DOI:
10.1039/C4RA10386F
(Paper)
RSC Adv., 2015,
5, 1712-1717
Hemoglobin detection using curcumin nanoparticles as a colorimetric chemosensor
Received
13th September 2014
, Accepted 21st November 2014
First published on 24th November 2014
Abstract
This article presents a simple and efficient measurement system for quantitative sensing of blood hemoglobin (Hgb) using curcumin nanoparticles (CURNs). The Hgb monitoring is based on CURNs aggregation in the presence of Hgb, which leads to a decrease in the absorption intensity of CURNs. In this study the analytical signal for the measurement of blood Hgb concentration was defined as the difference in absorption intensity of the CURNs in the absence and presence of Hgb. The method was optimized to enhance the selectivity and sensitivity of the method by investigating the effect of variables such as pH of the sample solution, buffer concentration, required amount of CURNs, equilibrium time and tolerance limit of various probable interferences. Under optimum conditions, the difference in absorption intensity of the CURNs was linearly proportional to the concentration of Hgb in two ranges 1–40 μg mL−1 and 150–1200 μg mL−1 with a detection limit of 0.1 μg mL−1. The relative standard deviation for ten replicate measurements of 20 μg mL−1 and 600 μg mL−1 of Hgb was 3.6% and 3.1% respectively. The sensing method was successfully applied for the measurement of Hgb in human blood with satisfactory results.
Introduction
Hemoglobin is the protein component of red blood cells that is responsible for carrying oxygen between the respiratory organs such as lungs or gills to the rest of the body. The Hgb molecule has a quaternary structure comprised of four polypeptide chains and each chain binds to a heme group. Each heme group contains an iron atom chelated to a protoporphyrin ring.1–5 The determination of the Hgb concentration is greatly utilized in blood tests for the diagnosis of many diseases such as anemia, thalassemia, heart disease, leukemia, sickle cell disease and excessive loss of blood.2,4,6–8 Considering the importance of the easy and accurate quantitative analysis of Hgb in blood cells, numerous attempts have been made to determine the concentration of Hgb. Hgb has been measured traditionally by Drabkin's test. In this standard method, cyanohaemoglobin is formed as a result of the interaction of cyanide ion with oxidized hemoglobin which shows an absorbance peak at λ = 540 nm.9 The other methods for Hgb measurement include electrochemistry,8,10–12 spectrophotometry,6,7,13,14 fluorimetry,1,5 chemiluminescence,15 high performance liquid chromatography16–18 and noninvasive methods.19–23 Various types of nanoparticles have been applied for the measurement of Hgb.1,3,4 Wang et al. investigated the use of N-acetyl-L-cysteine (NAC) capped CdHgSe quantum dot (QD) for determination of Hgb.1 Their proposed method for the measurement of Hgb was based on fluorescence quenching of the capped CdHgSe QDs. Pakapongpan et al. reported a self-assembled methylene blue-multiwalled carbon nanotubes nanohybrid modified on glassy carbon electrode as an electrochemical sensor for the determination of Hgb.4 In another work, Gao et al. developed a functional electrode for Hgb measurement. In research of this group, Hgb sensor electrode was prepared by implanting NH2+ into indium tin oxide (ITO) film followed by the deposition of gold nanoparticles on the surface of this electrode.3 In spite of the recent improvement in the determination of Hgb, development of simple, efficient, cost-effective and user-friendly method for Hgb measurement is still demanded. The majority of the aforementioned techniques suffer from the use of harmful, toxic and carcinogenic reagents, and some require sophisticated and expensive equipment.
