DOI:
10.1039/C5QO00274E
(Research Article)
Org. Chem. Front., 2015,
2, 1608-1615
Blue AIE luminogens bearing methyl groups: different linkage position, different number of methyl groups, and different intramolecular conjugation†
Received
1st September 2015
, Accepted 7th October 2015
First published on 8th October 2015
Abstract
In this paper, four AIE-active luminogens (Methyl-BTPE, mMethylTPE-pTPE, oMethyl-BTPE and oMethylTPE-pTPE) exhibiting blue emissions were successfully synthesized, in which methyl groups of different numbers were introduced at different positions, to subtly tune the intramolecular conjugation. Their thermal, optical and electronic properties were fully investigated. The corresponding non-doped OLEDs exhibited high electroluminescence efficiencies with Lmax, ηC, max, and ηP, max values of up to 14
644 cd m−2, 4.06 cd A−1, and 9.7 lm W−1, respectively, showing the feasibility of this facile synthetic approach for the construction of blue AIE fluorophores.
Introduction
Since the first report of a multi-layered Organic Light-Emitting Diode (OLED) by Tang et al. in 1987, extensive academic research and industrial applications have resulted in a great number of publications and patents during the past few decades.1 In order to realize full-color displays, the bottleneck to progress is the development of blue and deep-blue emitters. However, due to their intrinsically large band gap, the device performance of an OLED is often inferior to that of the red and green counterparts, no matter how good the blue luminogens reported so far have been.2,3 On the other hand, the notorious aggregation-caused quenching (ACQ) effect of conventional emitters is harmful to device efficiency. Therefore, the development of blue-emitting materials, especially deep-blue ones, with both good efficiencies and color purities becomes particularly important.4,5
Pioneered by the group of Ben Zhong Tang, great attention has been attracted to the development of efficient AIE (aggregation-induced emission) emitters in OLEDs and sensors, for their inherent advantages over conventional ACQ materials: they are highly emissive in the solid state while they show faint fluorescence when dissolved in a good solvent.6a–c Besides, there are also some other reports concerning this phenomenon.6d–g With consideration to both facile synthesis and excellent AIE effect, tetraphenylethene (TPE) was selected as the building block for the construction of AIE fluorophores.7 By directly attaching TPE to some traditional ACQ chromophores, new AIE emitters with outstanding EL performance have been obtained.8,9 However, blue or deep-blue AIE luminogens are still very scarce, because it is difficult to retain good EL performances and large band gaps for the molecules simultaneously. For example, 4,4′-bis(1,2,2-triphenylvinyl) biphenyl (BTPE),9c constructed by two TPE units, exhibited outstanding improvement in its electroluminescence (EL) performance, with a current efficiency of up to 7.3 cd A−1 (0.45 cd A−1 for TPE), however, the maximum EL emission wavelength was red-shifted to a large extent, from 445 (deep-blue) to 488 nm (sky-blue). In order to conquer this difficulty,10 recently we have reported that by simply introducing additional groups with different sizes and degrees of conjugation to the 2,2′-positions of BTPE,10e four derivatives with blue or deep-blue EL emissions can be obtained. Among them, Methyl-BTPE is the most promising derivative with deep-blue EL emission since, after adding the non-conjugated methyl groups, the dihedral angle of the biphenyl core is almost perpendicular according to the theoretical calculation. However, the current efficiency of the device is barely satisfactory. Therefore, in order to further improve the device efficiency of Methyl-BTPE without sacrificing the deep-blue EL emission, and also investigate the relationship between device performance and the molecular structure of AIE emitters, we have changed the linkage positions and number of methyl groups, and designed three Methyl-BTPE derivatives, namely mMethylTPE-pTPE, oMethyl-BTPE and oMethylTPE-pTPE (Scheme 1). It is anticipated that the intramolecular conjugation between the two TPE units or TPE itself could be well tuned by these additional methyl groups, resulting in deep-blue emission and good device efficiency (Chart 1). The resultant non-doped OLEDs demonstrate a maximum electroluminescence efficiency of 4.06 cd A−1, which is much higher than 2.31 cd A−1 from Methyl-BTPE.
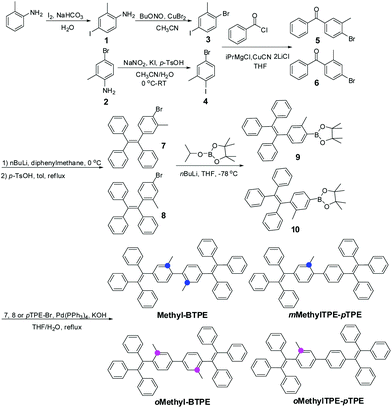 |
| Scheme 1 Synthetic routes to Methyl-BTPE, mMethylTPE-pTPE, oMethyl-BTPE and oMethylTPE-pTPE. | |
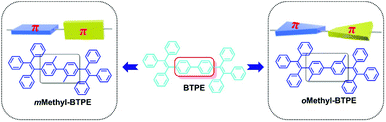 |
| Chart 1 A diagram of the synthetic idea presented in this work. | |
Results and discussion
The synthetic routes for Methyl-BTPE, mMethylTPE-pTPE, oMethyl-BTPE and oMethylTPE-pTPE are illustrated in Scheme 1. The key steps are the construction of the benzophenone derivatives 5 and 6, starting from aniline and 4-bromo-2-methylaniline respectively. By utilizing the different reactivities of iodine and bromine atoms, we successfully obtained the methyl-substituted precursors 3 and 4,11 which were converted to compounds 5 and 6 easily. The synthesis of bromo-TPE and boronic ester were started from compounds 5 and 6. The final products were conveniently produced by palladium-catalyzed Suzuki coupling reactions of the corresponding aromatic bromide and boronic ester with yields in the range of 50 to 85%. They were all purified by column chromatography on silica gel using petroleum ether-dichloromethane as eluent and were fully characterized by 1H and 13C NMR, mass spectrometry, and elemental analysis.
