Effects of stratospheric ozone depletion, solar UV radiation, and climate change on biogeochemical cycling: interactions and feedbacks
Received
20th October 2014
, Accepted 20th October 2014
First published on 7th November 2014
Abstract
Climate change modulates the effects of solar UV radiation on biogeochemical cycles in terrestrial and aquatic ecosystems, particularly for carbon cycling, resulting in UV-mediated positive or negative feedbacks on climate. Possible positive feedbacks discussed in this assessment include: (i) enhanced UV-induced mineralisation of above ground litter due to aridification; (ii) enhanced UV-induced mineralisation of photoreactive dissolved organic matter (DOM) in aquatic ecosystems due to changes in continental runoff and ice melting; (iii) reduced efficiency of the biological pump due to UV-induced bleaching of coloured dissolved organic matter (CDOM) in stratified aquatic ecosystems, where CDOM protects phytoplankton from the damaging solar UV-B radiation. Mineralisation of organic matter results in the production and release of CO2, whereas the biological pump is the main biological process for CO2 removal by aquatic ecosystems. This paper also assesses the interactive effects of solar UV radiation and climate change on the biogeochemical cycling of aerosols and trace gases other than CO2, as well as of chemical and biological contaminants. Interacting effects of solar UV radiation and climate change on biogeochemical cycles are particularly pronounced at terrestrial-aquatic interfaces.
Introduction
The Montreal protocol has been successful in phasing out ozone-depleting CFCs and, as a consequence, stratospheric ozone concentrations are recovering at low and mid-latitudes1,2 (see Bais et al.3). However, springtime ozone depletion is expected to continue at polar latitudes for many decades,4,5 largely due to climate change. A major consequence of stratospheric ozone change is altered intensity of solar UV-B radiation which in turn affects the biogeochemical cycling of carbon and other chemical elements. Terrestrial and aquatic biogeochemical cycles are discussed here in the context of their possible interactions with UV radiation and climate change (Fig. 1).
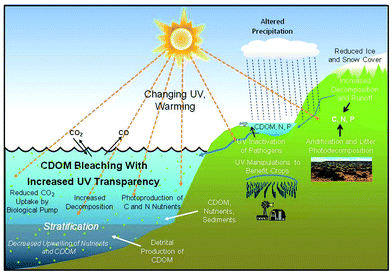 |
| Fig. 1 Conceptual model of aquatic and terrestrial processes that are possibly influenced by interactions between UV radiation and climate change. Recent findings on these interactions are discussed in more detail in this assessment. Reprinted from Williamson et al.,250 with permission from Macmillan Publishers Ltd: Nature Climate Change, copyright 2014. | |
The intensity of solar UV radiation reaching the Earth's surface is also controlled by climate-related variables such as cloud cover and aerosols. In addition, the penetration of UV-B radiation into water bodies largely depends on the concentration and the optical properties of chromophoric dissolved organic matter (referred to as coloured dissolved organic matter, CDOM, hereafter). Climate-change related effects on terrestrial and aquatic ecosystems, e.g. desertification, ocean acidification and stratification, as well as land use change interact in various ways with solar UV radiation, resulting in UV-mediated feedbacks on climate. Feedbacks, combined effects and interactions of solar UV radiation and climate change on biogeochemical cycles are discussed and assessed, and gaps in knowledge identified.
Interactive effects of stratospheric ozone depletion and climate change on terrestrial and aquatic ecosystems
We include a section that summarises some important issues of climate change, since climate change modulates the effects of solar UV radiation of biogeochemical cycles. Climate change also affects the stratospheric ozone concentration and thus the intensity of solar UV radiation reaching the Earth's surface.
Climate change and polar stratospheric ozone depletion
Some chemistry-climate models and observations predict that an ozone ‘hole’ may still be present in the Antarctic spring in 2100.4 A large loss of stratospheric ozone, comparable to that in the Antarctic ozone ‘hole’, was also observed over the Arctic in spring 20115 due, in part, to the extremely cold stratospheric Arctic winter 2010/2011.6 In spring 2011 increased levels of solar UV radiation were observed at Arctic and sub-Arctic ground stations.7 The Arctic stratosphere is particularly affected by radiative cooling due to Arctic amplification (enhanced warming in high northern latitudes relative to the northern hemisphere).8 There are several reasons for the fast response of the Arctic to global warming, including increased advection of water from the Atlantic to the Arctic,9,10 and reduced albedo due to sea ice melting and vegetation shifts.11 Hence, increasing attention is being paid to Arctic tipping points.12 The term “tipping point” is used for systems (e.g. Arctic ecosystems), where a small change in forcing could potentially cause a large change in future ecosystems.
Further detail on climate change and polar stratospheric ozone depletion is given in Bais et al.3
Ice melting: effects on local climates
Global warming has dramatically enhanced melting of glaciers, ice caps, and sea ice in both hemispheres, with the largest present rates of melting in the Northern Hemisphere.13–15 The Arctic sea ice, whose extent usually reaches a minimum each year in September, is particularly affected13,15 (Fig. 2) (also see Fig. 1 in Häder et al.16). Melting of Greenland and Antarctic sea ice is mainly due to warmer ocean temperatures.17 Increasing meltwater ponds on the surface of polar ice reduce albedo and allow more solar radiation, both UV and photosynthetically active radiation (PAR), to enter the water column through the ice18 (see Bais et al.3). Screen and Simmonds (2010)8 suggest that positive ice-temperature feedbacks occur in the Arctic due to increases in atmospheric water vapour content that enhances warming in the lower part of the atmosphere, especially in high northern latitudes. Sea ice melting at high latitudes could also be due to the transformation of solar UV-B radiation into heat by planktonic microorganisms in a cyclic reaction consisting of photooxidation of water at 300 nm that yields H2O2 with subsequent decomposition of H2O2 by catalase.19
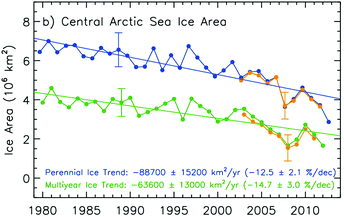 |
| Fig. 2 Arctic sea ice loss from 1979 to 2012 as derived from satellite passive microwave data. The yellow lines (after 2002) are from AMSR-E data. (Panel (b) of Fig. 4.4 in Chapter 4 “Observations: Cryosphere” of the IPCC Report 2013, Working Group I: Climate Change 2013: the Physical Science Basis).13 | |
The decline in Arctic sea ice affects local climates, particularly in North America, Europe, and East Asia with large impacts on biogeochemical cycles. For example, changes in rates of primary production and respiration in terrestrial and aquatic ecosystems may occur as a consequence of droughts and enhanced runoff of terrestrially derived organic carbon, respectively (see below). These effects on local climates can be summarised as follows: the melting of Arctic sea ice results in increased air temperatures due to evaporation and condensation of surface water, changes in the turbulent heat fluxes and, as a consequence, in increased geopotential height over the Arctic.20 As a result, the jet stream is transformed into Rossby waves that are characterised by cold air moving to the north and warm air moving to the south.21,22 The higher the amplitude of Rossby waves, the slower they move from west to east, resulting in more frequent episodes of blocking of weather patterns and therefore in longer lasting extreme weather events such as droughts, flooding, cold spells, and heat.21–25
Further impacts of land and sea ice melting are sea level rise,13 increases in freshwater input and hence changes in ocean circulation, reduced surface albedo26 (see Bais et al.3), and enhanced exposure of organisms in aquatic ecosystems and on land surfaces such as tundra to solar UV radiation (this assessment and Häder et al.16). Over the period 1901–2010, global mean sea level rose by 0.19 (0.17 to 0.21) m and the IPCC WGI Report 2013 (summary for policymakers)13 states with high confidence that the “rate of sea level rise since the mid-19th century has been larger than the mean rate during the previous two millennia”.