Curcumin [diferuloylmethane, or (E,E)-1,7-bis (4-hydroxy-3-methoxy-phenyl)-1,6-heptadiene-3,5-dione] is a biologically hydrophobic polyphenol compound which has been known as the yellow-orange pigment isolated from the dried root of the rhizome Curcuma Longa. Curcumin has been greatly consumed as a coloring, seasoning and preserving agent in several food. Curcumin shows keto–Enol tautomerism.24–26 Curcumin is a biomaterial exhibiting properties such as antioxidant, antibacterial, anti-inflammatory, anticarcinogenic, wound healing and can readily chelate the metal ions to form complexes.24,27 Complexing ability and affinity interaction of curcumin with some metal ions such as iron and copper was recognized and was applied in many studies.28 Jiao et al. reported that curcumin exhibits the characteristic of an iron chelator because of its high affinity for modulates proteins of iron metabolism in the cells. Ability to iron binding of curcumin is comparable to the iron chelator such as nitrilotriacetic acid and many other iron chelators.28,29 Pineda et al. have calculated the formation constants of the complexes curcumin with Fe(III) and Fe(II).30 Baum et al. have suggested that a possible mechanism by which curcumin may decrease the Alzheimer disease would be through its aptitude as an iron chelator.31 Within the past few years, nanomaterial and nanoparticle complexes have been utilized in various fields such as medicine and chemistry because of their fascinating propertie.32
In this work we have developed a novel and simple method for sensitive determination of Hgb using its effect on optical properties of curcumin nanoparticles. CURN interact with Hgb and undergoes aggregation. As a subsequence, the change in optical characteristics of CURNs occurs. This optical change in the absorption intensity of CURNs is proportional to the concentration of Hgb and was used as an analytical signal for quantitative determination of blood Hgb concentration.
Experimental
Apparatus
The spectrophotometric measurements and recording absorption spectra were performed by a GBC UV-visible spectrophotometer (Cintra 101, Sidney, Australia) using 1 cm glass cuvette. TEM images were acquired with a transmission electron microscope (Zeiss-EM10C-80 KV, Germany). A Metrohm digital pH-Meter model 632 with a combined glass electrode (Herisau, Switzerland) was used for the pH measurements. An ultrasound bath (DSA 100-SK2, 100 W power, 40 kHz Frequency, China) was utilized for fabrication of nanoparticles ultrasonically. A Labrota 4000 rotary evaporator (Heidolph, Germany) was used for solvent removing. Reference spectrophotometric method was performed using an auto hematology analyzer (BC 3000 plus, Mindray, China) for clinical determination of Hgb.
Reagents and materials
All chemicals were of analytical grade and double distilled water was used throughout. A stock solution of Hgb (2000 mg L−1) was prepared by diluting 1 mL of Hgb solution with a concentration of 20 g dL−1 (Ziest Chemie Diagnostics, Iran) to 100 mL in a volumetric flask with water. This stock solution was kept at 4 °C before use. Britton–Robinson buffer pH 12 was prepared by the addition of NaOH 0.2 mol L−1 solution to the mixture of phosphoric acid (Merck), boric acid (Merck) and citric acid (Merck) (0.04 mol L−1 each) and adjusting the pH to 12 using a pH meter. 5% (v/v) of Triton X-100 (Merck) was prepared by diluting 5 mL of the reagent to 100 mL.
Preparation of curcumin nanoparticles
The CURNs were synthesized according to our previously reported technique33 as follows: 125 mg of curcumin was completely dissolved in 25 mL of dichloromethane to prepare the organic phase. The aqueous phase was prepared by adding 10 mL of Triton X-100 5% (v/v) to 90 mL of hot water (70–80 °C). After this step, 2 mL of organic phase was slowly and dropwise introduced into the aqueous phase (about 10 drops per minute) under ultrasonic conditions, with an ultrasonic power of 100 W and a frequency of 40 kHz. After adding the organic phase into the aqueous phase, a yellowish clear solution was observed. Additional sonication process was applied for 20 min to ensure that the complete dispersion is occurred. Following that, the content was stirred on a magnetic stirrer at room temperature for 20 min. Finally, this solution was placed in a rotary evaporator under reduced pressure in order to remove dichloromethane completely. The CURNs solution was stored in a brown bottle. The total concentration of CURNs solution was 0.271 mmol L−1 and it was stable for more than six months. TEM image of the synthesized CURN is presented in Fig. 1.