The thermal properties of the new compounds were investigated using thermal gravimetric analysis (TGA) and differential scanning calorimetry (DSC). As listed in Table 1, the four BTPE derivatives exhibit high thermal decomposition temperatures (Td, corresponding to 5% weight loss, Fig. 1A), ranging from 378 to 393 °C. Among them, Methyl-BTPE shows a lower Td value than the other three, possibly due to its more twisted conformation caused by the methyl groups at the 2,2′-positions of the biphenyl core. Only the glass transition temperatures (Tg, Fig. 1B) for Methyl-BTPE and oMethylTPE-pTPE were observed with the Tg values of 88 and 105 °C, respectively, indicating the less twisted conformation of oMethylTPE-pTPE with only one methyl group at the 3-position of the biphenyl core.
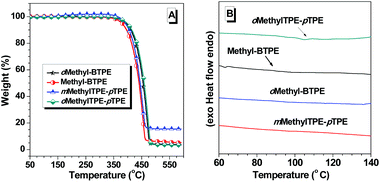 |
| Fig. 1 (a) TGA and (b) DSC (second heating cycle) thermograms of Methyl-BTPE, mMethylTPE-pTPE, oMethyl-BTPE and oMethylTPE-pTPE recorded under N2 at a heating rate of (A) 10 and (B) 15 °C min−1. | |
Table 1 Thermo, optical and electrochemical properties of Methyl-BTPE, mMethylTPE-pTPE, oMethyl-BTPE and oMethylTPE-pTPE
|
T
d
(°C) |
T
g
(°C) |
E
g
(eV) |
E
HOMO
(eV) |
E
LUMO
(eV) |
PL λmax |
Φ
F (%) |
λ
max, abs
|
Aggrf (nm) |
Film (nm) |
Aggrf |
Solng (nm) |
5% weight loss temperature measured by TGA under N2.
Glass-transition temperature measured by DSC under N2.
Band gap estimated from the optical absorption band edge of the solution.
Calculated from the onset oxidation potentials of the compounds.
Estimated using the empirical equation ELUMO = EHOMO + Eg.
Determined in THF : H2O = 1 : 99 solution.
Observed from absorption spectra in dilute THF solution, 10 μM.
|
Methyl-BTPE |
378 |
88 |
3.30 |
−5.53 |
−2.23 |
469 |
457 |
63.3 |
315 |
mMethylTPE-pTPE |
392 |
— |
3.53 |
−5.54 |
−2.01 |
482 |
481 |
89.1 |
312 |
oMethyl-BTPE |
393 |
— |
3.41 |
−5.53 |
−2.12 |
466 |
485 |
57.8 |
300 |
oMethylTPE-pTPE |
397 |
105 |
3.24 |
−5.54 |
−2.30 |
487 |
474 |
62.4 |
322 |
Fig. 2 shows the UV-vis absorption spectra of Methyl-BTPE, mMethylTPE-pTPE, oMethyl-BTPE and oMethylTPE-pTPE in THF. Their maximum absorption wavelengths are 315, 312, 300 and 322 nm, further showing that the intramolecular conjugation could be well tuned by changing the number and linkage positions of the methyl groups (340 nm for BTPE). Among them, it was observed that oMethyl-BTPE is even less conjugated than Methyl-BTPE. Probably, this should be due to the reduced conjugation degree of TPE itself when the methyl groups are at the 3,3′-positions, although the dihedral angle of the biphenyl core remained almost the same. To evaluate their AIE characteristics, THF and water were chosen as the solvent pair due to their good miscibility. Fig. 3 shows the PL spectra of the new luminogens in THF–water mixtures at different water fractions (fw). It is clearly observed that, the PL curves are all practically flat lines parallel to the abscissa in pure THF solutions, demonstrating the weak emission properties of the solution. However, when the water fraction is over 90%, the emission intensities increase swiftly, indicating the formation of nano-aggregates. From a pure THF solution to the THF–H2O mixture with 95% or 99% water content, the emission intensities increase over 100-fold, with PL peaks at 469, 482, 466 and 487 nm for Methyl-BTPE, mMethylTPE-pTPE, oMethyl-BTPE and oMethylTPE-pTPE, respectively. The quantitative enhancement of emission was evaluated from the PL quantum yields (ΦF), using 9,10-diphenylanthracene as the standard (Fig. 3A). From a pure solution in THF to the aggregate state in 95% or 99% aqueous mixture, the ΦF values increased from nearly 0 to 63%, 89%, 58% and 62%. Moreover, the PL properties of the emitters were also investigated in the solid state. As shown in Fig. 3B, the solid films of Methyl-BTPE, mMethylTPE-pTPE, oMethyl-BTPE and oMethylTPE-pTPE exhibit blue or deep-blue emissions ranging from 457 to 485 nm, due to the introduction of methyl groups with a steric hindrance effect.
