DOI:
10.1039/C5QI00157A
(Research Article)
Inorg. Chem. Front., 2015,
2, 1085-1090
Tuning oxygen-sensing behaviour of a porous coordination framework by a guest fluorophore†
Received
21st August 2015
, Accepted 25th September 2015
First published on 29th September 2015
Abstract
Tris(8-hydroxyquinolinato)aluminium (AlQ3), a classic fluorescent molecule insensitive to oxygen, is encapsulated as a guest fluorophore into a highly porous coordination framework [Zn4O(bpz)2(bdc)] (MAF-X10, H2bpz = 3,3′,5,5′-tetramethyl-4,4′-bipyrazole, H2bdc = 1,4-benzenedicarboxylic acid) to obtain a highly luminescent host–guest hybrid material AlQ3@MAF-X10 combining the advantages of excellent fluorescence of AlQ3, high oxygen permeability of MAF-X10, and the resonance energy transfer between AlQ3 and MAF-X10. Photoluminescence studies showed that AlQ3@MAF-X10 exhibits yellowish green fluorescence with a Stokes shift (9606 cm−1) as large as those of phosphorescent noble metal complexes, long lifetime (37.4 ns) and a considerable oxygen permeability (4.4 × 10−9 mol cm−1 s−1 bar−1), giving rise to high oxygen sensitivity (88.5% quenched at 1 bar, i.e., I0/I100 = 8.7, KSV = 7.6 bar−1) with fast response and good reversibility.
Introduction
Luminescent porous coordination polymers (PCPs) or metal–organic frameworks (MOFs)1–8 are emerging as unique optical oxygen-sensing materials because of their diversified excitation/emission properties coupled with high oxygen permeabilities.5,9–14 Using phosphorescent coordination complexes as chromophores, luminescent PCPs can achieve high quantum yields, long lifetimes and large Stokes shifts (Scheme 1a), giving moderate to high oxygen sensitivity.15–20 However, precious-metal ions such as Pt, Pd, Ru, Au, Ir, Re are generally necessary for phosphorescence emission.21
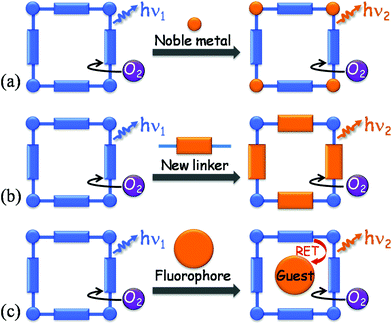 |
| Scheme 1 Strategies for tuning the output signal (blue and orange arrows represent relatively short and long emission wavelengths, respectively) of luminescent oxygen sensing materials. (a) Replacing conventional metal ions with noble metal ions, (b) increasing the π-conjugation of organic linkers, and (c) encapsulating guest fluorophores. | |
Recently, we found that some noble-metal-free fluorescent PCPs can be used as oxygen-sensing materials, among which, [Zn4O(bpz)2(abdc)] (MAF-X11, H2bpz = 3,3′,5,5′-tetramethyl-4,4′-bipyrazole, H2abdc = 2-amino-1,4-benzenedicarboxylic acid) shows exceptionally high sensitivity due to its high oxygen permeability as well as a relatively long lifetime of ca. 14 ns.10 However, most of the other fluorescent PCPs, such as [Zn4O(bpz)2(bdc)] (MAF-X10, H2bdc = 1,4-benzenedicarboxylic acid), [Zn4O(bdc)3] (IRMOF-1), and [Zn4O(abdc)3] (IRMOF-3), which are analogues of MAF-X11, often show unnoticeable O2 quenching properties due to their weak emission intensities and/or near ultraviolet emission colors. For example, the emission maxima of MAF-X10 and IRMOF-1 are located at ca. 400 nm.10 Also, fluorescent oxygen-sensing materials, including PCPs and organic molecules immobilized in porous matrices, usually show small Stokes shifts between the excitation and emission bands, which are disadvantageous to avoid interference between the excitation and emission lights and complicate device configurations.22–24
Although increasing the π-conjugation of organic linkers can give red-shifted emissions (Scheme 1b),25 such a strategy is complicated for the design/synthesis of new organic ligands and PCPs. Fortunately, many cheap and well-studied fluorescent molecules can be embedded inside porous matrices,26,27 such as zeolites,28–32 organic polymers33–36 and PCPs.37–46 In this context, the resonance energy transfer (RET) mechanism between different chromophores may be utilized in these host–guest systems to obtain the desirable excitation/emission characteristics (Scheme 1c). Herein, we show that such a strategy can lead to new oxygen-sensing materials combining the advantages of both the host and guest fluorophores.