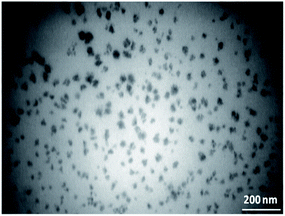 |
| Fig. 1 TEM image of synthesized CURNs. | |
Measurement procedure
In typical experiments, test solutions were prepared by placing 1.0 mL of the synthesized CURNs solution (0.271 mmol L−1), 3.0 mL of Britton–Robinson buffer solution pH 12 and different amount of Hgb solutions into 10 mL volumetric flask and diluting the solution to the mark with water and mixing thoroughly. After 90 min, the absorbance of the solution was measured at 465 nm. A blank solution was also prepared in the same way without adding any Hgb solution.
Results and discussion
Sensing strategy
Our observation showed that the absorption intensity of CURNs is decreased after the addition of Hgb. Fig. 2 illustrates the UV-vis absorption spectra of CURNs in the presence of various concentration of Hgb. As shown in Fig. 2 the absorption intensity of the CURNs is significantly decreased after addition of Hgb. This decrease in absorption intensity is proportional to the Hgb concentration and was utilized as an analytical signal for Hgb measurement. The possible mechanism here for the color change of the CURN induced by Hgb is due to the interaction of CURN as an iron chelator with hem group of Hgb as an iron source. Thereupon, an energy transfer complex is forming between π-electron rich CURNs and Hgb as an electron deficient compound.28–30 As an outcome of this interaction, CURNs undergoes aggregation and consequently the absorption intensity is increased in the presence of Hgb. The TEM images of CURNs in the presence and absence of Hgb shown in Fig. 3 indicates the aggregation of CURNs in the presence of Hgb. A plausible mechanism for above suggested strategy is schematically depicted in Scheme 1.
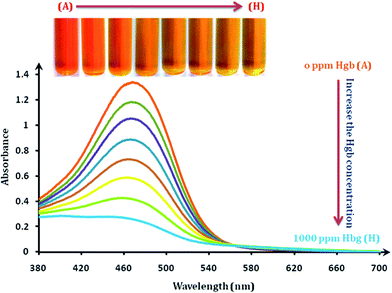 |
| Fig. 2 The UV-vis spectra and color changes of CURNs after addition of hemoglobin with different final concentrations. | |
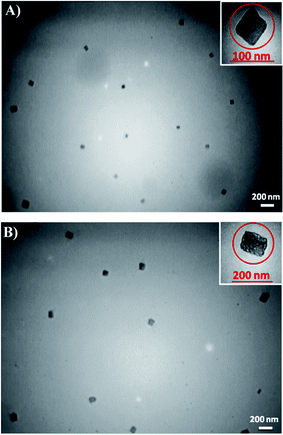 |
| Fig. 3 TEM images of CURNs (A) in the absence of hemoglobin and (B) in the presence of hemoglobin at pH 12. | |
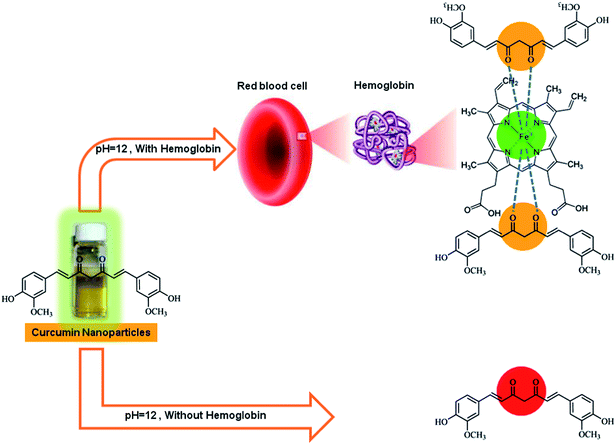 |
| Scheme 1 Schematic representation for determination of blood hemoglobin concentration using CURNs. | |
Effect of pH
During recent years, various strategies were applied for the investigation the pH and its effect on chemical interactions, owing to importance of pH as an important factor.34–37