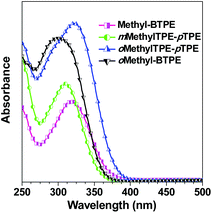 |
| Fig. 2 UV spectra of Methyl-BTPE, mMethylTPE-pTPE, oMethyl-BTPE and oMethylTPE-pTPE in THF. Concentration ∼10 μM. | |
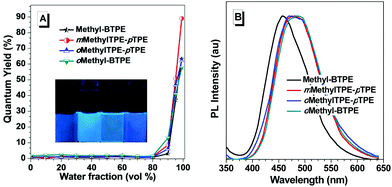 |
| Fig. 3 (A) Plots of fluorescence quantum yields for Methyl-BTPE, mMethylTPE-pTPE, oMethyl-BTPE and oMethylTPE-pTPE determined in THF/H2O solutions using 9,10-diphenylanthracene (Φ = 90% in cyclohexane) as standard versus water fractions. Inset: photos of the four luminogens in THF/water mixture (fw = 95%) taken under the illumination of a 365 nm UV lamp. (B) PL spectra of solid thin films the four luminogens. The thin films were spin-coated onto ITO glass from dilute THF solution with concentrations of 1 mg mL−1. | |
The structure–property relationship of the four compounds was further investigated using Density Functional Theory (DFT) calculations (B3LYP/6-31g*). Fig. S2† and Chart 2 show their orbital distribution and optimized molecular structures. The electron clouds in both HOMO and LUMO energy levels of Methyl-BTPE, mMethylTPE-pTPE, oMethyl-BTPE and oMethylTPE-pTPE are mainly located on the TPE units, demonstrating their weak intramolecular charge transfer. Their optical properties are closely related with the TPE skeleton. For Methyl-BTPE and mMethylTPE-pTPE, when the number of methyl groups at the 2,2′-positions changed from two to one, the dihedral angles of the biphenyl cores decreased by a large extent (from 89° to 51°), which is still higher than that of BTPE. Therefore, we can observe that on one hand, the intramolecular conjugation could be tuned by the linked methyl groups; on the other hand, if we take one TPE moiety as a whole, the conjugation degree between the two TPE units of mMethylTPE-pTPE are higher than that of Methyl-BTPE. However, for oMethyl-BTPE and oMethylTPE-pTPE with methyl groups at the 3,3′-positions, the dihedral angles of the biphenyl cores are almost the same as that of BTPE. But the tolyl groups are more twisted in reference to the double bond of TPE, showing that TPE itself is less conjugated with methyl groups at the 3,3′-positions.
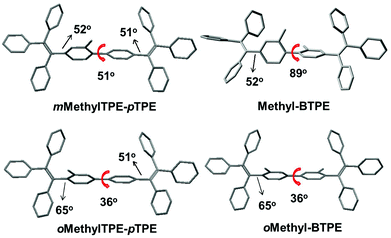 |
| Chart 2 The dihedral angles and single bond lengths between the two TPE moieties according to the optimized structures of Methyl-BTPE, mMethylTPE-pTPE, oMethyl-BTPE and oMethylTPE-pTPE. | |
Previously, we attempted to modify the conjugation lengths of the BTPE derivatives by changing the linkage modes or introducing additional groups to the 2,2′-positions of BTPE, and achieved some good results.10c,e In these two approaches, the design idea is to adjust the conjugation effect between the two pieces of TPE, for the reduction of the conjugation length of the whole BTPE molecule. However, in oMethyl-BTPE and oMethylTPE-pTPE, as discussed above, the dihedral angles of the two TPE moieties were similar to that of BTPE, thus, the conjugation effect between the two pieces of TPE should be almost the same as that in BTPE. But, with the presence of the linked methyl group at the 3-position, each TPE moiety itself became more twisted than the normal TPE one, directly leading to less conjugation effect in the TPE moiety itself, which then resulted in the decreased conjugation length of the whole molecule. This case could also be partially confirmed by the blue-shifted maximum absorption in the UV-vis spectrum of oMethyl-BTPE. Thus, perhaps, this should be another approach to modify the conjugation of TPE-based luminogens, and more work should be conducted to fully investigate the role of the linked groups at this position. In comparison with the approach of the introduction of additional groups at the 2-position, this idea could be visually shown as that in Chart 1: with the presence of the linked methyl group at the 3-position, the π structure of one TPE group changed (the decreased conjugation length), largely different from the case of Methyl-BTPE bearing the methyl group at the 2-position with a nearly unchanged conjugation length. Thus, it might be concluded that the same group could bring about a different effect even with the subtle change of a different linkage position, reminding us of the powerful, but perhaps magical power of molecular design for functional materials.
The good thermal stability and efficient solid state emission of the four Methyl-BTPE derivatives prompted us to investigate their electroluminescent properties. Non-doped light-emitting devices were fabricated with a configuration of ITO/polyethylenedioxythiophene-polystyrene sulfonic acid (PEDOT:PSS, 50 nm)/NPB (30 nm)/EML (10 nm)/TPBi (35 nm)/Ca:Ag. In these devices, PEDOT:PSS, NPB, and TPBi worked as the hole-injecting, hole-transporting, and hole-blocking layers, respectively, and Methyl-BTPE, mMethylTPE-pTPE, oMethyl-BTPE and oMethylTPE-pTPE served as the emitters. Fig. 4 shows the current density–voltage–brightness (J–V–L) characteristics, current efficiency versus current density curves and EL spectra of the OLEDs. As listed in Table 2, the device based on oMethylTPE-pTPE shows a lower turn-on voltage (3.5 V) than those of the other three compounds (4.0 V), probably due to its less twisted conformation. Generally, emitters with only one methyl group show a better device performance than those with two methylated ones. In detail, devices based on Methyl-BTPE and oMethyl-BTPE exhibit maximum luminances (Lmax) of 6289 and 8685 cd m−2, and maximum current efficiencies (ηC, max) of 2.31 and 1.57 cd A−1. oMethyl-BTPE is slightly red-shifted with an EL peak and Commission International de l'Eclairage (CIE) coordinates of 450 nm and (0.18, 0.18), compared to Methyl-BTPE with those of 442 nm and (0.17, 0.15). According to the theoretical calculation results, if we take TPE as a separate unit, when the two methyl groups are at 2,2′- or 3,3′-positions, the dihedral angle of the biphenyl core or between the tolyl group and the double bond in one TPE unit will vary accordingly. Therefore, the intramolecular conjugation could be further tuned by either changing the conjugation between two TPE moieties or of TPE itself. Based on the EL spectra and CIE data, we can see that Methyl-BTPE is less conjugated with methyl groups at 2,2′-positions.