Experimental section
Materials and methods
Commercially available reagents were used as received without further purification. Elemental analyses were performed with an Elementar Vario EL analyzer. Infrared (IR) spectra were recorded on a Bruker Tensor 27 FT-IR spectrometer in the 400–4000 cm−1 region with KBr pellets. Powder X-ray diffraction (PXRD) patterns were recorded on a Bruker D8-Advance diffractometer with Cu Kα radiation and a LynxEye detector. Thermogravimetry (TG) analyses were performed on a TA Q50 thermogravimetric analyzer with a heating rate of 10 °C min−1 under nitrogen flow. Gas sorption isotherms were measured by using an automatic volumetric adsorption apparatus (Micromeritics ASAP 2020M). Ultrahigh-purity-grade (99.999%) gases were used for all measurements. The as-synthesized sample (weight 100 mg) was placed in the sample holder and dried for 8 h at 393 K to remove the remnant solvent molecules prior to measurement. The measurement temperatures were controlled by using a liquid-nitrogen bath (77 K) or a water bath (298 K). Inductive coupled plasma (ICP) analysis was performed with a PerkinElmer Optima 8300 spectrometer. The as-synthesized sample was digested in HNO3 at 150 °C for 10 min prior to ICP analysis.
Photoluminescence measurement
Steady state photoluminescence spectra and lifetime measurements were performed on an Edinburgh FLS920 spectrometer equipped with a continuous Xe900 Xenon lamp and a 405 nm laser flash lamp. All instrument parameters such as excitation split, emission split, and scanning speed were fixed during the in situ measurements. The oxygen responses of photoluminescence were measured via the “Kinetic scan” module in a sealed chamber equipped with quartz windows and a three-way valve which connects the chamber to a vacuum pump and an O2 cylinder.
Synthesis
AlQ3@MAF-X10.
MAF-X10 and AlQ3 were synthesized according to the literature methods.47–49 MAF-X10 (100 mg) was immersed in a saturated ethanol solution of AlQ3 (5 mmol L−1, 10 mL) and kept at 100 °C for 48 hours. After being cooled to room temperature, the resulting yellow microcrystals were filtered, then washed with ethanol (3 × 2 mL) and dried in air (yield 112 mg). Elemental analysis: Calcd for [Zn4O(bpz)2(bdc)]·0.34AlQ3·5H2O (C37.18H44.12Al0.34Zn4N9.02O11.02): C 41.95, H 4.18, N 11.87. Found: C 42.24, H 3.86, N 11.59%. IR (KBr): 3412m, 2964m, 2924m, 1581vs, 1501m, 1427m, 1379vs, 1136m, 1093w, 1052s, 884w, 823m, 753m, 705w, 516m cm−1.