Interacting effects of stratospheric ozone depletion and climate change on ecosystems
The interplay between stratospheric ozone depletion and climate change affects atmospheric circulation, the speed and direction of winds, and, as a consequence, ocean mixing. There is a global trend of increasing values of surface and higher level wind speed, linked, in part, to stratospheric ozone depletion.27–29 Causes of this phenomenon have been reported more than ten years ago in a key paper by Gillet and Thompson (2003).30 Based on both observations and modeling results, these investigators found falling geopotential heights at constant pressure (500 hPa) at high southern latitudes from 1979–2000 due to Antarctic stratospheric ozone depletion and thus decreasing temperatures. Concomitantly, 500 hPa heights were rising in the middle southern latitudes, due to increasing greenhouse gas concentrations and thus increasing temperatures. This interplay between Antarctic stratospheric ozone depletion and climate change results in an upward trend of the Southern Annular Mode (SAM).28,31,32 Positive SAM indices result in mean high values of the westerly polar vortexes and a poleward shift of the westerly wind belts at the Earth's surface.27,33 As a consequence, net uptake of carbon dioxide in the Southern Ocean, believed to be a major sink for atmospheric CO2,34,35 may be reduced by enhanced wind-driven upwelling of carbon-rich deepwater.36,37 Paleo-oceanographic studies suggest that a Southern Ocean CO2 ventilation event might have caused glacial-interglacial changes in the atmospheric CO2 concentration.38–41 Changes in atmospheric and ocean circulation due to the combined effects of Antarctic stratospheric ozone depletion and climate change alter Southern Hemisphere weather. These alterations potentially include increased incidence of extreme events, floods, droughts, and wildfires.42
Ocean warming, stratification, acidification, and deoxygenation
Global warming results in increased sea-surface temperatures (SST) and, in turn, in thermal stratification. Another effect of increasing SST is ocean deoxygenation as a consequence of reduced oxygen solubility. Furthermore, increasing CO2 concentrations result in ocean acidification.34 Ocean acidification lowers the saturation state of calcite and aragonite (Fig. 3) and thus the ability of calcifiers such as coccolithophores and corals to produce and maintain their shells of calcite and aragonite.34,43
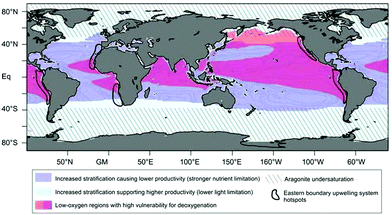 |
| Fig. 3 Global map showing oceanic regions of particular vulnerability to climate-change effects (see legend above). Reprinted from Gruber, 201134 with permission from The Royal Society: Philosophical Transactions A, copyright 2011. | |
Framework building corals are also sensitive to ocean warming because of the breakdown of the symbiosis with symbiotic algae (zooxanthellae), resulting in coral bleaching.34,44–47 Algae that no longer live in symbiosis with corals are less protected from solar UV-B radiation. Furthermore, iron stress of zooxanthellae has been shown to reduce their photosynthetic efficiency and to alter the pigment composition at elevated temperatures.48 The combined effects of increasing SST, ocean acidification and stratification, and solar UV radiation on marine ecosystems are likely to reduce the rate of primary production by phytoplankton (see below). Based on a Biogeochemical Elemental Cycling model, Laufkoetter et al. (2013)49 reported a decrease in global net primary production of phytoplankton with increasing SST from 1960–2006. An excellent review article on these topics has been published by Gruber (2011).34
Changes in hydroclimatic conditions and continental runoff
The interplay between global warming and stratospheric ozone depletion affects precipitation patterns in space and time and enhances the intensity of precipitation extremes.33,50–53 Cooling of the Antarctic stratosphere due to ozone depletion causes circulation cells to shift polewards, resulting in wetter conditions in the southern subtropics in the austral summer and drier conditions around 40° S.33,51 On the other hand, strong El Niño/Southern Oscillation (ENSO) events, potentially due to global warming, cause the South Pacific convergence zone to shift towards the Equator with concomitant changes in hydroclimatic conditions resulting in more frequent weather events such as droughts, floods, and tropical cyclones.54 In the Northern Hemisphere, the intensity of heavy precipitation events has increased over much of the land area due to warming.52,55,56 Changes in hydroclimatic conditions may result in an enhanced input of terrestrial organic matter into aquatic systems57–60 with consequences for the rate of UV-induced mineralisation of organic carbon (see below).
Changes in continental runoff of CDOM have consequences for the transmission of solar UV radiation into water bodies and thus for the protection of phytoplankton against the damaging UV-B radiation (see below). Remote sensing studies have provided new information on the extent of CDOM runoff and its impact on the penetration depth of UV radiation in the biologically-rich waters and coral reefs of South Florida (Fig. 4; Barnes et al. 201461).
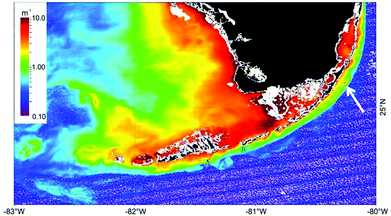 |
| Fig. 4 Image showing UV attenuation caused by runoff of colored dissolved organic matter in coastal regions of South Florida. Results were derived from satellite measurements (MODIS/A) on 9 January 2009 using a model described by Barnes et al.61 Land is masked in black. White indicates coastline, clouds or algorithm failure. Figure modified from Barnes et al.61. | |
Vegetation shifts, droughts, fires, and tipping points
Climate related changes in the spatial distribution and regional intensities of precipitation and temperatures extremes, among other variables, drive vegetation shifts, droughts and the occurrence and extent of fires. This results in changes in the biogeochemical cycles that are initiated by UV interactions with the terrestrial biosphere and fire-scarred continental surfaces. Solar UV radiation also impacts the oxidative state of the atmosphere, in both the gas and aerosol phases, further influencing greenhouse gases concentrations and budgets.62 This section seeks to provide heuristic linkages between climate related drivers such as wild fires, droughts and how UV fluxes, and changes and trends in UV fluxes, interact may push terrestrial ecosystems up to and over tipping points in ecosystem health.
Intensification of agriculture and the evolution of natural landscapes and ecosystems to managed ecosystems have increased rapidly over the past 50 years.11,63,64 Changes in plant species also occur in regions that are undergoing large climate-related ecosystem shifts.65 Climate-related changes in some of the Earth's largest terrestrial ecosystems are being detected.66,67 Droughts, for example, are reducing terrestrial primary production and are increasing in many regions of the Earth.68–71 UV radiation interacts extensively with the biogeochemical cycles that are shifting with changing land surface characteristics. The interactions of vegetation with the changing UV fluxes result in altered trace gas emission to the atmosphere including volatile organic carbon compounds (VOCs) (see below and Bornman et al.72). These UV-induced trace gas emissions include both direct emission of VOCs from plants, thought to be weakly sensitive to changes in UV radiation, and UV interactions with burned and/or modified litter. It is, however, difficult to separate changes in ecosystems due to ecosystem maturation and those due to climate change.73
As ozone, aerosols and trace gases change and evolve in the atmosphere, the UV radiation at the surface changes, as well as the characteristics of the surface itself.74 UV radiation-mediated release of trace gases and aerosols from the terrestrial biosphere then changes as a result of the altered make-up of the land surface. Fires have emerged as an important component of such land surface change.75,76 Not only do fires input massive amounts of chemicals and aerosols to the atmosphere, altering atmospheric UV radiation and chemistry, but they also leave charred substrate behind. Charcoal can persist for long periods in terrestrial systems. As a result, increasing amounts of black carbon, an important constituent of charred substrate, are entering rivers and flowing into lakes and the ocean where photochemical transformations are important sinks.77–80 The intent of this section is to highlight and draw attention to the often ignored interactions between UV radiation, atmospheric chemistry and surface land characteristics that are dependent on the extant ecosystems. The way in which these interacting systems are changing at the same time, often at different rates, is also addressed.
