weight were observed, the histopathological examination of major organs and blood chemistry of liver function requires further investigation.
In summary, we showed that UA, a fungi-derived dibenzofuran, preferentially kills breast cancer cells by inducing ROS accumulation and JNK stimulation, resulting in the activation of the mitochondrial apoptotic pathway. In an MCF-7 xenograft model, UA significantly suppressed the growth of tumors by inducing apoptosis in the tumor cells without overt toxic side effects. Taken together, the present study demonstrated that UA-induced apoptosis is mediated through a ROS/JNK-dependent mitochondrial/caspase pathway. With its remarkable antitumoral effects and lower toxicity, with further structural modification, UA may be a new cancer drug candidate for the prevention and possible treatment of human breast cancer.
Conflicts of interest
The authors declare no conflict of interest.
Abbreviations
UA | Usnic acid |
ROS | Reactive oxygen species |
NAC | N-Acetylcysteine |
JNK | c-Jun-N-terminal kinase |
MMP | Mitochondrial membrane potential |
BC | Breast cancer |
MAPK | Mitogen-activated protein kinases |
CTX | Cyclophosphamide |
DCFH-DA | 2′7′-Dichloroflurescein diacetate |
ERK | Extracellular regulated protein kinases |
PARP | Poly ADP-ribose polymerase |
FITC | Fluorescein isothiocyanate |
TUNEL | Terminal deoxynucleotidyl transferase-mediated dUTP nick end labeling. |
Acknowledgements
This work was supported by the Jilin Science & Technology Development Plan (grant no. 200505108)
Notes and references
- C. M. Friedenreich, Recent Results Cancer Res., 2011, 188, 125–139 CrossRef CAS.
- C. DeSantis, R. Siegel, P. Bandi and A. Jemal, Ca-Cancer J. Clin., 2011, 61, 409–418 CrossRef PubMed.
- J. L. Khatcheressian, P. Hurley, E. Bantug, L. J. Esserman, E. Grunfeld, F. Halberg, A. Hantel, N. L. Henry, H. B. Muss, T. J. Smith, V. G. Vogel, A. C. Wolff, M. R. Somerfield and N. E. Davidson, J. Clin. Oncol., 2013, 31, 961–965 CrossRef CAS PubMed.
- M. K. Mejdahl, K. G. Andersen, R. Gartner, N. Kroman and H. Kehlet, BMJ, 2013, 346, f1865 CrossRef PubMed.
- L. Ouyang, Z. Shi, S. Zhao, F. T. Wang, T. T. Zhou, B. Liu and J. K. Bao, Cell Proliferation, 2012, 45, 487–498 CrossRef CAS PubMed.
- H. Kennecke, R. Yerushalmi, R. Woods, M. C. Cheang, D. Voduc, C. H. Speers, T. O. Nielsen and K. Gelmon, J. Clin. Oncol., 2010, 28, 3271–3277 CrossRef PubMed.
- R. Nahta, D. Yu, M. C. Hung, G. N. Hortobagyi and F. J. Esteva, Nat. Clin. Pract. Oncol., 2006, 3, 269–280 CrossRef CAS PubMed.
- R. G. Mehta, G. Murillo, R. Naithani and X. Peng, Pharm. Res., 2010, 27, 950–961 CrossRef CAS PubMed.
- L. M. Leone and S. C. Roberts, in Natural Products and Cancer Drug Discovery, Springer, 2013, pp. 193–211 Search PubMed.
- L. D. Kaplan, S. R. Deitcher, J. A. Silverman and G. Morgan, Clin. Lymphoma, Myeloma Leuk., 2014, 14, 37–42 CrossRef PubMed.
- D. D. Von Hoff, T. Ervin, F. P. Arena, E. G. Chiorean, J. Infante, M. Moore, T. Seay, S. A. Tjulandin, W. W. Ma, M. N. Saleh, M. Harris, M. Reni, S. Dowden, D. Laheru, N. Bahary, R. K. Ramanathan, J. Tabernero, M. Hidalgo, D. Goldstein, E. Van Cutsem, X. Wei, J. Iglesias and M. F. Renschler, N. Engl. J. Med., 2013, 369, 1691–1703 CrossRef CAS PubMed.
- L. Beranova, A. R. Pombinho, J. Spegarova, M. Koc, M. Klanova, J. Molinsky, P. Klener, P. Bartunek and L. Andera, Apoptosis, 2013, 18, 739–750 CrossRef CAS PubMed.
- J. H. Kim, M. Chae, W. K. Kim, Y. J. Kim, H. S. Kang, H. S. Kim and S. Yoon, Br. J. Pharmacol., 2011, 162, 773–784 CrossRef CAS PubMed.
- V. J. Venditto and E. E. Simanek, Mol. Pharm., 2010, 7, 307–349 CrossRef CAS PubMed.
- L. Wang, T. Narui, H. Harada, C. F. Culberson and W. L. Culberson, Bryologist, 2001, 104, 345–349 CrossRef.