Results and discussion
Design and synthesis
MAF-X10 is a non-interpenetrated pcu type coordination network, being isostructural with MAF-X11 and isoreticular with IRMOF-1 and IRMOF-3.10,48–50 Similar to its analogues, MAF-X10 is highly porous (void = 63.4%) with a BET surface area of over 2000 m2 g−1.49 MAF-X10 possesses an effective aperture size of 5.8 × 6.6 Å2 and a cavity size of 9.4 × 9.9 × 13.2 Å3 (excluding van der Waals radii). Tris(8-hydroxyquinolinato)aluminium (AlQ3), with a molecular diameter of ca. 8–9 Å,37,38 is a well-studied fluorescent material showing high quantum yield. Grand Canonical Monte Carlo (GCMC) simulations showed that AlQ3 can be accommodated into the cavity of MAF-X10 (Fig. 1). More importantly, the excitation maximum of AlQ3 is located at 395 nm,51 meaning that a RET process from MAF-X10 (an emission maximum at ca. 400 nm) to AlQ3 is possible, because it was described that RET can occur if the emission spectrum of the donor overlaps the excitation spectrum of the acceptor.52 When O2 molecules quench the fluorescence of the MAF-X10 host framework of the host–guest inclusion compound AlQ3@MAF-X10, the visible effect would be the decreased fluorescence of the AlQ3 guest molecules. In other words, the signaling function of MAF-X10 will be replaced by AlQ3.
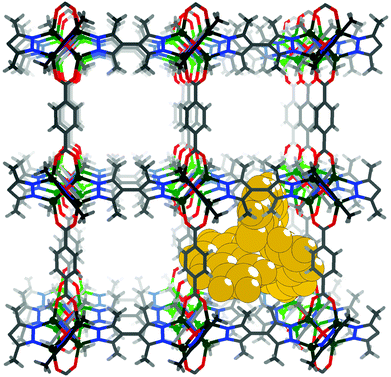 |
| Fig. 1 Preferential AlQ3 location in MAF-X10 obtained from GCMC calculations. | |
Colorless microcrystals of MAF-X10·EtOH (ethanol as the guest) were synthesized according to the previously reported method (Fig. S1†).49 AlQ3@MAF-X10 was prepared by immersing the PCP microcrystals into a saturated ethanol solution of AlQ3 at 100 °C for two days. After the treatment, the microcrystals retain the original morphology but turn yellow. PXRD showed that the yellow microcrystals contain only the characteristic diffraction peaks of MAF-X10 with some changes in relative peak intensities, and no extra peak was observed for AlQ3 or any other impurities (Fig. S1†). TG showed that MAF-X10 and AlQ3@MAF-X10 can maintain framework integrity until ca. 550 °C (Fig. S2†). ICP analysis of acid-digested AlQ3@MAF-X10 showed an Al/Zn molar ratio of ca. 0.084, corresponding to a chemical formula of [Zn4O(bpz)2(bdc)]·0.34AlQ3 (average 0.34 AlQ3 per cavity), which was consistent with the results of elemental analysis. Encapsulation of guests with molecular sizes larger than the aperture size of the host has been observed for other PCPs, for example, in the analogous compound [Zn4O(carboxypyrazolato)3].53,54
N2 sorption isotherms measured at 77 K showed that AlQ3@MAF-X10 (Fig. 2 and S3†) possesses Langmuir/BET surface areas of 1575/1370 m2 g−1 and a total pore volume of 0.554 cm3 g−1, which are ca. 30% lower than those of MAF-X10 (2239/2032 m2 g−1 and 0.798 cm3 g−1). Horvath–Kawazoe calculations of the N2 isotherms of AlQ3@MAF-X10 and MAF-X10 indicated pore-diameter distributions both centered at ca. 7.2 Å (Fig. S3†), indicating that the unoccupied cavities in AlQ3@MAF-X10 are basically the same as in MAF-X10.