Terrestrial biosphere tipping points occur due to the complex interactions between UV radiation, drought-induced aerosol distributions, anthropogenic pollution and column ozone depletion over specific areas.7,81 UV radiation changes could represent the final source of variability that pushes/pulls the terrestrial biosphere over tipping points with large impacts on global biogeochemical cycles. Even with the general success of the Montreal protocol, there are still large areas with high UV radiation reaching the Earth's surface due to column ozone depletion.7 Tipping points in biogeochemical cycles may be traversed by strong gradients in surface UV radiation. Biogeochemical cycles are occurring over large regions of similar ecosystem make-up and experience large differences in UV flux due to atmospheric chemistry and physical climate variables such as clouds. As in the purely physical climate system, extreme events often have a greater impact on biogeochemical cycles than a change in the mean state.82,83 There are direct feedbacks where the processes of afforestation lead to changes in the general circulation of the atmosphere resulting in changes in precipitation.84 Extreme events, physical climate system shifts, fires and droughts all may contribute to UV initiation of tipping points in both terrestrial and aquatic biogeochemical cycles. For example, extensive increases in melting snow and ice cover lead to increases in the exposure of aquatic ecosystems to UV and PAR,18 which have the potential to create tipping points – shifts in photosynthetic vs. heterotrophic organisms where community as well as ecosystem structure and function are fundamentally altered.85
As ecosystems change due to climate change, fire and droughts, the chemicals released (i.e. hydrocarbon, CO2, CH4, aerosols) alter atmospheric chemistry. These biogeochemical changes have, in turn, implications for the residence times of greenhouse gases that are modulated by UV photochemistry. These interactions and biogeochemical cycling aspects of the Earth system often fall outside of traditional boundaries of scientific specialization and are only now becoming more fully appreciated.
Interactive effects of solar UV radiation and climate change on the carbon cycle
Carbon exchange between ocean and atmosphere
Air-sea CO2 fluxes vary locally and seasonally and depend on the partial pressure of CO2 and, in turn, on ocean temperature, mixing, and biology.86,87 The main biological process that controls the uptake of CO2 by the ocean is the so-called biological pump (CO2 binding in photosynthesis by phytoplankton and export of dead particulate organic matter to the ocean sediment) (Fig. 5).
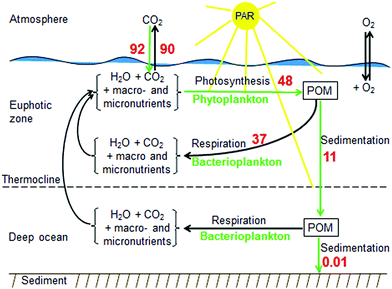 |
| Fig. 5 Schematic representation of the biological pump, indicated by the green arrows. The efficiency of the biological pump depends on the rates of both photosynthesis (primary production) with photosynthetic active radiation (PAR) and the formation and sedimentation of particulate organic matter (POM). Numbers in red are from Houghton251 and correspond to approximate global mean fluxes in Pg C year−1. | |
Rates of primary production depend on various factors including light and nutrient availability, temperature, and the species composition of phytoplankton. Also phytoplankton performance is important for efficient CO2 uptake and may be negatively affected by solar UV-B radiation.88,89 Evidence has continued to mount that the concentration of CDOM controls the penetration of UV and short-wavelength visible radiation into lakes and the coastal ocean.61,88–91 CDOM effectively protects aquatic ecosystems from harmful UV-B radiation while permitting PAR to be transmitted into the water column. The degradation of CDOM by photochemical and biological processes leads to a loss of colour and reduced UV absorbance, both in the UV-B and UV-A regions.92–98 As a consequence of CDOM photobleaching, the penetration depths of solar UV radiation into water bodies increase, thereby increasing the exposure of phytoplankton to damaging solar UV-B radiation (see also Häder et al.16). CDOM photobleaching is particularly evident in thermally stratified waters bodies.95,98,99 Stratification results in a shallower mixing depth, which in addition leads to a greater exposure of phytoplankton to UV-B radiation.100 Enhanced stratification, due to increasing sea surface temperatures, occurs mainly in low latitude marine environments where it also hinders the transport of nutrients to the euphotic zone from deeper water layers and hence negatively affects primary production.49,101 Stratification has also been observed in ice-free Arctic marine regions due to increased meltwater input.102 Cai et al.102 predict that an ice-free Arctic Ocean will not become a larger CO2 sink due, in part, to an inefficient biological pump as a consequence of negative effects of solar UV radiation on phytoplankton.
CDOM in aquatic systems originates from several sources. In the coastal ocean, continental runoff of CDOM plays an important role (see also above).90,91,96,103–105 In the open ocean and large lakes, CDOM is a by-product of biological degradation of dead phytoplankton.90,97,106 Thus, a reduced concentration of phytoplankton will drive decreased CDOM production, further increasing transmission of UV radiation into the ocean. The observed reductions in chlorophyll concentration that have been attributed to increasing sea surface temperatures101 are probably caused by a combination of increased exposure to UV radiation and reduced nutrient upwelling.107
An important part of the biological pump is the export production, i.e. the formation and sedimentation of particulate organic matter (POM) stemming from dead phytoplankton and zooplankton material. The export production has decreased by 8% from 1960–2006 globally with strong spatial variability, based on model calculations.49 One reason for this decline may be a global decrease in the biomass of small phytoplankton and diatoms in the period 1960–2006 (8.5% and 3%, respectively49). Ocean acidification may further reduce the biomass of coccolithophores, small calcifying phytoplankton, and hence the production of CaCO3 “ballast” that enhances sinking rates of carbonate-rich POM.34,49,108,109 Overall, the interaction of solar UV radiation with climate-change effects such as ocean stratification is likely to decrease the efficiency of the biological pump and to cause UV-mediated, positive feedbacks on atmospheric CO2 (see also below).
Carbon exchange between terrestrial ecosystems and atmosphere
The uptake of CO2 by terrestrial ecosystems via photosynthesis of plants and the release of CO2 to the atmosphere via decomposition of senescent plant material (litter) are affected both positively and negatively by solar UV radiation and climate change.89,110 Climate change, and in particular the intensity and frequency of droughts in terrestrial ecosystems could negatively impact primary production by plants and crops and thus CO2 uptake by terrestrial ecosystems.13,68,71 At the same time, changes in climate that decrease cloudiness and increase UV radiation at the terrestrial surface may increase losses of carbon from terrestrial ecosystems due to increased photochemical degradation of plant material.111
A part of the CO2 fixed by plants may be sequestered in forests or long-lived plant soil components such as woody tissue or peat in soil organic matter, thus increasing its residence time in organic pools. Plant litter on the soil surface is, however, subject to rapid degradation, which results in emissions of CO2 and other greenhouse gases to the atmosphere.89,112–114 The release of CO2 from terrestrial ecosystems by microbial degradation of soil organic matter is strongly affected by temperature and moisture.115–117 In addition, there is increasing evidence of abiotic decomposition of above ground litter due to UV radiation, particularly in arid and semiarid regions with low soil biotic activity.111,112,114,116–124 Rates of plant litter photodegradation due to exposure to UV radiation depend on various factors, including: (i) plant cover,111 (Fig. 6), (ii) the intensity of solar UV radiation (altitude, latitude), (iii) exposure of litter mass to solar radiation due to differences in litter area to mass ratio, and (iv) chemical composition of plant litter118,122 (Fig. 7) (see also Bornman et al.72).