To evaluate the effect of pH on Hgb sensing and in order to establish an optimum pH, the Hgb monitoring procedure was examined in various pH medium in the range of 9–13 whereas the other experimental parameters were kept constant. The pH was adjusted to the desire value by the addition of 3 mL of Britton–Robinson buffer with pH values in the range of 9–13 to the solution. The results of this investigation presented in Fig. 4 reveal that as the pH of the medium was above 9, the analytical signal increased significantly. The analytical signal for determination of 1000 μg mL−1 of Hgb was highest at pH 12 and decreased after that. Above pH 8, the enolate form of curcumin predominates, and acts mainly as an electron donor.24 Hence, the pH value of 12 was chosen for further experiments. On the other hand, choosing a proper buffer to adjust the pH of aqueous medium is important when a sensing system is designed. Hence the influence of various buffers besides Britton–Robinson with pH 12 such as phosphate buffer on the absorption intensity of this method was investigated. The experimental results demonstrated that the maximum analytical signal occurred when the Britton–Robinson buffer was used. In order to assess the effect of the buffer concentration pH 12 on the current study, different concentration of Britton–Robinson buffer pH 12 was examined. As can be seen in Fig. 5, by increasing the buffer concentration, the analytical signal increased and reached a plateau at 0.012 mol L−1 and above this concentration, no significant change in the analytical signal was observed. Thus, the concentration of 0.012 mol L−1 of Britton–Robinson buffer was selected for further studies.
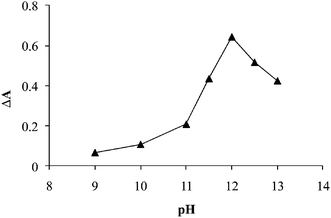 |
| Fig. 4 The effect of pH on determination of 1000 μg mL−1 of hemoglobin using CURNs. | |
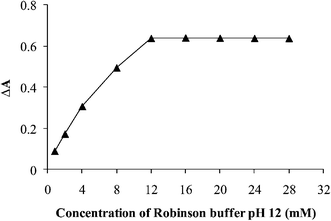 |
| Fig. 5 The effect of concentration of Britton–Robinson buffer pH = 12 on determination of 1000 μg mL−1 of hemoglobin using CURNs. | |
Effect of CURNs concentration
The effect of CURNs concentration was investigated in order to choose the optimum conditions for the determination of Hgb. The results of this study indicated that the concentration of the CURNs has a significant effect on the absorption intensity of the system and the analytical signal is increased by increasing the curcumin concentration and as a consequence higher ΔA was achieved when CURNs concentration was 27.1 μmol L−1. Therefore, this concentration was chosen as optimum in further investigations and 1.0 mL of synthesized curcumin (0.27 mmol L−1) was added to 10 mL samples to provide this concentration.
Effect of equilibrium time
During the course of this investigation, we found that Hgb monitoring using CURNs is time dependent. Hence, the effect of time on current work in range of 2–120 min was investigated. As can be seen in Fig. 6 as the equilibrium time was prolonged, the analytical signal increased quickly and eventually reached a plateau after 90 min. The equilibrium times above 90 min did not have significant effect on the method performance. Therefore, equilibrium time of 90 min was chosen as optimum time for Hgb monitoring.