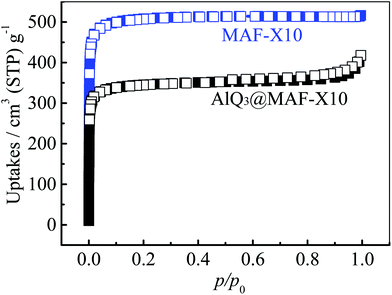 |
| Fig. 2 N2 adsorption (solid) and desorption (open) isotherms at 77 K for MAF-X10 and AlQ3@MAF-X10. | |
Photoluminescence and oxygen-sensing properties
As reported previously, MAF-X10 exhibits weak bluish fluorescence with a maximum at 407 nm, which is difficult to identify with the naked eye,10 while AlQ3 exhibits strong yellowish green fluorescence centered at 520 nm. Under UV light, AlQ3@MAF-X10 shows bright yellowish green photoluminescence centered at 516 nm (Fig. S4†), which is similar to that of AlQ3 and considerably red-shifts 109 nm from that of MAF-X10 (Fig. 3). Accordingly, the Stokes shift between the excitation (345 nm) and emission peaks of 9606 cm−1 for AlQ3@MAF-X10 is comparable with those of many other PCPs based on phosphorescent noble metal complexes.13 The fluorescence spectra of AlQ3@MAF-X10 are similar to those of AlQ3 rather than MAF-X10, implying that the fluorescence emission of AlQ3@MAF-X10 originates from AlQ3. Notably, there is no peak at ca. 407 nm in the emission spectra of AlQ3@MAF-X10, indicating that the RET from MAF-X10 (donor) to AlQ3 (acceptor) is very efficient.52 The luminescence lifetime of AlQ3@MAF-X10 was measured as ca. 37.4 ns (Fig. S5†), which is much longer than that of MAF-X10 (3.45 ns) and slightly longer than that of AlQ3 (12–32 ns).37,38 The increased fluorescence lifetime of the AlQ3 molecule is related to the high fluorescence intensity of AlQ3@MAF-X10 and can be explained by the reduction of non-radiative decays after confinement of AlQ3 in the host framework MAF-X10.
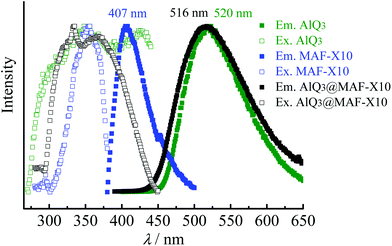 |
| Fig. 3 Photoluminescence spectra of crystalline AlQ3, MAF-X10 and AlQ3@MAF-X10. | |
The emission spectra of the activated MAF-X10 and AlQ3@MAF-X10 under different O2 pressures (from vacuum to 1 bar) were recorded (Fig. 4a), whose intensities were found to decrease with linear relationships against the O2 pressure. At 1 bar O2, 84.5% (I0/I100 = 6.5) and 88.5% (I0/I100 = 8.7) emission intensities were quenched for MAF-X10 and AlQ3@MAF-X10, respectively. Linear fitting of the quenching data at different pressures gave the Stern–Volmer constants Ksv (slope) of 5.2 and 7.6 bar−1 for MAF-X10 and AlQ3@MAF-X10, respectively (Fig. 4b), which are larger than those of many PCPs based on phosphorescent noble metal complexes,16–20 though lower than those of MAF-X11 and the copper(I) triazolate framework MAF-2.10,13 The good linearity of the Stern–Volmer plot of AlQ3@MAF-X10 indicated homogeneous distribution of AlQ3 molecules within MAF-X10. Further, the kinetic fluorescent responses of MAF-X10 and AlQ3@MAF-X10 were continuously recorded with stepwise changes and cycling of O2 pressures between 0 and 1 bar, which showed instant changes of emission intensity (Fig. 5 and S6†), indicating that the quenching responses are rapid, reversible and highly stable.