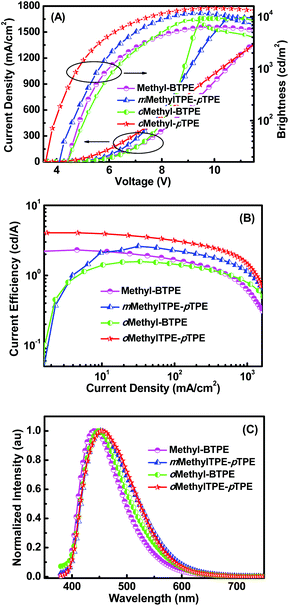 |
| Fig. 4 Current density–voltage–luminance characteristics (A), changes in current efficiency with the current density (B) and EL spectra (C) of multilayer EL devices of Methyl-BTPE, mMethylTPE-pTPE, oMethyl-BTPE and oMethylTPE-pTPE. Device configurations: ITO/MoO3 (10 nm)/NPB (60 nm)/EML (15 nm)/TPBi (35 nm)/LiF (1 nm)/Al. | |
Table 2 EL performances of Methyl-BTPE, mMethylTPE-pTPE, oMethyl-BTPE and oMethylTPE-pTPEa
|
λ
EL (nm) |
V
on (V) |
L
max (cd m−2) |
L
P, max (lm W−1) |
η
C, max (cd A−1) |
CIEb (x, y) |
Abbreviations: Von = turn-on voltage at 1 cd m−2, Lmax = maximum luminance, LP, max and ηC, max = maximum luminous power and current efficiency, respectively.
CIE = Commission International de l'Eclairage coordinates at 100 mA cm−2.
|
Methyl-BTPE |
442 |
4.0 |
6289 |
4.9 |
2.31 |
0.17, 0.15 |
mMethylTPE-pTPE |
452 |
4.0 |
11 668 |
8.4 |
2.60 |
0.18, 0.21 |
oMethyl-BTPE |
450 |
4.0 |
8685 |
6.7 |
1.57 |
0.18, 0.18 |
oMethylTPE-pTPE |
454 |
3.5 |
14 644 |
9.7 |
4.06 |
0.17, 0.21 |
Better EL performances were achieved for mMethylTPE-pTPE and oMethylTPE-pTPE with Lmax and ηC, max values of 11
668 and 14
644 cd m−2, and 2.60 and 4.06 cd A−1 respectively. Blue emissions were observed for both of the devices that were based on mMethylTPE-pTPE and oMethylTPE-pTPE, with similar CIE coordinates of (0.18, 0.21) and (0.17, 0.21). As discussed above, for mMethylTPE-pTPE and oMethylTPE-pTPE, with only one methyl group, their molecular structures are more planar than those of Methyl-BTPE and oMethylTPE-BTPE. Thus, their EL emissions are more red-shifted with CIE coordinates y > 0.20. Excitingly, we also observed that for oMethylTPE-pTPE with a methyl group at the 3-position of the biphenyl core, the maximum current efficiency increased substantially to over 4 cd A−1 with the EL emission remaining in the blue region, which is almost two times higher than that of Methyl-BTPE and oMethylTPE-BTPE. It clearly demonstrates that the intramolecular conjugation lengths and device performances could be well tuned by simply changing the number and position of additional methyl groups. When the methyl group is at the 3-position, the dihedral angle of the biphenyl core remains almost the same, but the TPE unit itself becomes more twisted, which may be more beneficial to the charge transfer and recombination. This is consistent with the lower turn-on voltage of the device that is based on oMethylTPE-pTPE. Generally, in OLEDs, amorphous films are closely related to the molecular orientation and alignment. mMethylTPE-pTPE and oMethylTPE-pTPE are less twisted than the other two molecules, thus, the charge transport may be easier in EML. On the other hand, they possess unsymmetric molecular structures which should be beneficial to the formation of amorphous films in the corresponding LED devices without pinholes.
Although there are many reported AIE emitters with very good device performance (up to ∼20 cd A−1),6c their EL spectra and CIE coordinates are seldom in the blue or deep-blue region. We focus on the design and synthesis of blue and deep-blue AIE emitters. Based on previous work with methyl, isopropyl, phenyl and carbazole groups attached to BTPE units, in this manuscript, the device performances of deep-blue AIE emitters are efficiently increased with minor red-shifted EL emissions.
Conclusion
In summary, four methyl-substituted BTPE derivatives have been successfully constructed. The intramolecular conjugation lengths have been well tuned by simply changing the number and position of methyl groups, resulting in blue or deep blue EL emissions. In comparison with the reported emitter of Methyl-BTPE (M1), the newly obtained compound of oMethylTPE-pTPE shows a better device performance with the maximum current efficiency being over 4 cd A−1, demonstrating well the instructive method of introducing methyl groups with large steric hindrance for use in constructing efficient blue and deep-blue AIE emitters. It is noteworthy to point out that the introduction of an additional group at the 3 position of TPE could twist the conformation of TPE itself to subtly adjust the conjugation effect, possibly providing a new approach to control the intramolecular conjugation of TPE-based luminogens.