- K. Ingolfsdottir, Phytochemistry, 2002, 61, 729–736 CrossRef CAS.
- M. Cocchietto, N. Skert, P. L. Nimis and G. Sava, Naturwissenschaften, 2002, 89, 137–146 CrossRef CAS.
- M. Mayer, M. A. O'Neill, K. E. Murray, N. S. Santos-Magalhaes, A. M. Carneiro-Leao, A. M. Thompson and V. C. Appleyard, Anticancer Drugs, 2005, 16, 805–809 CrossRef CAS.
- E. Einarsdottir, J. Groeneweg, G. G. Bjornsdottir, G. Harethardottir, S. Omarsdottir, K. Ingolfsdottir and H. M. Ogmundsdottir, Planta Med., 2010, 76, 969–974 CrossRef CAS PubMed.
- N. Singh, D. Nambiar, R. K. Kale and R. P. Singh, Nutr. Cancer, 2013, 65(suppl 1), 36–43 CrossRef CAS PubMed.
- M. Backorova, R. Jendzelovsky, M. Kello, M. Backor, J. Mikes and P. Fedorocko, Toxicol. In Vitro, 2012, 26, 462–468 CrossRef CAS PubMed.
- S. Fulda and K. M. Debatin, Oncogene, 2006, 25, 4798–4811 CrossRef CAS PubMed.
- A. G. Letai, Nat. Rev. Cancer, 2008, 8, 121–132 CrossRef CAS PubMed.
- M. Valko, D. Leibfritz, J. Moncol, M. T. Cronin, M. Mazur and J. Telser, Int. J. Biochem. Cell Biol., 2007, 39, 44–84 CrossRef CAS PubMed.
- H. U. Simon, A. Haj-Yehia and F. Levi-Schaffer, Apoptosis, 2000, 5, 415–418 CrossRef CAS.
- G. Barja, Prog. Mol. Biol. Transl. Sci., 2014, 127, 1–27 Search PubMed.
- J. Chandra, A. Samali and S. Orrenius, Free Radical Biol. Med., 2000, 29, 323–333 CrossRef CAS.
- T. Wada and J. M. Penninger, Oncogene, 2004, 23, 2838–2849 CrossRef CAS PubMed.
- F. Chen, Cancer Res., 2012, 72, 379–386 CrossRef CAS PubMed.
- Y. T. Ip and R. J. Davis, Curr. Opin. Cell Biol., 1998, 10, 205–219 CrossRef CAS.
- Y. Son, Y. K. Cheong, N. H. Kim, H. T. Chung, D. G. Kang and H. O. Pae, J. Signal Transduction, 2011, 7, 926–939 Search PubMed.
- C. Runchel, A. Matsuzawa and H. Ichijo, Antioxid. Redox Signaling, 2011, 15, 205–218 CrossRef CAS PubMed.
- L. Raj, T. Ide, A. U. Gurkar, M. Foley, M. Schenone, X. Li, N. J. Tolliday, T. R. Golub, S. A. Carr, A. F. Shamji, A. M. Stern, A. Mandinova, S. L. Schreiber and S. W. Lee, Nature, 2011, 475, 231–234 CrossRef CAS PubMed.
- V. Sharma, D. Anderson and A. Dhawan, Apoptosis, 2012, 17, 852–870 CrossRef CAS PubMed.
- B. Sung, S. Prasad, J. Ravindran, V. R. Yadav and B. B. Aggarwal, Free Radical Biol. Med., 2012, 53, 1977–1987 CrossRef CAS PubMed.
- W. Song, P. Hu, Y. Shan, M. Du, A. Liu and R. Ye, Food Funct., 2014, 5, 2486–2493 CAS.
- C. H. Wong, K. B. Iskandar, S. K. Yadav, J. L. Hirpara, T. Loh and S. Pervaiz, PLoS One, 2010, 5, e9996 Search PubMed.
- J. C. Martinou and R. J. Youle, Dev. Cell, 2011, 21, 92–101 CrossRef CAS PubMed.
- A. H. Boulares, A. G. Yakovlev, V. Ivanova, B. A. Stoica, G. Wang, S. Iyer and M. Smulson, J. Biol. Chem., 1999, 274, 22932–22940 CrossRef CAS PubMed.
- N. Penel, A. Adenis and G. Bocci, Crit. Rev. Oncol. Hematol., 2012, 82, 40–50 CrossRef PubMed.
- B. B. Mishra and V. K. Tiwari, Eur. J. Med. Chem., 2011, 46, 4769–4807 CrossRef CAS PubMed.
- F. A. Durazo, C. Lassman, S. H. Han, S. Saab, N. P. Lee, M. Kawano, B. Saggi, S. Gordon, D. G. Farmer, H. Yersiz, R. L. Goldstein, M. Ghobrial and R. W. Busuttil, Am. J. Gastroenterol., 2004, 99, 950–952 CrossRef PubMed.
|
This journal is © The Royal Society of Chemistry 2015 |