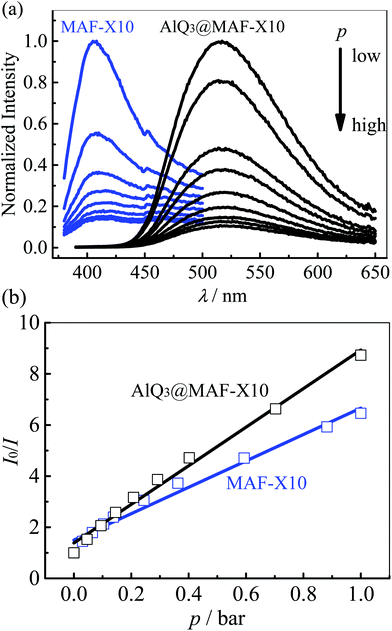 |
| Fig. 4 (a) O2 pressure dependent emission spectra and (b) the corresponding Stern–Volmer plots (the line represents the linear fitting) for MAF-X10 and AlQ3@MAF-X10. | |
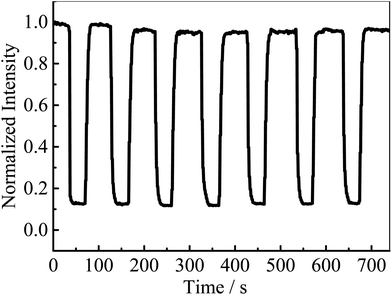 |
| Fig. 5 Fluorescence intensity of AlQ3@MAF-X10 in a repeatedly alternating O2 (1 bar) and vacuum environment. | |
Oxygen adsorption and diffusion properties
Oxygen permeability and luminescence lifetime of the luminescent material are key factors that affect the oxygen quenching sensitivity.10,55 The O2 sorption experiments were carried out at 298 K for MAF-X10 and AlQ3@MAF-X10, which showed linear and reversible isotherms (Fig. 6), indicating weak host–guest interactions between the highly porous host and the low-polarity gas. Nevertheless, their O2 uptakes reach 5.5 and 4.6 cm3(STP) g−1 at 1 bar, being equivalent to a solubility of 0.20 and 0.19 mol L−1, respectively, which are comparable with those of the most oxygen-sensitive PCPs, such as MAF-X11 (0.21 mol L−1) and MAF-2 (0.12 mol L−1).10,13
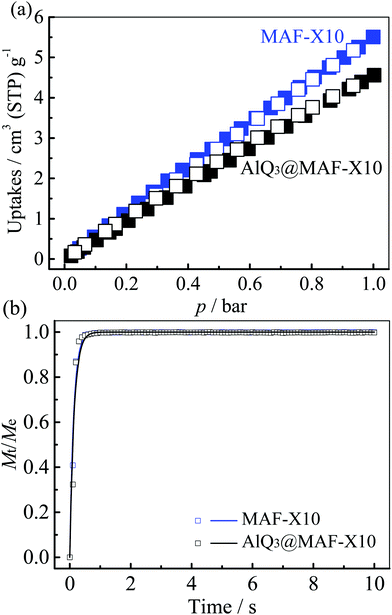 |
| Fig. 6 (a) O2 adsorption (solid) and desorption (open) isotherms at 298 K and (b) the corresponding kinetic profiles (the line represents the exponential fitting) for MAF-X10 and AlQ3@MAF-X10. | |
Further, the O2 adsorption/desorption show very fast kinetics and can reach equilibrium within 1 second. Fitting the kinetic profiles of O2 adsorption at 298 K gave large diffusion coefficients of 2.5 × 10−5 and 2.3 × 10−5 cm2 s−1 for MAF-X10 and AlQ3@MAF-X10, respectively (Fig. 6b, S7 and S8†). Therefore, their oxygen permeabilities can be calculated as 5.0 × 10−9 and 4.4 × 10−9 mol cm−1 s−1 bar−1, respectively, which are similar to those of the most oxygen-sensitive PCPs.10,13 The slightly smaller O2 adsorption ability and slower diffusion rate of AlQ3@MAF-X10 can be ascribed to the reduction of porosity by the AlQ3 molecules. Obviously, the difference in the oxygen permeabilities between MAF-X10 and AlQ3@MAF-X10 is smaller than that of their fluorescence lifetimes. Therefore, the significantly longer lifetime of AlQ3@MAF-X10 accounts for its slightly higher oxygen-sensing efficiency.