Experimental
Characterization
1H and 13C NMR spectra were measured on a MECUYRVX300 spectrometer. Mass spectra were measured on a ZAB 3F-HF spectrophotometer. UV-vis absorption spectra were recorded on a Shimadzu UV-2500 recording spectrophotometer. Photoluminescence spectra were recorded on a Hitachi F-4500 fluorescence spectrophotometer. MALDI-TOF mass spectra were recorded on a GCT premier CAB048 mass spectrometer. Elemental analyses of carbon, hydrogen, and nitrogen were performed on a CARLOERBA-1106 microanalyzer. Differential scanning calorimetry (DSC) was performed on a Mettler Toledo DSC 822e at a heating and cooling rate of 15 °C min−1 from room temperature to 180 °C under argon. The glass transition temperature (Tg) was determined from the second heating scan. Thermogravimetric analysis (TGA) was undertaken with a NETZSCH STA 449C instrument. The thermal stability of the samples under a nitrogen atmosphere was determined by measuring their weight loss while heating at a rate of 10 °C min−1 from 25 to 600 °C. Cyclic voltammetry (CV) was carried out on a CHI voltammetric analyzer in a three-electrode cell with a Pt counter electrode, a Ag/AgCl reference electrode, and a glassy carbon working electrode at a scan rate of 100 mV s−1 with 0.1 M tetrabutylammonium perchlorate (purchased from Alfa Aesar) as the supporting electrolyte, in anhydrous dichloromethane solution purged with nitrogen. The potential values obtained in reference to the Ag/Ag+ electrode were converted to values versus the saturated calomel electrode (SCE) by means of an internal ferrocenium/ferrocene (Fc+/Fc) standard.
Computational details
The geometrical and electronic properties were optimized at B3LYP/6-31g(d) level using the Gaussian 09 program. The molecular orbitals were obtained at the same level of theory.
OLED device fabrication and measurement
NPB and TPBi were purchased from Aldrich Chemical Co. and were purified by thermal sublimation. In a general procedure, indium-tin oxide (ITO)-coated glass substrates were etched, patterned, and washed with detergent, deionized water, acetone, and ethanol in turn. Typical multilayer devices of ITO/polyethylenedioxythiophene–polystyrene sulfonic acid (PEDOT:PSS, 50 nm)/NPB (30 nm)/EML (10 nm)/TPBi (35 nm)/Ca:Ag (100 nm) were fabricated, where NPB and TPBi work as the hole-transporting and the hole-blocking layers, respectively. PEDOT:PSS was used to smooth the ITO surface and to decrease the hole injection barrier, and it was important to improve the device efficiency. The emitting layer thickness was 10 nm. All of the organic layers were successively deposited by means of conventional vacuum deposition onto the ITO/glass substrates at a pressure of 8 × 10−5 Pa. The active area of the device was 6 mm2. A quartz crystal oscillator placed near the substrate was used to monitor the thickness of each layer, which was calibrated ex situ using an Ambios Technology XP-2 surface profilometer. EL spectra and chromaticity coordinates were measured with a SpectraScan PR 655 photometer. All device tests were carried out under an ambient atmosphere at room temperature. Current density–voltage–luminance (J–V–L) measurements were conducted simultaneously using a Keithley 4200 semiconductor parameter analyzer combined with a Newport multifunction 2835-C optical meter, with luminance being measured in the forward direction.
Preparation of compounds
All other chemicals and reagents were obtained from commercial sources and used as received without further purification. Solvents for chemical synthesis were purified according to the standard procedures. Compounds 1, 3, 5, 7, 9 and Methyl-BTPE were synthesized according to the reported literature.10
Synthesis of compound 4
Compound 2 was added to a solution of p-toluenesulfonic acid in acetonitrile under vigorous stirring. The suspension was cooled to 0 °C, and the aqueous solution of sodium nitrite and potassium iodide was added dropwise. The mixture was stirred for 30 min at 0 °C and then was warmed to room temperature. The reaction was terminated by adding water. The mixture was extracted using dichloromethane, and the organic layer was combined and washed with saturated sodium hydrogen sulfite three times and dried with anhydrous sodium sulfate. After filtration and solvent evaporation, the crude product was purified by silica gel column chromatography using petroleum ether as eluent. Colorless oil was obtained at a yield of 65%. 1H NMR (300 MHz, CDCl3) δ (ppm): 7.60–7.56 (m, 1H), 7.21–7.17 (m, 2H), 2.35 (s, 3H).
Synthesis of compound 6
A solution of iPrMgCl (1.3 M, 11.5 mL, 15 mmol) in THF was added dropwise to compound 4 (4.02 g, 13.5 mmol) in THF (40 mL) under N2 at −78 °C. The mixture was stirred at −78 °C for 3 h. A solution of CuCN·2LiCl (15 mL, 14.9 mmol) in THF and benzoyl chloride (2.30 g, 16.3 mmol) were added successively at −78 °C. The mixture was warmed to room temperature overnight. After hydrolysis with saturated NH4Cl, the mixture was extracted with CH2Cl2 (3 × 50 mL). The combined organic layers were dried over Na2SO4 and evaporated under reduced pressure. The crude product was purified by flash chromatography using petroleum ether/dichloromethane as eluent to obtain a white solid at a yield of 50%. 1H NMR (300 MHz, CDCl3) δ (ppm): 7.78–7.75 (m, 2H), 7.66–7.57 (m, 3H), 7.50–7.44 (m, 3H), 2.47 (s, 3H).