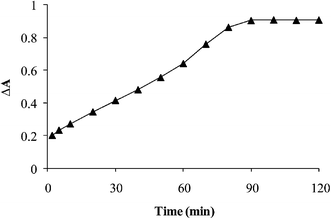 |
| Fig. 6 The effect of time on determination of 1000 μg mL−1 of hemoglobin using CURNs. | |
Analytical performance and validation
The analytical characteristics of the proposed method were evaluated using the recommended procedure under the optimum conditions. As can be seen in Fig. 7, the calibration curve was linear in two different ranges of 1–40 μg mL−1 of Hgb and 150–1200 μg mL−1 of Hgb with correlation coefficients (r) of 0.9973 and 0.9987 and the linear regression equations for Hgb were ΔA = 0.0078C + 0.0741 and ΔA = 0.0004C + 0.4934 respectively, where C is the concentration of Hgb in μg mL−1. The limit of detection was determined as a concentration giving an absorption signal to noise ratio of (3
:
1) was found to be 0.1 μg mL−1. The relative standard deviation for ten replicate measurements of 20 μg mL−1 and 600 μg mL−1 of Hgb were 3.6% and 3.1% respectively.
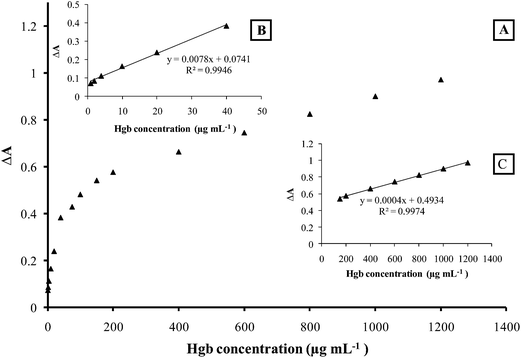 |
| Fig. 7 Calibration plots of hemoglobin sensing using CURNs (A) in the range 1–1200 μg mL−1 (B) in the range 1–40 μg mL−1 and (C) in the range 150–1200 μg mL−1 of hemoglobin. | |
Interferences
The competitive experiments were performed by adding some chemicals probably existing in the blood samples and some other ions to evaluate their ability to affect the Hgb sensing under the optimum experimental conditions stated above. Any relative error equal or greater than ±5% from the analytical signal value was considered as interference. The experiments were carried out at a fixed concentration of Hgb (1000 μg mL−1) and measuring the analytical signal with or without the probable interfering chemical. The results of this investigation are listed in Table 1. Compounds such as creatine, oxalate, protein and glucose did not interfere up to 2000 μg mL−1.
Table 1 The influence of co-exiting chemical interfering on the Hgb measurement
Interfering ion |
coexisting ion concentration (μg mL−1) |
Na+, Cl−, Ca2+, K+, PO43−, urea |
10 000 |
Creatinine, oxalate, SO42−, NO3−, NH4+ |
2000 |
Glucose, protein, EDTA, Br−, I−, Mg2+ |
1000 |
CO32−, HCO3−, Pb2+, C2O42− |
500 |
Cysteine, Zn2+ |
100 |
Fe3+, Cu2+ |
2 |
Determination of Hgb concentration in clinical blood
The clinical efficiency of the developed method for determination of Hgb concentration was validated by analyzing blood samples of 15 patients (volunteers). The blood samples were centrifuged for the settlement of red blood cells (RBCs) and the RBC phase was diluted by 20-fold with water. Then 1.0 mL of CURNs, 3 mL of Britton–Robinson buffer pH 12 and 1 mL of diluted blood sample were transferred into a 10 mL volumetric flask, made up to the mark with water and mixed thoroughly. The absorbance was measured at 465 nm after 90 min. The Hgb concentration of blood samples was also determined using an auto hematology analyzer as the reference method for clinical determination of Hb. The results of both methods are plotted in Fig. 8 for comparison. The regression coefficient for this comparison was 0.9894, which indicate that a good agreement is achieved between the results of the two methods. Moreover, the obtained results show the usability of proposed method for Hgb measurement in clinical laboratories.