Conclusions
In summary, we demonstrated that the luminescence properties of an oxygen-sensing PCP can be facilely modified by encapsulating the AlQ3 molecule as the guest fluorophore to increase the Stokes shift and oxygen sensitivity. Preliminary experiments using other oxygen-sensing PCPs and oxygen-insensitive fluorescent molecules, such as MAF-X11 and acriflavinium chloride, were also successful. This strategy may open a new perspective for future design and fabrication of novel optical oxygen-sensing materials.
Acknowledgements
This work was supported by the “973 Project” (2014CB845602 and 2012CB821706) and NSFC (21225105, 21290173, and 21473260).
Notes and references
- N. Yanai, K. Kitayama, Y. Hijikata, H. Sato, R. Matsuda, Y. Kubota, M. Takata, M. Mizuno, T. Uemura and S. Kitagawa, Nat. Mater., 2011, 10, 787–793 CrossRef CAS PubMed.
- C. Y. Sun, X.-L. Wang, X. Zhang, C. Qin, P. Li, Z.-M. Su, D.-X. Zhu, G.-G. Shan, K.-Z. Shao, H. Wu and J. Li, Nat. Commun., 2013, 4, 2717 Search PubMed.
- S. S. Nagarkar, B. Joarder, A. K. Chaudhari, S. Mukherjee and S. K. Ghosh, Angew. Chem., Int. Ed., 2013, 52, 2881–2885 CrossRef CAS PubMed.
- Y. Kang, F. Wang, J. Zhang and X.-H. Bu, J. Am. Chem. Soc., 2012, 134, 17881–17884 CrossRef CAS PubMed.
- Y. J. Cui, Y.-F. Yue, G.-D. Qian and B.-L. Chen, Chem. Rev., 2012, 112, 1126–1162 CrossRef CAS PubMed.
- Y. Q. Chen, G.-R. Li, Z. Chang, Y. K. Qu, Y. H. Zhang and X. H. Bu, Chem. Sci., 2013, 4, 3678–3682 RSC.
- X. L. Qi, R. B. Lin, Q. Chen, J. B. Lin, J. P. Zhang and X. M. Chen, Chem. Sci., 2011, 2, 2214–2218 RSC.
- T. H. Noh, H. Lee, J. Jang and O. S. Jung, Angew. Chem., Int. Ed., 2015, 54, 9284–9288 CrossRef CAS PubMed.
- L. E. Kreno, K. Leong, O. K. Farha, M. Allendorf, R. P. Van Duyne and J. T. Hupp, Chem. Rev., 2012, 112, 1105–1125 CrossRef CAS PubMed.
- R. B. Lin, F. Li, S. Y. Liu, X. L. Qi, J. P. Zhang and X. M. Chen, Angew. Chem., Int. Ed., 2013, 52, 13429–13433 CrossRef CAS PubMed.
- Z. C. Hu, B. J. Deibert and J. Li, Chem. Soc. Rev., 2014, 43, 5815–5840 RSC.
- D. M. Liu, K. D. Lu, C. Poon and W. B. Lin, Inorg. Chem., 2014, 53, 1916–1924 CrossRef CAS PubMed.
- S. Y. Liu, X. L. Qi, R. B. Lin, X. N. Cheng, P. Q. Liao, J. P. Zhang and X. M. Chen, Adv. Funct. Mater., 2014, 24, 5866–5872 CrossRef CAS PubMed.
- S. S. Nagarkar, A. V. Desai and S. K. Ghosh, Chem. – Asian J., 2014, 9, 2358–2376 CrossRef CAS PubMed.
- Z. G. Xie, L. Q. Ma, K. E. deKrafft, A. Jin and W. B. Lin, J. Am. Chem. Soc., 2009, 132, 922–923 CrossRef PubMed.
- J. An, C. M. Shade, D. A. Chengelis-Czegan, S. Petoud and N. L. Rosi, J. Am. Chem. Soc., 2011, 133, 1220–1223 CrossRef CAS PubMed.