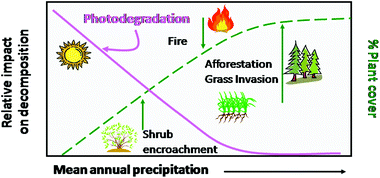 |
| Fig. 6 Hypothesized effect of plant cover on photodegradation in terrestrial ecosystems. Plant cover is a key factor which will affect the degree of exposure to UV radiation and impacts of photodegradation as a vector of carbon loss in arid and semiarid ecosystems. As precipitation increases, primary production and resulting plant cover increase, thereby reducing the relative importance of photodegradation in mesic and humid ecosystems. Global changes such as shrub encroachment, increased fire frequency, invasion of non-native annual species and afforestation will all affect plant cover and consequently the relative impact of photodegradation on decomposition in these ecosystems. Red line shows the importance of photodegradation as rainfall increases, the dashed line is changes in plant cover. Green arrows indicate the direction of change in plant cover with global change. Figure modified from Austin (2011).111 | |
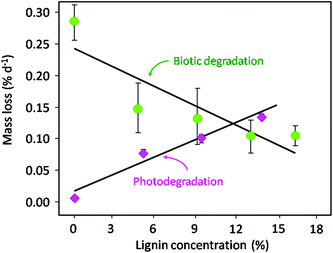 |
| Fig. 7 Effects of lignin concentration on biotic decomposition and photodegradation. While biotic degradation of plant litter is inhibited by high lignin concentrations, lignin serves as a light absorbing compound facilitating photodegradation and carbon release from decomposing plant litter. Green circles indicate material of varying lignin concentrations which was decomposed in shaded conditions in contact with soil (biotic decomposition) while red diamonds indicate identical material of varying lignin concentrations that was isolated from the soil surface and exposed to full solar radiation. Figure modified from Austin and Ballaré (2010).118 | |
Recent information regarding the role of litter chemistry in determining the rate of UV-induced litter degradation has identified the importance of lignin in the process of photodegradation. Several studies have shown a decrease in litter lignin content with exposure to solar or UV-B radiation125,126 and that lignin content of litter is positively correlated with higher rates of mass loss (Fig. 7, Austin and Ballaré118). The latter can be explained in terms of a higher percentage of aromatic components in lignin and thus a higher rate of light absorption in the UV and visible range.118,120,121,127 Although abiotic photodegradation increases with the lignin content, the rate of microbial degradation tends to decrease with increasing lignin content, due to the bio-recalcitrant nature of lignin118 (Fig. 7). Finally, there is some evidence that exposure of plant litter to solar UV-radiation can increase the bioavailability of some compounds to soil microorganisms,112,119–121 although the mechanistic basis of this stimulation has not been established.
The direct and indirect effects of UV radiation on decomposition of plant litter is likely to become a much more significant global pathway for terrestrial organic matter decomposition in the future. Aridification due to land use and/or climate change could amplify photodegradative losses from senescent plant litter, with large potential impacts on the carbon balance of terrestrial ecosystems. Particular attention needs to be paid to Arctic terrestrial ecosystems. The Arctic region is experiencing rapid warming and its land area, including the tundra, has large stores of carbon that are likely to be rapidly converted to GHGs with warming. For example, losses from plant litter may be enhanced due to the thawing of permafrost119 and Arctic tundra wildfires,128 (see also above). All of these global changes have potential to affect plant cover (Fig. 6, Austin111), and hence increase the importance of photodegradation in these modified ecosystems.
The terrestrial-aquatic interface
Terrestrial and aquatic ecosystems are largely linked via the biogeochemical cycling of carbon. Runoff and leaching of plant litter is a major source of CDOM in aquatic environments, especially in freshwaters. In coastal marine environments, a large fraction of organic carbon originates from terrestrial ecosystems that is transported to coastal oceans (i) via rivers,59,129–133 and (ii) fluxes from wetlands, particularly from mangrove swamps134 (Fig. 4). Terrestrial organic matter (tDOM), in particular its CDOM fraction, more strongly attenuates solar UV radiation than organic matter produced within lakes and the sea due to its relatively high aromatic content,89,94,96,103,105,135,136 where the optical properties of terrigenous CDOM arise primarily from partially oxidised lignins originating from vascular plant sources137 (see also above). UV-induced photodegradation occurs more readily with terrestrially derived CDOM than with microbial CDOM89,94,136,138,139 where microbial CDOM is derived from autochthonous organic sources in the ocean and lakes such as dead phytoplankton cells. In the Arctic Ocean, continental runoff is a major source of terrigenous organic material and nutrients, thereby influencing water column stratification, gas exchange, light attenuation, surface heating, biological productivity, and carbon sequestration. A remote sensing study of the pan-Arctic distribution of tDOM and continental runoff in the surface Arctic Ocean indicated a correspondence between climate-driven changes in river discharge and tDOM inventories in the Kara Sea.103
The major carbon substances resulting from UV-induced photodegradation of CDOM are inorganic species: dissolved inorganic carbon (DIC) and to a lesser extent carbon monoxide (CO)96,105,140 (see Fig. 8). Photochemical DIC formation may strongly impact carbon cycling in seawater and other natural waters. For example, the annual photodegradation of CDOM exceeded the annual terrestrial input of photoreactive CDOM to the Baltic Sea, indicating that photochemical transformation is a major sink for terrestrial CDOM in such coastal systems.141 Other mechanistic studies of the efficiencies of CDOM photodegradation have shown that DIC photoproduction rates are up to 30 times greater than CO photoproduction96,105,140 (Fig. 8). Although not precise, these estimates suggest that CO2 photoproduction rates are comparable to other oceanic CO2 production terms, e.g. microbial respiration.
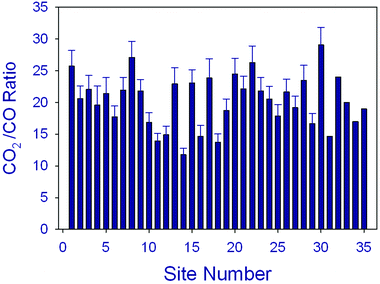 |
| Fig. 8 Measured ratios of sunlight-induced CO2/CO photoproduction in oceanic water samples collected from a variety of locations and times. The average ratio is close to 20 in marine systems but somewhat lower in freshwaters.96,105,140 | |
Not only the UV-induced but also the microbial degradation of DOM (respiration, see Fig. 5) results in production of CO2. The rate of this process is largely controlled by the availability of DOM to heterotrophic bacteria, which in turn depends on the chemical composition of DOM.59,127,142,143 UV-induced transformations generally decrease the bioavailability of microbial CDOM and enhance the bioavailability of terrigenous CDOM.89,127,142 Such a UV-induced enhancement also has been observed in the case of terrestrial plant litter where the term “photochemical priming” has been used to describe this enhancement (see Bornman et al.72).
Climate change can influence the UV-induced degradation of CDOM through effects on its sources. For examples, melting of glaciers, ice sheets, and thawing permafrost heightens the input of microbial DOM into aquatic systems144–146 and this source of CDOM generally becomes less biodegradable when transformed by solar UV radiation. Furthermore, both changes in continental hydrology and land use change may increase fluxes of tDOM from land to ocean59,60,147–149 thereby enhancing UV-induced CO2 production via mineralisation of tDOM.132 According to Regnier et al. (2013),148 the carbon flux to inland waters from soils has risen by 1.0 Pg C per year since preindustrial times. Also land use changes can affect sources of CDOM. For example, disturbance of peatlands has resulted in a 32% increase in fluvial organic flux from southeast Asia147 and this source of tDOM likely is photodegradable. Arctic aquatic ecosystems are particularly affected by increased input of DOM due to changes in rainfall, melting of ice, snow, and the permafrost. Depending on its source, DOM in Arctic aquatic ecosystems is subject to UV-induced or microbial mineralisation, where photochemical priming plays an important role.150
Links between carbon and nutrient cycles
In aquatic ecosystems, CO2 fixation in photosynthesis by phytoplankton (primary production) plays a key role in linking the carbon and nutrient cycles. The rate of primary production depends, in part, on the concentration and bioavailability of macro- and micronutrients present in the euphotic zone. Many nutrients are transported into the euphotic zone from deeper water layers.151 However, ocean stratification due to increasing sea-surface temperatures (SST) hinders the upwelling of nutrients and, as a consequence, negatively affects primary production and hence the biological pump49,101,107(see above). Micronutrients (essential trace metals including Fe, Cu, Zn, Mn, Co, and Cd) may co-limit phytoplankton growth and primary production in marine environments.48,152–154 The biological availability of essential trace metals depends on their chemical speciation. In general, only dissolved and weakly-bound or unchelated metals are available to phytoplankton.155–157 For this reason, oxidation and mainly reduction processes (redox reactions) induced by solar UV radiation play an important role in the formation of bioavailable metal species.158 Increased vertical mixing of water bodies reduces rates of UV-induced redox cycling of essential metals. For example, sea-ice loss in the western Antarctic Peninsula is hypothesised to reduce iron bioavailability because of greater vertical mixing in winters with little sea ice.159
Iron plays a key role as a micronutrient and co-limits phytoplankton growth particularly in the so-called high-nutrient-low chlorophyll (HNLC) oceanic regions.152,153 In oxygenated marine environments, Fe(III) is largely present in the form of solid Fe(III) phases, with low solubility. In the Southern Ocean, for example, dissolved iron concentrations range between 0.4 and 1.5 nmol L−1.160 However, these solid iron phases can undergo UV-induced dissolution yielding dissolved iron species161,162 and this photochemical process has been shown to occur at pH-values of marine waters.161 Ocean acidification (see above) can result in reduced availability of essential trace metals such as Fe, Zn, and Cd to phytoplankton, either via changes in the chemical speciation of metals or by decreasing the effectiveness of the enzymatic reduction of metals.157,163 For example, the uptake rate of iron bound to the siderophore desferrioxamine B by a model diatom was by a factor of ∼2 lower in seawater samples with pH 7.8 as compared to samples with pH 8.7.163 Ocean acidification also slows down nitrogen uptake by phytoplankton under low iron conditions by lowering the efficiency of N2 fixation.164
Combined effects of solar UV radiation and climate change on the biogeochemistry of trace gases and aerosols
Terrestrial and aquatic environments are important atmospheric sources and sinks of trace gases and aerosols. Recent research on the air–sea exchange of trace gases has provided further evidence that these sources are strongly influenced by interactions between solar UV radiation and climate change.