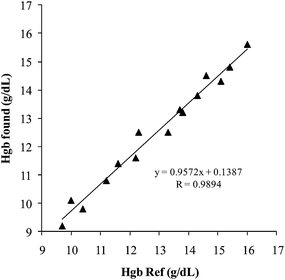 |
| Fig. 8 Correlation between obtained results of blood hemoglobin analysis using CURNs and spectrophotometric assay using auto hematology analyzer as reference instrumental method recommended for clinical uses. | |
Conclusions
The present work describes a novel straightforward method for Hgb monitoring. This method offers an alternative colorimetric approach to the classical colorimetric sensing of Hgb. The developed colorimetric method for Hgb sensing is simple, facile and cost-effective because does not require sophisticated instrumentation and surface modification of the nanoparticles. The method was successfully applied to the determination of Hgb in human blood samples and was validated by comparing the results with those obtained by a reference spectrophotometric method. The results of the present investigations in this study confirmed that the method is precise and accurate and could be suggested for utilizing in clinical labs for Hgb monitoring. The analytical characteristics of the recommended method for the determination of Hgb concentration are significantly improved over previously reported methods.
Acknowledgements
The authors are sincerely grateful to Shahid Chamran University, Research Council for the financial support of this project (Grant 1393). The financial support of the Iranian Nanotechnology Initiative Council is greatly appreciated.
References
- Q. Wang, G. Zhan and Ch. Li, Spectrochim. Acta, Part A, 2014, 117, 198 CrossRef CAS PubMed.
- S. R. Zarchi, A. A. Saboury, J. Hong, H. Ghourchian, P. Norouzi, A. A. M. Movahedi, M. R. Ganjali and A. Javed, Biotechnol. Appl. Biochem., 2007, 47, 153 CrossRef PubMed.
- D. M. Gao, Y. Y. Sun, Q. Zhao, J. B. Hu and Q. L. Li, Microchim. Acta, 2008, 160, 241 CrossRef CAS.
- S. Pakapongpan, R. Palangsuntikul and W. Surareungchai, Electrochim. Acta, 2011, 56, 6831 CrossRef CAS PubMed.
- X. F. Yang, X. Q. Guo and H. Li, Talanta, 2003, 61, 439 CrossRef CAS.
- M. Bond, C. Elguea, J. S. Yan, M. Pawlowski, J. Williams, A. Wahed, M. Oden, T. S. Tkaczyk and R. R. Kortum, Lab Chip, 2013, 13, 2381 RSC.
- D. S. Kim, J. H. Choi, M. H. Nam, J. W. Yang, J. J. Pak and S. Seo, Sens. Actuators, B, 2011, 157, 103 CrossRef CAS PubMed.
- A. K. Tatikonda, M. Tkachev and R. Naaman, Biosens. Bioelectron., 2013, 45, 201 CrossRef CAS PubMed.
- D. L. Drabkin and H. J. Austin, J. Biol. Chem., 1935, 112, 105 CAS.
- Sh. Wu, W. Tan and H. Xu, Analyst, 2010, 135, 2523 RSC.
- M. Liu, G. Shi, L. Zhang, G. Zhao and L. Jin, Anal. Bioanal. Chem., 2008, 391, 1951 CrossRef CAS PubMed.
- M. R. Majidi, A. Saadatirad and E. Alipour, Electroanalysis, 2011, 23, 1984 CrossRef CAS.
- M. D. Frenchik, S. J. McFaul and L. I. Tsonev, Clin. Chim. Acta, 2004, 339, 199 CrossRef CAS PubMed.
- E. Mieczkowska, R. Koncki and L. Tymecki, Anal. Bioanal. Chem., 2011, 399, 3293 CrossRef CAS PubMed.
- T. I. Quickenden and P. D. Cooper, Luminescence, 2001, 16, 251 CrossRef CAS PubMed.
- M. R. van Bommel, A. P. J. M. de Jong, U. R. Tjaden, H. Irth and J. van der Greef, J. Chromatogr. A, 2000, 886, 19 CrossRef CAS.