- M. L. Ho, Y. A. Chen, T. C. Chen, P. J. Chang, Y. P. Yu, K. Y. Cheng, C. H. Shih, G. H. Lee and H. S. Sheu, Dalton Trans., 2012, 41, 2592–2600 RSC.
- S. M. Barrett, C. Wang and W. B. Lin, J. Mater. Chem., 2012, 22, 10329–10334 RSC.
- X. L. Qi, S. Y. Liu, R. B. Lin, P. Q. Liao, J. W. Ye, Z. H. Lai, Y. Y. Guan, X. N. Cheng, J. P. Zhang and X. M. Chen, Chem. Commun., 2013, 49, 6864–6866 RSC.
- Z. S. Dou, J. C. Yu, Y. J. Cui, Y. Yang, Z. Y. Wang, D. R. Yang and G. D. Qian, J. Am. Chem. Soc., 2014, 136, 5527–5530 CrossRef CAS PubMed.
- X. D. Wang and O. S. Wolfbeis, Chem. Soc. Rev., 2014, 43, 3666–3761 RSC.
- C. Ulbricht, B. Beyer, C. Friebe, A. Winter and U. S. Schubert, Adv. Mater., 2009, 21, 4418–4441 CrossRef CAS PubMed.
- Q. Zhao, F. Y. Li and C. H. Huang, Chem. Soc. Rev., 2010, 39, 3007–3030 RSC.
- Y. Feng, J. H. Cheng, L. Zhou, X. G. Zhou and H. F. Xiang, Analyst, 2012, 137, 4885–4901 RSC.
- M. D. Allendorf, C. A. Bauer, R. K. Bhakta and R. J. T. Houk, Chem. Soc. Rev., 2009, 38, 1330–1352 RSC.
- X. Jing, C. He, Y. Yang and C. Y. Duan, J. Am. Chem. Soc., 2015, 137, 3967–3974 CrossRef CAS PubMed.
- J. Zhang, Z. G. Yao, S. J. Liao, J. C. Dai and Z. Y. Fu, J. Mater. Chem. A, 2013, 1, 4945–4948 CAS.
- M. Fang, Y. Wang, P. Zhang, S. G. Li and R. R. Xu, J. Lumin., 2000, 91, 67–70 CrossRef CAS.
- H. D. Zhang, Y. H. Sun, K. Q. Ye, P. Zhang and Y. Wang, J. Mater. Chem., 2005, 15, 3181–3186 RSC.
- H. S. Wang, J. H. Huang, S. J. Wu, C. Xu, L. H. Xing, L. Xu and Q. B. Kan, Mater. Lett., 2006, 60, 2662–2665 CrossRef CAS PubMed.
- J. Liu, S. B. Hartono, Y. G. Jin, Z. Li, G. Q. Lu and S. Z. Qiao, J. Mater. Chem., 2010, 20, 4595–4601 RSC.
- Q. P. Lin, X. H. Bu, C. Y. Mao, X. Zhao, K. Sasan and P. Y. Feng, J. Am. Chem. Soc., 2015, 137, 6184–6187 CrossRef CAS PubMed.
- A. Meyers and M. Weck, Macromolecules, 2003, 36, 1766–1768 CrossRef CAS.
- B. H. Han, M. A. Winnik, A. B. Bourlinos and E. P. Giannelis, Chem. Mater., 2005, 17, 4001–4009 CrossRef CAS.
- A. Kimyonok and M. Weck, Macromol. Rapid Commun., 2007, 28, 152–157 CrossRef CAS PubMed.
- C. S. K. Mak, D. Pentlehner, M. Stich, O. S. Wolfbeis, W. K. Chan and H. Yersin, Chem. Mater., 2009, 21, 2173–2175 CrossRef CAS.
- Y. Q. Lan, H. L. Jiang, S. L. Li and Q. Xu, Inorg. Chem., 2012, 51, 7484–7491 CrossRef CAS PubMed.
- G. S. Yang, M. N. Li, S. L. Li, Y. Q. Lan, W. W. He, X. L. Wang, J. S. Qin and Z. M. Su, J. Mater. Chem., 2012, 22, 17947–17953 RSC.