Methane
Methane is an important greenhouse gas that is stored in various reservoirs in the terrestrial, aquatic and atmospheric systems. UV radiation is involved in the release and exchange of CH4 between these reservoirs as well as atmospheric chemical processes in the troposphere and stratosphere.165–167 Temperature, water vapour, stratospheric ozone, biomass burning, and lightning NOx are the dominant sources of inter-annual changes in methane lifetime.166 UV radiation plays a critical role in determining the effectiveness of each of these sources in inter-annual changes in methane lifetime which is ∼9 year.168 The linkages and inferences of this section revolve around the UV-mediated atmospheric chemistry, ozone, OH etc. that drive atmospheric methane concentrations, and how CH4 release and uptake from land, aquatic and atmospheric reservoirs is influenced by UV radiation and biogeochemical cycles in general.
New satellite and airborne data assimilation techniques allow high-resolution, global estimates of the sources and sinks of atmospheric methane.169 These emerging technologies allow quantification and evaluation of the wild-fire production of atmospheric methane, an increasing source due to the climate related increased occurrence of wildfires.170,171 UV radiation plays a critical role in the atmospheric chemistry of methane as well as modulating its production in soils and other components of the terrestrial biosphere,172,173 although Morsky et al.174 found its role in methane production in a sub-arctic fen was more modest. The release of methane to the atmosphere from land surfaces is dependent upon climate change in the terrestrial biosphere as well as heat and UV supply to specific methane reservoirs such as permafrost regions.
Methane fluxes from the Arctic Ocean are strong and occur mainly in areas of open water between sea-ice (leads) and fractional sea-ice up to 82° N.175 Additional sources from submarine permafrost regions have also been reported.176 Such oceanic regions are likely to become increasing sources of atmospheric methane in the future due to climate-related changes in sea-ice cover. Warming has also been shown to affect the emission of methane in Western Siberian lakes with implications for atmospheric chemistry and UV interactions.177 We posit that as climate changes, ocean sea-ice coverage changes and often decreases, resulting in new and increased distributions of air-sea methane flux. The resultant methane releases will change and will be occurring simultaneously with the evolving atmospheric UV radiation budget.
Carbon monoxide
Carbon monoxide (CO) is widely produced through interactions of solar UV radiation with organic substances in aquatic and terrestrial systems. The average ratio of measured CO2/CO fluxes from various marine environments is close to 20 (Fig. 8). CO participates in chemical reactions that change air quality and it is one of the most important sinks for atmospheric hydroxyl radicals (˙OH). Due to its significant effects on ˙OH, CO also indirectly influences the concentrations of methane, halocarbons and other gases in the troposphere (see above). Recent research has provided further evidence that UV-induced processes as well as abiotic thermal processes involving tDOM in aquatic systems and plant litter on land are significant sources of CO to the atmosphere.96,105,122,140,178–182 tDOM generally is a more efficient source of CO (higher apparent quantum yields) than microbial CDOM derived from marine sources such as algal detritus. For example, CO photoproduction efficiencies in the Tyne estuary were highest for high CDOM riverine samples and almost an order of magnitude lower for low CDOM coastal seawater samples.180 One exception to this general observation is the unusually high efficiency of CO photoproduction from CDOM within floating Sargassum colonies.96 There are also significant CO microbial sinks in aquatic and terrestrial systems and the competition between these sources and sinks often results in diurnal fluctuations in the net exchange of CO between biosphere and atmosphere.181–183
An extensive study of the sea to air exchange of CO2 in the sub-arctic estuarine water body, the Canadian St. Lawrence estuary system, showed that the rates of photoproduction and microbial consumption of CO are approximately balanced.182 Other findings are that the photochemical efficiency for CO production from DOM in aquatic systems decreases with increasing salinity across the freshwater-marine mixing zone.140,173,179,184
Studies of terrestrial plants have provided additional evidence that exposure to UV radiation leads to production of CO from dead plant matter (litter) and that production rates depend on the litter traits.123 Studies of the effects of changing wavelength on CO photoproduction from litter are uncommon but one study showed that UV radiation induces CO production from Sequoia dendrongiganteum litter. CO emissions were strongly reduced in the absence of oxygen as compared to air.185 Other studies have shown that living plant leaves also photoproduce CO,186 although the production efficiency generally is much lower than for senescent or dead vegetation.
Nitrogen compounds
Among nitrogen-containing gases, nitrogen oxides (NOx = NO + NO2) play an important role in atmospheric chemistry, and many nitrogen gases have important interactions with UV radiation. In the troposphere, NOx enhances the formation of O3via UV-induced reactions (see Madronich et al.187). In the stratosphere, the opposite is the case, where NOx destroys O3 by the following reaction: NO + O3 → NO2 + O2, where NO2 acts as a catalyst in stratospheric O3 destruction.188 Furthermore, the reaction of NO2 with ClO yields ClONO2, a reservoir of reactive chlorine that continues to play an important role in polar stratospheric ozone depletion in the Arctic and Antarctic spring. Given these important interactions, changes in the atmospheric concentrations of nitrogen oxides due to climate change or other human activity may indirectly affect ozone recovery and the amount of UV radiation reaching the Earth's surface.