Synthesis of compound 8
A 2.2 M solution of n-butyllithium in hexane (2.04 M, 1.2 mL, 2.4 mmol) was added to a solution of diphenylmethane (505 mg, 3 mmol) in anhydrous tetrahydrofuran (10 mL) at 0 °C under a N2 atmosphere. After stirring for 1 h at this temperature, benzophenone derivative 6 (551 mg, 2 mmol) was added. After 2 h, the mixture was slowly warmed to room temperature. Then, the reaction was quenched with an aqueous solution of ammonium chloride and the mixture was extracted with dichloromethane. The organic layer was evaporated after drying with anhydrous sodium sulfate and the resultant crude product was dissolved in toluene (30 mL). The p-toluenesulfonic acid (70 mg, 0.4 mmol) was added, the mixture was refluxed overnight and cooled to room temperature. The mixture was then evaporated and the crude product was purified by silica gel column chromatography using petroleum ether as eluent to obtain a white powder at a yield of 60%. 1H NMR (300 MHz, CDCl3) δ (ppm): 7.18–7.13 (m, 4H), 7.11–7.05 (m, 9H), 6.94–6.91 (m, 5H), 2.04 (s, 3H).
Synthesis of compound 10
A 2.2 M solution of n-butyllithium in hexane (2.2 M, 0.25 mL, 1.08 mmol) was added to a solution of compound 8 (305 mg, 0.72 mmol) in anhydrous tetrahydrofuran (10 mL) at −78 °C under a N2 atmosphere. After stirring for 4 h, 2-isopropoxy-4,4,5,5-tetramethyl-1,3,2-dioxaborolane (1.53 mL, 1.44 mmol) was added. After 2 h, the mixture was slowly warmed to room temperature. After stirring overnight, the reaction was terminated by adding brine. The mixture was extracted with dichloromethane and the organic layer was combined, and dried with anhydrous sodium sulfate. After filtration and solvent evaporation, the crude product was purified by silica gel column chromatography using petroleum ether/dichloromethane as eluent. White powder was obtained at a yield of 85%. 1H NMR (300 MHz, CDCl3) δ (ppm): 7.48–7.42 (m, 2H), 7.13–7.03 (m, 13H), 6.95–6.94 (m, 4H), 2.08 (s, 3H), 1.33 (s, 12H).
General procedures for the synthesis of mMethylTPE-pTPE and oMethyl-BTPE, oMehtylTPE-pTPE
All of the compounds were synthesized using a similar procedure to the one shown below in 50–80% yields. A mixture of aryl bromide (7, 8 or pTPE-Br) (1 mmol), aryl boronic ester (9 or 10) (1 mmol), Pd(PPh3)4 (20 mg) and potassium hydroxide (5 mmol) in 15 mL of THF and 5 mL of distilled water in a 50 ml Schlenk tube was refluxed for 2 days under N2. The mixture was extracted with dichloromethane. The combined organic extracts were dried over anhydrous Na2SO4 and concentrated by rotary evaporation. The crude product was purified by column chromatography on silica gel using petroleum ether/dichloromethane as eluent.
mMethylTPE-pTPE.
1H NMR (300 MHz, CDCl3) δ (ppm): 7.10–7.05 (m, 29H), 7.04–7.01 (m, 5H), 6.91–6.85 (m, 3H), 2.02 (s, 3H). 13C NMR (75 MHz, CDCl3) δ (ppm): 144.0, 143.9, 143.8, 142.5, 142.2, 141.2, 141.0, 140.9, 139.9, 134.5, 133.5, 131.6, 131.1, 129.1, 129.0, 128.7, 127.8, 126.6, 20.6. HRMS (ESI, m/z): [M + H]+ for C53H41, 677.3210; found, 677.3208. Anal. Calcd for C53H40: C, 94.04; H, 5.96. Found: C, 93.85; H, 5.85.
oMethylTPE-pTPE.
1H NMR (300 MHz, CDCl3) δ (ppm): 7.33–7.26 (m, 5H), 7.04–7.01 (m, 5H), 7.13–7.04 (m, 28H), 7.00–6.97 (m, 4H), 2.10 (s, 3H). 13C NMR (75 MHz, CDCl3) δ (ppm): 144.0, 143.8, 143.6, 142.9, 142.8, 142.3, 142.0, 141.2, 140.8, 140.0, 138.9, 138.6, 137.1, 132.4, 131.9, 131.6, 130.9, 130.8, 128.6, 128.0, 127.9, 127.7, 126.7, 126.5, 126.1, 124.0, 20.7. HRMS (ESI, m/z): [M + Na]+ for C53H40Na, 699.3028; found, 699.3064. Anal. Calcd for C53H40: C, 94.04; H, 5.96. Found: C, 94.30; H, 6.08.
oMethyl-BTPE.
1H NMR (300 MHz, CDCl3) δ (ppm): 7.26–7.20 (m, 5H), 7.14–7.13 (m, 7H), 7.09–7.04 (m, 16H), 7.01–6.95 (m, 8H), 2.10 (s, 6H). 13C NMR (75 MHz, CDCl3) δ (ppm): 144.5, 144.3, 143.7, 142.9, 142.6, 140.8, 139.8, 137.8, 133.1, 132.4, 131.7, 131.5, 129.4, 128.7, 128.5, 128.4, 127.5, 127.2, 124.7, 21.4. HRMS (ESI, m/z): [M + H]+ for C53H43, 691.3358; found, 691.3365. Anal. Calcd for C54H42: C, 93.87; H, 6.13. Found: C, 94.05; H, 6.02.
Acknowledgements
We are grateful to the National Fundamental Key Research Program (2013CB834701) and the National Natural Science Foundation of China (no. 21325416, 61106017) for financial support.