- J. C. Yu, Y. J. Cui, C. D. Wu, Y. Yang, Z. Y. Wang, M. O'Keeffe, B. L. Chen and G. D. Qian, Angew. Chem., Int. Ed., 2012, 51, 10542–10545 CrossRef CAS PubMed.
- J. C. Yu, Y. J. Cui, H. Xu, Y. Yang, Z. Y. Wang, B. L. Chen and G. D. Qian, Nat. Commun., 2013, 4, 2719 Search PubMed.
- J. V. Morabito, L. Y. Chou, Z. H. Li, C. M. Manna, C. A. Petroff, R. J. Kyada, J. M. Palomba, J. A. Byers and C. K. Tsung, J. Am. Chem. Soc., 2014, 136, 12540–12543 CrossRef CAS PubMed.
- L. Wang, W. T. Yang, Y. X. Li, Z. G. Xie, W. Zhu and Z. M. Sun, Chem. Commun., 2014, 50, 11653–11656 RSC.
- Y. J. Cui, R. J. Song, J. C. Yu, M. Liu, Z. Q. Wang, C. D. Wu, Y. Yang, Z. Y. Wang, B. L. Chen and G. D. Qian, Adv. Mater., 2015, 27, 1420–1425 CrossRef CAS PubMed.
- D. H. Liu, T. F. Liu, Y. P. Chen, L. F. Zou, D. W. Feng, K. C. Wang, Q. Zhang, S. Yuan, C. L. Zhong and H. C. Zhou, J. Am. Chem. Soc., 2015, 137, 7740–7746 CrossRef CAS PubMed.
- H. Wang, J. Xu, D. S. Zhang, Q. Chen, R. M. Wen, Z. Chang and X. H. Bu, Angew. Chem., Int. Ed., 2015, 54, 5966–5970 CrossRef CAS PubMed.
- D. Luo, X.-P. Zhou and D. Li, Angew. Chem., Int. Ed., 2015, 54, 6190–6195 CrossRef CAS PubMed.
- W. Chen, Q. Peng and Y. D. Li, Adv. Mater., 2008, 20, 2747–2750 CrossRef CAS PubMed.
- L. Hou, Y. Y. Lin and X. M. Chen, Inorg. Chem., 2008, 47, 1346–1351 CrossRef CAS PubMed.
- R. B. Lin, T. Y. Li, H. L. Zhou, C. T. He, J. P. Zhang and X. M. Chen, Chem. Sci., 2015, 6, 2516–2521 RSC.
- M. Eddaoudi, J. Kim, N. Rosi, D. Vodak, J. Wachter, M. O'Keeffe and O. M. Yaghi, Science, 2002, 295, 469–472 CrossRef CAS PubMed.
- G. Baldacchini, P. Chiacchiaretta, R. Reisfeld and E. Zigansky, J. Lumin., 2009, 129, 1849–1852 CrossRef CAS PubMed.
-
B. Valeur, in Molecular Fluorescence: Principles and Applications, Wiley-VCH Verlag GmbH, Weinheim, FRG, 2001, pp. 1–272 Search PubMed.
- C. Montoro, F. Linares, E. Quartapelle Procopio, I. Senkovska, S. Kaskel, S. Galli, N. Masciocchi, E. Barea and J. A. R. Navarro, J. Am. Chem. Soc., 2011, 133, 11888–11891 CrossRef CAS PubMed.
- A. Bétard, S. Wannapaiboon and R. A. Fischer, Chem. Commun., 2012, 48, 10493–10495 RSC.
- X. Lu and M. A. Winnik, Chem. Mater., 2001, 13, 3449–3463 CrossRef CAS.
Footnote |
† Electronic supplementary information (ESI) available: Experimental section, PXRD patterns and characterization details. See DOI: 10.1039/c5qi00157a |
|
This journal is © the Partner Organisations 2015 |