Anthropogenic NOx emission from combustion of fossil fuel, is an important source of NOx to the atmosphere. Additionally, nitric oxide (NO) from natural sources is produced mainly by anaerobic bacteria that reduce nitrate (NO−3) to nitrogen gas N2;189 NO is also a by-product of the oxidation of ammonium (NH4+) to nitrate by ammonia-oxidising soil bacteria.189,190 Nitrous acid (HONO) emissions from ammonia-oxidising bacteria are comparable with emissions of NO.190,191 Since soil emissions of both NO and HONO depend on the soil water content,190 increasing soil dryness, due to climate change, may increase the emission of NO and HONO from soils. UV-induced photolysis of HONO is a major source of the hydroxyl radical (HO˙) (up to ∼30% of production in the lower atmosphere191). In addition to soil emissions of NOx from biotic processes, UV-induced photolysis of NO3− (photodenitrification) is an important source of NOx to the atmosphere.192,193 This abiotic process has been shown recently to occur on the surface of nitrate-containing snow in the Arctic,192 and in sea salt particles containing Cl− and Br−.193
Another key nitrogen-containing gas is nitrous oxide (N2O), an important greenhouse and stratospheric ozone depleting gas. The surface emission of N2O approximately balances the stratospheric N2O loss188via formation of the ozone destroying NO [N2O + O* → 2NO, where O* is produced via photolysis of ozone: O3 + hν → O* + O2]. In terrestrial ecosystems, N2O is formed by denitrifying soil bacteria that reduce NO3− to N2.189 N2O formation in soils depends on soil water content (SWC) and temperature and occurs at higher SWC than soil emission of HONO and NO.190,194 Van Groenigen et al.195 predict that rising levels of CO2 will result in increased emissions of N2O from upland soil due to reduced plant respiration and thus higher SWC. In addition, more frequent precipitation events, due to climate change, that increase SWC could thus enhance the N2O flux from soils.194
In aquatic ecosystems, ammonia is produced by the UV-induced degradation of DOM184 and N2O by both denitrifying bacteria and ammonia oxidising archaea.196 N2O formation is particularly efficient in suboxic and hypoxic oceanic regions (∼10% of the ocean volume).197,198 The area of such regions is likely to expand (Fig. 3) due to the following factors: (i) eutrophication of coastal waters, (ii) decreasing O2 solubility with warmer water temperatures, and (iii) shifts in respiration towards the upper oceanic water column because of reduced sinking velocity of particles in the ocean108,197,199 (see also above). Furthermore, it has been shown that during storms, N2O rich subsurface water is mixed upwards198 suggesting that more frequent storm events, due to climate change, may result in increased emission of N2O from marine environments. In addition, sea-ice retreat has been suggested to decrease the residence time of N2O in the surface water of the Arctic Ocean and thus could enhance the sea-air flux of N2O.200,201 Geoengineering via fertilisation of marine regions with iron could enhance marine N2O production,202 (see also Bais et al.3).
Halogen compounds
Biogenic processes in terrestrial and aquatic ecosystems are important sources of halocarbons other than CFC (e.g. the methyl halides CH3Br, CH3Cl, and CH3I) to the atmosphere.203–207 In aquatic ecosystems, phytoplankton is involved in the formation of these halocarbons, particularly pico-sized phytoplankton,206 and to a lesser extent bacteria belonging to Erythrobacter or Pseudomonas.204 The rate of halocarbon production in aquatic ecosystems also depends on the chemical composition of dissolved organic matter (DOM), with tDOM playing an important role in the formation of halocarbons such as bromoform (CHBr3) and dibromomethane (CH2Br2).207 Hence increased fluxes of tDOM from land to ocean, due to climate change, may enhance the formation of halocarbons, particularly in eutrophic coastal waters. In addition to the biotic production of halocarbons, abiotic UV-induced processes could also be involved in the formation of CH2ClI, and CH2I2 in seawater.206
In the atmosphere, halocarbons are transformed into reactive halogen species (RHS, e.g. the radicals Br˙ and BrO˙), where the hydroxyl radical (HO˙, formed in reactions induced by solar UV radiation) acts as the oxidant.191,208 In the troposphere, RHS react with ozone209,210 and other gases, e.g. gaseous elemental mercury (Hg0)211 (see also below). Halocarbons can reach the lowermost stratosphere and undergo UV radiation-induced transformations yielding RHS, particularly BrO˙, that participate in stratospheric ozone depletion.208,212 Models suggest that the loading of the stratosphere with reactive bromine species will increase in the future due to climate change213 and thus the destruction of ozone in the stratosphere by RHS represents a positive feedback on solar UV radiation. This feedback could be enhanced by climate-change related processes, particularly by increased input of tDOM into coastal zones207 and by increases in ocean mixing and thus transport of CH3Br to the lower stratosphere.208
Aerosols
Atmospheric aerosols play an important role in the biogeochemistry of greenhouse gases, atmospheric circulation trends due to changes in climate and atmospheric chemistry.214 The surfaces of atmospheric aerosol particles provide reactive multi-phase regions for chemical transformations in the atmosphere. Surfaces, as well as the liquid phase portions inside the aerosols, interact with UV radiation to process chemicals critical to climate and atmospheric chemistry in general. Aerosol distributions and trends in distributions due to climate change alter UV fluxes to Earth's surface. This is due to direct blocking of UV radiation by aerosols in the atmosphere and the fact that the distributions and the radiative and chemical make–up of the aerosols are evolving as climate changes.
Aerosols affect circulation directly via interactions with radiation with attendant thermal gradient impacts.215,216 This means that strong feedbacks exist between aerosol distributions, UV radiation, and the circulation that influence precipitation and atmospheric transport.217 Changes in the distribution of atmospheric aerosols are occurring at the same time as shifts in terrestrial and aquatic and marine ecosystems.218 UV radiation is involved in and affected by biogeochemical feedbacks that result from chemical exchanges at the Earth surface, physical circulation changes and human-induced modification of the environment. UV interactions with the liquid phase of marine aerosol particles result in trace gas production, which alter the oxidation state of the atmosphere. Fe compounds have recently been implicated in these aerosol multiphase interactions.219 Aerosols not only scatter and absorb atmospheric UV radiation they influence and change atmospheric gas and liquid phase chemistry.
Urbanisation and managed agriculture have resulted in changing patterns of atmospheric dust distributions and deposition.220 These dust aerosols, which affect the transparency of the troposphere, have a concurrent impact on UV flux to aquatic and terrestrial ecosystems. Non-Asian sources are a significant source of dust to the North Pacific221 and will likely result in significant changes in the supply of nutrients, as organically bound Fe and P is transported atmospherically from continental regions to ocean ecosystems.147,218,222 Information from studies of the impacts of volcanic supplies of aerosols are being used to predict the impact of future aerosol distributions on the carbon cycle.223
There is an emerging realisation that liquid phase UV-driven aerosol chemistry can influence biogeochemical cycling of atmospheric greenhouse gases.224 This means that not only do atmospheric aerosols modulate surface UV fluxes via their distribution in the atmospheric column, but also the multi-phase chemical processes that occur on/in aerosols affect the oxidative state of the atmosphere. An example of this multi-phase chemistry is the Fe chemistry in aerosols that influences atmospheric radicals, oxidative state and lifetimes of greenhouse gases.219 These newly identified biogeochemical cycles assist in understanding some of the interactions between UV radiation, aerosol chemistry and atmospheric greenhouse gas distributions.
Combined effects of solar UV radiation and climate change on chemical and biological contaminants
Organics
Global climate change and interactions with solar ultraviolet radiation will potentially influence chemical fate and bioaccumulation in terrestrial and aquatic environments.225,226 Reviews and modeling studies have particularly focused on the Arctic and persistent organic pollutants (POPs)227–229 and predict the following effects: (1) Increased ambient temperatures could lead to increased emissions of chemicals through passive volatilisation from materials and stockpiles; (2) Increased emissions of POPs are predicted as a result of changing land-use patterns and changes in global agricultural practices, such as pesticide formulations and application rates; (3) Changes in energy use will affect use and release of chemicals; (4) Increases in the frequency of forest fires will increase the emissions of polycyclic aromatic hydrocarbons and other combustion by-products such as charcoal and black carbon.
Although POPs are chemicals such as chlorinated compounds that are resistant to biodegradation and/or to photodegradation through direct absorption of sunlight, these chemicals can be transformed by indirect photoreactions that involve natural substances such as tDOM (or related natural organic substances that were isolated from soils or natural waters), peroxides, nitrate or certain trace metals. Most of the recent examples of indirect photoreactions involve participation of tDOM.228,230–233 Chemicals having a wide variety of structural features participate in these indirect photoreactions including chlorinated contaminants,228,233 antibiotics,232 beta-blockers,231 and other drugs.230
One manner through which environmental variables influenced by global climate change could affect contaminant toxicity involves direct effects of the variable on chemical characteristics. The toxicity of polycyclic aromatic hydrocarbons (PAHs), for example, can be photoactivated by solar UV radiation.226 The intensity and wavelength distribution of UV radiation, key factors in determining PAH phototoxicity, are likely to be affected by variables altered by climate change. For example, these variables could include decreases in pH that can increase water clarity, thereby increasing exposure of aquatic animals to UV radiation, and increased inputs of CDOM or particulate carbon to aquatic systems, which would effectively reduce UV penetration. Hence, specific influences of climate change on UV intensity in aquatic systems are likely to be site- and situation-specific.