Notes and references
-
(a) C. W. Tang and S. A. Vanslyke, Appl. Phys. Lett., 1987, 51, 913 CrossRef CAS PubMed;
(b) C. Adachi, T. Tsutsui and S. Saito, Appl. Phys. Lett., 1989, 55, 1489 CrossRef CAS PubMed;
(c) M. A. Baldo, M. E. Thompson and S. R. Forrest, Nature, 2000, 403, 750 CrossRef CAS PubMed;
(d) B. W. D'Andrade and S. R. Forrest, Adv. Mater., 2004, 16, 1585 CrossRef PubMed;
(e) P. L. Burn, S. C. Lo and I. D. W. Samuel, Adv. Mater., 2007, 19, 1675 CrossRef CAS PubMed.
-
(a) K. C. Wu, P. J. Ku, C. S. Lin, H. T. Shih, F. I. Wu, M. J. Huang, J. J. Lin, I. C. Chen and C. H. Cheng, Adv. Funct. Mater., 2008, 18, 67 CrossRef CAS PubMed;
(b) I. Cho, S. H. Kim, J. H. Kim, S. Park and S. Y. Park, J. Mater. Chem., 2012, 22, 123 RSC;
(c) S. H. Kim, I. Cho, M. K. Sim, S. Park and S. Y. Park, J. Mater. Chem., 2011, 21, 9139 RSC;
(d) H. Park, J. Lee, I. Kang, H. Y. Chu, J. I. Lee, S. K. Kwon and Y. H. Kim, J. Mater. Chem., 2012, 22, 2695 RSC;
(e) L. M. Leung, W. Y. Lo, S. K. So, K. M. Lee and W. K. Choi, J. Am. Chem. Soc., 2000, 122, 5640 CrossRef CAS;
(f) Y. Y. Lyu, J. Kwak, O. Kwon, S. H. Lee, D. Kim, C. Lee and K. Char, Adv. Mater., 2008, 20, 2720 CrossRef CAS PubMed;
(g) R. J. Tseng, R. C. Chiechi, F. Wudl and Y. Yang, Appl. Phys. Lett., 2006, 88, 093512 CrossRef PubMed;
(h) T. P. I. Saragi, T. Spehr, A. Siebert, T. Fuhrmann-Lieker and J. Salbeck, Chem. Rev., 2007, 107, 1011 CrossRef CAS PubMed.
-
(a) L. H. Chan, R. H. Lee, C. F. Hsieh, H. C. Yeh and C. T. Chen, J. Am. Chem. Soc., 2002, 124, 6469 CrossRef CAS PubMed;
(b) M. T. Lee, C. H. Liao, C. H. Tasi and C. H. Chen, Adv. Mater., 2005, 17, 2493 CrossRef CAS PubMed;
(c) B. C. Krummacher, V. E. Choong, M. K. Mathsi, S. A. Choulis, F. Jermann, T. Fiedler and M. Zachau, Appl. Phys. Lett., 2006, 88, 113506 CrossRef PubMed;
(d) R. C. Chiechi, R. J. Tseng, F. Marchioni, Y. Yang and F. Wudl, Adv. Mater., 2006, 18, 325 CrossRef CAS PubMed;
(e) K. T. Kamtekar, A. P. Monkman and M. R. Bryce, Adv. Mater., 2010, 22, 572 CrossRef CAS PubMed;
(f) Y.-H. Kim, H.-C. Jeong, S.-H. Kim, K. Yang and S.-K. Kwon, Adv. Funct. Mater., 2005, 15, 1799 CrossRef CAS PubMed.
-
(a) S. W. Thomas III, G. D. Joly and T. M. Swager, Chem. Rev., 2007, 107, 1339 CrossRef PubMed;
(b) A. C. Grimsdale, K. L. Chan, R. E. Martin, P. G. Jokisz and A. B. Holmes, Chem. Rev., 2009, 109, 897 CrossRef CAS PubMed.
-
(a) M. T. Lee, H. H. Chen, C. H. Liao, C. H. Tsai and C. H. Chen, Appl. Phys. Lett., 2004, 85, 3301 CrossRef CAS PubMed;
(b) P. F. Wang, Z. R. Hong, Z. Y. Xie, S. W. Tong, O. Y. Wong, C. S. Lee, N. B. Wong, L. S. Hung and S. T. Lee, Chem. Commun., 2003, 1664 RSC;
(c) S. Hecht and J. M. J. Frechet, Angew. Chem., Int. Ed., 2001, 40, 74 CrossRef CAS;
(d) C. Fan, S. Wang, J. W. Hong, G. C. Bazan, K. W. Plaxco and A. J. Heeger, Proc. Natl. Acad. Sci. U. S. A., 2003, 100, 6297 CrossRef CAS PubMed;
(e) S.-F. Lim, R. H. Friend, I. D. Rees, J. Li, Y. Ma, K. Robinson, A. B. Holms, E. Hennebicq, D. Beljonne and F. Cacialli, Adv. Funct. Mater., 2005, 15, 98 CrossRef PubMed.