- T. H. J. Huisman, Anal. Chim. Acta, 1997, 352, 187 CrossRef CAS.
- R. Wissiack, B. de la Calle, G. Bordin and A. R. Rodriguez, Meat Sci., 2003, 64, 427 CrossRef CAS.
- O. Abdallah, K. Abo Alam and A. Bolz, IFMBE Proceedings, 2008, vol. 22, p. 1738 Search PubMed.
- U. Timm, E. Lewis, D. McGrath, J. Kraitl and H. Ewald, ICBME Proceedings, 2009, vol. 23, p. 825 Search PubMed.
- A. Opp, U. G. Hofmann, S. Boye, L. Dibbelt and H. Gehring, IFMBE Proceedings, 2009, vol. 25, p. 590 Search PubMed.
- T. Isosu, Sh. Obara, A. Hosono, S. Ohashi, Y. Nakano, T. Imaizumi, M. Mogami and M. Murakawa, J. Clin. Monit. Comput., 2013, 27, 55 CrossRef PubMed.
- D. Myers, M. McGraw, M. George, K. Mulier and G. Beilman, Crit. Care, 2009, 13(suppl 5), S2 CrossRef PubMed.
- R. A. Sharma, A. J. Gescher and W. P. Steward, Eur. J. Cancer, 2005, 41, 1955 CrossRef CAS PubMed.
- Bhawana, R. K. Basniwal, H. S. Buttar, V. K. Jain and N. Jain, J. Agric. Food Chem., 2011, 59, 2056 CrossRef CAS PubMed.
- F.-L. Yen, T.-H. WU, Ch.-W. Tzeng, L.-T. Lin and Ch.-Ch. Lin, J. Agric. Food Chem., 2010, 58, 7376 CrossRef CAS PubMed.
- A. Pandya, H. Goswami, A. Lodha and Sh. K. Menon, Analyst, 2012, 137, 1771 RSC.
- Y. Jiao, J. Wilkinson, E. Ch. Pietsch, J. L. Buss, W. Wang, R. Planalp, F. M. Torti and S. V. Torti, Free Radical Biol. Med., 2006, 40, 1152 CrossRef CAS PubMed.
- Y. Jiao, J. Wilkinson, X. Di, W. Wang, H. Hatcher, N. D. kock, R. D'Agostino, M. A. Knovich, F. M. Torti and S. V. Torti, Blood, 2009, 113, 462 CrossRef CAS PubMed.
- M. B. Pineda, M. T. R. Silva, M. A. R. Romob, E. G. Vergara and A. R. Hernandez, Spectrochim. Acta, Part B, 2004, 60, 1105 CrossRef.
- L. Baum and A. Ng, J. Alzheimer's Dis., 2004, 6, 367 CAS.
- G. Hribar, A. Znidarsic, M. Bele, U. Maver, S. Caserman, M. Gaberscek and V. Gaberc-Porekar, J. Nanopart. Res., 2011, 13, 3019 CrossRef CAS.
- N. Pourreza and H. Golmohammadi, Talanta, 2014, 119, 181 CrossRef CAS PubMed.
- U. Maver, T. Maver, A. Znidarsic, Z. Persin, M. Gaberscek and K. Stana-Kleinschek, Mater. Tehnol., 2011, 45, 259 CAS.
- U. Maver, A. Znidarsic and M. Gaberscek, J. Mater. Chem., 2011, 21, 4071 RSC.
- M. Navarro and J. A. Planell, Nanotechnology in regenerative medicine: methods and protocols, Humana Press; Springer, New York, 2011, vol. xi, p. 319 Search PubMed.
- Y. F. Dufrene and P. Hinterdorfer, Pfluegers Arch., 2008, 456, 237 CrossRef CAS PubMed.
|
This journal is © The Royal Society of Chemistry 2015 |