Although increased UV-B radiation can negatively affect the growth and viability of many organisms in aquatic food webs, sensitivity to UV radiation has the beneficial effect of disinfecting pathogens.141,234–236 This process is facilitated by climate and UV-induced changes that alter exposure of surface-dwelling organisms through increased water transparency and stratification, and reduced ice and snow cover. For example, human pathogenic viruses, which are frequently found in rivers, lakes and drinking water, are sensitive to solar UV radiation236 and disinfection by solar UV irradiation (SODIS) is becoming a widely-used tool for purification of drinking water in developing countries.235 The observed increases in CDOM in aquatic environments can have opposing effects on pathogen levels. On the one hand, the increases in CDOM reduce UV exposure and thus disinfection by direct photoinactivation of parasites and pathogens. On the other hand, photoinactivation of pathogens can be enhanced through photosensitisation by CDOM.236
Inorganics: mercury
Mercury (Hg) is one of the inorganic priority pollutants and its biological availability and thus toxicity is strongly affected by solar UV radiation and climate change. Mercury is not an essential metal and is toxic at very low concentrations. Most mercury released to the atmosphere is in the form of gaseous elemental mercury (Hg0), which can be transported over long distances and is deposited mainly in the oxidised form as divalent mercury (HgII).237 In the oxidation process, the radicals BrO˙ and Br˙, that are formed in UV-induced reactions, play a key role.211 In addition, UV-induced re-reduction of HgII occurs in the troposphere, however, at a lower rate than Hg0 oxidation.237 Following deposition to terrestrial and aquatic ecosystems, HgII undergoes methylation yielding methylmercury (MeHg), the toxic form of mercury. In sunlit surface waters MeHg may undergo UV-induced decomposition, a process that is enhanced by DOM.60
Climate change may amplify effects of solar UV radiation on the biogeochemical cycling of mercury. For example, ocean warming increases the sea-air flux of Hg0.238 As a consequence, the rate of oxidation of Hg0 to HgII (via UV-induced formation of reactive radicals, see above) can be expected to increase since the oxidation rate depends on the concentration of both reactive radicals and Hg0. Hence it is likely that the combined effects of ocean warming and solar UV radiation on the biogeochemical cycling of mercury result in increased formation of the toxic form of mercury.
Nanomaterials
In addition to organic contaminants and mercury, new chemicals are coming into global markets that require initial assessment. Inorganic and organic engineered nanomaterials (ENMs) and their composites with polymers and coatings (see also Andrady et al.239) are being introduced into the environment through widespread use in consumer products. Concerns about their health and safety have stimulated research on their persistence and toxicity. UV-induced photoreactions are important environmental processes for ENMs such as nanosilver, nano-titanium dioxide, fullerenes, carbon nanotubes and graphene oxide. These photoreactions are sensitised by tDOM in some cases. For example, nanosilver can be readily formed by the tDOM-induced photoreduction of ionic silver,240,241 a sunlight-induced process that helps reverse toxicity associated with oxidation of nanosilver. UV-induced processes initiate the environmental release of ENMs from polymer nanocomposites by photodegradation of the polymer matrix.242
Gaps in knowledge
Our understanding of the interacting effects of solar UV radiation and climate change on the biogeochemical cycling of carbon, nitrogen and halogen compounds, essential and toxic metals, and on the fate of organic pollutants has increased in the four years since our last assessment. However, there remain important gaps in knowledge. For example, climate models generally do not include effects of solar UV radiation on biogeochemical cycles. To do this would be important for predicting trends in the net CO2 sink strength of terrestrial and aquatic ecosystems.
Terrestrial and aquatic ecosystems are currently net CO2 sinks on a global average, where the CO2 uptake corresponds to ca. 30% and 25%, respectively, of anthropogenic CO2 emission.243,244 However, the CO2 sink strength of terrestrial and aquatic ecosystems may decrease due to climate change49,245,246 and interactions between solar UV radiation and climate change could accelerate this decline. Based on a biogeochemical elemental cycling model (without taking into account effects of solar UV radiation), Laufkoetter et al.49 simulated a decrease in phytoplankton net primary production by 6.5% within 50 years (1960–2006) on a global average, due to various climate-change related factors, including ocean stratification. A decrease in net primary production by phytoplankton is likely to reduce the efficiency of the biological pump and thus the CO2 sink strength of aquatic ecosystems. Even without a decrease in the global CO2 sink strength of terrestrial and aquatic ecosystems, their capacity to take up CO2 does not keep pace with increasing atmospheric CO2 concentrations.243,247,248
As discussed earlier, an important effect of solar UV-radiation in aquatic ecosystems is photobleaching of CDOM, resulting in increased transmission of the damaging solar UV-B radiation into water bodies with negative effects on photosynthetic organisms (organisms such as phytoplankton that transform CO2 into organic matter). The enhancement of UV-induced bleaching of CDOM due to stratification of water bodies therefore represents a UV-mediated, positive feedback on climate. In terrestrial ecosystems a positive feedback on climate is increased UV-induced decomposition and mineralisation of above ground litter (under release of CO2) due to aridification and decreased plant cover from human activity.
Important greenhouse gases other than CO2 are nitrous oxide (N2O) and methane (CH4). Climate change, via changing precipitation and temperature distributions and extreme events, can have large impacts on the emission of these gases. For example, N2O and CH4 emissions from plants may be increased through temperature effects, indirectly due to rising CO2 levels. UV-induced inhibition of microbial processes in aquatic systems can also influence trace gas exchange such as CO and N2O to air. Interactions in atmospheric chemistry also exist via UV-induced reactions involving N2O resulting in the formation of ozone destroying compounds.
Halocarbons such as methyl bromide (CH3Br), which are emitted by phytoplankton, are other gases that undergo UV-induced transformations to highly reactive species and thus also have the potential to cause stratospheric ozone depletion. Changes in oceanic phytoplankton distributions and community structure due to climate change is expected to alter rates of emission of these trace gases. Highly reactive species formed in UV-induced transformations of halocarbons also react with tropospheric pollutants, e.g. gaseous elemental mercury yielding the precursor of the bioavailable and thus toxic form of mercury. While there exists a large body of literature on the effects of climate change or of solar UV radiation on the fate of mercury, the interactive effects of climate change and solar UV radiation on the biogeochemical cycling of mercury have, to our knowledge, not yet been extensively studied.
Levels of organic pollutants and pathogens in aquatic environments are affected by interactions with changing climate and solar UV radiation. For example, UV radiation can inactivate pathogenic viruses by direct pathways and also by indirect pathways that involve sensitisation by CDOM. CDOM, a climate-sensitive component of most aquatic environments, can also modulate pathogen levels by screening out the UV component of sunlight.
The combined effects of solar UV radiation and climate change on biogeochemical cycles are likely to be particularly pronounced in the Arctic due to Arctic amplification that also hinders the recovery of the stratospheric ozone concentration in Arctic spring. As a consequence of Arctic stratospheric ozone depletion, increased levels of solar UV radiation reach Arctic terrestrial and aquatic ecosystems. Negative effects of solar UV-B radiation on phytoplankton and thus on the biological pump are expected in ice-free Arctic marine regions, particularly in combination with stratification due to increasing melt-water input. Based on a radiation transfer model, Fountoulakis et al. (2014)249 predict that up to 10 times higher levels of solar UV irradiance will enter large parts of the Arctic Ocean by 2100, compared to the 1950s, mainly due to Arctic sea-ice melting. Furthermore, increased emissions of CO2via mineralisation of organic matter due to thawing permafrost and Arctic tundra wildfires as well as decreased albedo due to vegetation shifts,11 have large effects on carbon cycling in the Arctic150 and together can act as a positive feedback on global warming. Therefore, more attention needs to be paid to Arctic tipping points.