-
(a) J. Luo, Z. Xie, J. W. Y. Lam, L. Cheng, H. Chen, C. Qiu, H. S. Kwok, X. Zhan, Y. Liu, D. Zhu and B. Z. Tang, Chem. Commun., 2001, 1740 RSC;
(b) Y. Dong, J. W. Y. Lam, A. Qin, J. Liu, Z. Li and B. Z. Tang, Appl. Phys. Lett., 2007, 91, 011111 CrossRef PubMed;
(c) H. Chen, W. Y. Lam, J. Luo, Y. L. Ho, B. Z. Tang, D. Zhu, M. Wong and H. S. Kwok, Appl. Phys. Lett., 2002, 81, 547 CrossRef PubMed;
(d) B. K. An, S. K. Kwon, S. D. Jung and S. Y. Park, J. Am. Chem. Soc., 2002, 124, 14410 CrossRef CAS PubMed; B. K. An, J. Gierschner and S. Y. Park, Acc. Chem. Res., 2012, 45, 544 Search PubMed;
(e) D. Oelkrug, A. Tompert, J. Gierschner, H. J. Egelhaaf, M. Hanack, M. Hohloch and E. Steinhuber, J. Phys. Chem. B, 1998, 102, 1902 CrossRef CAS;
(f) F. Würthner, R. Sens, K. H. Etzbach and G. Seybold, Angew. Chem., Int. Ed., 1999, 38, 1649 CrossRef;
(g) W. S. Xia, R. H. Schmehl and C. J. Li, Chem. Commun., 2000, 695 RSC;
(h) Z. Li and A. Qin, Natl. Sci. Rev., 2014, 1, 22 CrossRef PubMed.
-
(a) J. Chen, B. Xu, X. Ouyang, B. Z. Tang and Y. Cao, J. Phys. Chem. A, 2004, 108, 7522 CrossRef CAS;
(b) Z. Li, Y. Dong, B. Mi, Y. Tang, H. Tong, P. Dong, J. W. Y. Lam, Y. Ren, H. H. Y. Sun, K. Wong, P. Gao, I. D. Williams, H. S. Kwok and B. Z. Tang, J. Phys. Chem. B, 2005, 109, 10061 CrossRef CAS PubMed;
(c) Z. Ning, Z. Chen, Q. Zhang, Y. Yan, S. Qian, Y. Cao and H. Tian, Adv. Funct. Mater., 2007, 17, 3799 CrossRef CAS PubMed;
(d) D. Shultz and M. A. Fox, J. Am. Chem. Soc., 1989, 111, 6311 CrossRef CAS;
(e) M. A. Fox and D. Shultz, J. Org. Chem., 1988, 53, 4386 CrossRef CAS;
(f) Y. P. Sun and M. A. Fox, J. Am. Chem. Soc., 1993, 115, 747 CrossRef CAS;
(g) M. O. Wolf, H. H. Fox and M. A. Fox, J. Org. Chem., 1996, 61, 287 CrossRef CAS;
(h) J. L. Muzyka and M. A. Fox, J. Org. Chem., 1991, 56, 4549 CrossRef CAS.
- Z. Zhao, J. W. Y. Lam and B. Z. Tang, J. Mater. Chem., 2012, 22, 23726 RSC.
-
(a) S. K. Kim, Y. Park, I. N. Kang and J. W. Park, J. Mater. Chem., 2007, 17, 4670 RSC;
(b) P. Shih, C. Y. Chuang, C. H. Chien, E. W. G. Diau and C. F. Shu, Adv. Funct. Mater., 2007, 17, 3141 CrossRef CAS PubMed;
(c) Y. Hong, J. W. Y. Lam and B. Z. Tang, Chem. Soc. Rev., 2011, 40, 5361 RSC;
(d) C. Y. K. Chan, Z. Zhao, J. W. Y. Lam, J. Liu, S. Chen, P. Lu, F. Mahtab, X. Chen, H. H. Y. Sung, H. S. Kwok, Y. Ma, I. D. Williams, K. S. Wong and B. Z. Tang, Adv. Funct. Mater., 2012, 22, 378 CrossRef CAS PubMed;
(e) B. He, S. Ye, Y. Guo, B. Chen, X. Xu, H. Qiu and Z. Zhao, Sci. China: Chem., 2013, 56, 1221 CrossRef CAS;
(f) K. Li, D. Ding, Q. Zhao, J. Sun, B. Tang and B. Liu, Sci. China: Chem., 2013, 56, 1228 CrossRef CAS.
-
(a) J. Huang, X. Yang, J. Wang, C. Zhong, L. Wang, J. Qin and Z. Li, J. Mater. Chem., 2012, 22, 2478 RSC;
(b) J. Huang, N. Sun, J. Yang, R. Tang, Q. Li, D. Ma, J. Qin and Z. Li, J. Mater. Chem., 2012, 22, 12001 RSC;
(c) J. Huang, N. Sun, Y. Dong, R. Tang, P. Lu, P. Cai, Q. Li, D. Ma, J. Qin and Z. Li, Adv. Funct. Mater., 2013, 23, 2329 CrossRef CAS PubMed;
(d) J. Huang, N. Sun, J. Yang, R. Tang, Q. Li, D. Ma and Z. Li, Adv. Funct. Mater., 2014, 24, 7645 CrossRef CAS PubMed;
(e) J. Huang, N. Sun, P. Chen, R. Tang, Q. Li, D. Ma and Z. Li, Chem. Commun., 2014, 50, 2136 RSC;
(f) J. Huang, P. Chen, X. Yang, R. Tang, L. Wang, J. Qin and Z. Li, Sci. China: Chem., 2013, 56, 1213 CrossRef CAS.
-
(a) K. R. Wee, W. S. Han, J. E. Kim, A. L. Kim, S. Kwon and S. O. Kang, J. Mater. Chem., 2011, 21, 1115 RSC;
(b) K. Traskovskis, I. Mihailovs, A. Tokmakovs, A. Jurgis, V. Kokars and M. Rutkis, J. Mater. Chem., 2012, 22, 11268 RSC.
Footnote |
† Electronic supplementary information (ESI) available: AIE curves and calculated molecular orbital amplitude plots of HOMO and LUMO levels and optimized molecular structures for Methyl-BTPE, mMethylTPE-pTPE, oMethyl-BTPE and oMethylTPE-pTPE respectively. See DOI: 10.1039/c5qo00274e |
|
This journal is © the Partner Organisations 2015 |