Acknowledgements
D. E. was supported by the U.S. Global Change Research Program and by the Oak Ridge National Laboratory, which is supported by the Office of Science of the U.S. Department of Energy under Contract Number DE-AC05-00OR22725. B. S. was supported by Eawag: Swiss Federal Institute of Aquatic Science and Technology. R. Z. was supported by the National Exposure Research Laboratory, Ecosystems Research Division, U.S. Environmental Protection Agency, and A. A. was supported by grants from the National Agency of Science and Technology of Argentina (ANPCyT).
References
- R. L. McKenzie, P. J. Aucamp, A. F. Bais, L. O. Björn, M. Ilyas and S. Madronich, Ozone depletion and climate change: Impacts on UV radiation, Photochem. Photobiol. Sci., 2011, 10, 182–198 CAS.
- Y. Wu, L. M. Polvani and R. Seager, The importance of the Montreal Protocol in protecting Earth's hydroclimate, J. Climate, 2013, 26, 4049–4068 CrossRef.
- A. F. Bais, R. L. McKenzie, P. J. Aucamp, M. Ilyas, S. Madronich, G. Bernhard and K. Tourpali, Ozone depletion and climate change: Impacts on UV radiation, Photochem. Photobiol. Sci., 2015, 14 10.1039/c4pp90032d , this issue.
- J. Austin, H. Struthers, J. Scinocca, D. A. Plummer, H. Akiyoshi, A. J. G. Baumgaertner, S. Bekki, G. E. Bodeker, P. Braesicke, C. Bruehl, N. Butchart, M. P. Chipperfield, D. Cugnet, M. Dameris, S. Dhomse, S. Frith, H. Garny, A. Gettelman, S. C. Hardiman, P. Joeckel, D. Kinnison, A. Kubin, J. F. Lamarque, U. Langematz, E. Mancini, M. Marchand, M. Michou, O. Morgenstern, T. Nakamura, J. E. Nielsen, G. Pitari, J. Pyle, E. Rozanov, T. G. Shepherd, K. Shibata, D. Smale, H. Teyssedre and Y. Yamashita, Chemistry-climate model simulations of spring Antarctic ozone, J. Geophys. Res., [Atmos.], 2010, 115 Search PubMed.
- G. L. Manney, M. L. Santee, M. Rex, N. J. Livesey, M. C. Pitts, P. Veefkind, E. R. Nash, I. Wohltmann, R. Lehmann, L. Froidevaux, L. R. Poole, M. R. Schoeberl, D. P. Haffner, J. Davies, V. Dorokhov, H. Gernandt, B. Johnson, R. Kivi, E. Kyro, N. Larsen, P. F. Levelt, A. Makshtas, C. T. McElroy, H. Nakajima, M. C. Parrondo, D. W. Tarasick, P. von der Gathen, K. A. Walker and N. S. Zinoviev, Unprecedented Arctic ozone loss in 2011, Nature, 2011, 478, 469–U465 CrossRef CAS PubMed.
- B. M. Sinnhuber, G. Stiller, R. Ruhnke, T. von Clarmann, S. Kellmann and J. Aschmann, Arctic winter 2010/2011 at the brink of an ozone hole, Geophys. Res. Lett., 2011, 38 Search PubMed.
- G. Bernhard, A. Dahlback, V. Fioletov, A. Heikkila, B. Johnsen, T. Koskela, K. Lakkala and T. Svendby, High levels of ultraviolet radiation observed by ground-based instruments below the 2011 Arctic ozone hole, Atmos. Chem. Phys., 2013, 13, 10573–10590 Search PubMed.
- J. A. Screen and I. Simmonds, The central role of diminishing sea ice in recent Arctic temperature amplification, Nature, 2010, 464, 1334–1337 CrossRef CAS PubMed.
- F. Pithan and T. Mauritsen, Arctic amplification dominated by temperature feedbacks in contemporary climate models, Nat. Geosci., 2014, 7, 181–184 CrossRef CAS.
- R. F. Spielhagen, K. Werner, S. A. Sorensen, K. Zamelczyk, E. Kandiano, G. Budeus, K. Husum, T. M. Marchitto and M. Hald, Enhanced modern heat transfer to the arctic by warm Atlantic water, Science, 2011, 331, 450–453 CrossRef CAS PubMed.
- R. G. Pearson, S. J. Phillips, M. M. Loranty, P. S. A. Beck, T. Damoulas, S. J. Knight and S. J. Goetz, Shifts in Arctic vegetation and associated feedbacks under climate change, Nat. Clim. Change, 2013, 3, 673–677 CrossRef.
- T. M. Lenton, Arctic climate tipping points, Ambio, 2012, 41, 10–22 CrossRef PubMed.
-
IPCC, Climate Change 2013: The Physical Science Basis. Contribution of Working Group I to the Fifth Assessment Report of the Intergovernmental Panel on Climate Change, 2013.
- A. Shepherd, A reconciled estimate of ice-sheet mass balance (vol 57, pg 88, 2010), Science, 2012, 338, 1539–1539 CrossRef PubMed.
- J. E. Walsh, Melting ice what is happening to Arctic Sea Ice, and what does it mean for us?, Oceanography, 2013, 26, 171–181 CrossRef.
- D.-P. Häder, C. E. Williamson, S.-Å. Wängberg, M. Rautio, K. C. Rose, K. Gao, E. W. Helbling, R. P. Sinha and R. Worrest, Effects of UV radiation on aquatic ecosystems and interactions with other environmental factors, Photochem. Photobiol. Sci., 2015, 14 10.1039/c4pp90035a , this issue.
- I. Joughin, R. B. Alley and D. M. Holland, Ice-sheet response to oceanic forcing, Science, 2012, 338, 1172–1176 CrossRef CAS PubMed.
- K. Frey, D. Perovich and B. Light, The spatial distribution of solar radiation under a melting Arctic sea ice cover, Geophys. Res. Lett., 2011, 38, L22501 CrossRef.
- C. M. Moreno, Hydrogen peroxide production driven by UV-B in planktonic microorganisms: a photocatalytic factor in sea warming and ice melting in regions with ozone depletion?, Biogeochemistry, 2012, 107, 1–8 CrossRef CAS.
- J. Sedlacek, R. Knutti, O. Martius and U. Beyerle, Impact of reduced Arctic Sea ice cover on ocean and atmospheric properties, J. Clim., 2012, 25, 307–319 CrossRef.
- J. A. Francis, The where and when of wetter and drier: disappearing Arctic sea ice plays a role, Environ. Res. Lett., 2013, 8 Search PubMed.
- J. A. Francis and S. J. Vavrus, Evidence linking Arctic amplification to extreme weather in mid-latitudes, Geophys. Res. Lett., 2012, 39 Search PubMed.
- S. Haekkinen, P. B. Rhines and D. L. Worthen, Atmospheric blocking and Atlantic multidecadal ocean variability, Science, 2011, 334, 655–659 CrossRef CAS PubMed.
- J. Liu, J. A. Curry, H. Wang, M. Song and R. M. Horton, Impact of declining Arctic sea ice on winter snowfall (vol 109, pg 4074, 2012), Proc. Natl. Acad. Sci. U. S. A., 2012, 109, 6781–6783 CrossRef CAS.
- Q. Tang, X. Zhang, X. Yang and J. A. Francis, Cold winter extremes in northern continents linked to Arctic sea ice loss, Environ. Res. Lett., 2013, 8 Search PubMed.
- A. Hu, G. A. Meehl, W. Han, J. Lu and W. G. Strand, Energy balance in a warm world without the ocean conveyor belt and sea ice, Geophys. Res. Lett., 2013, 40, 6242–6246 CrossRef.
- S. Lee and S. B. Feldstein, Detecting ozone- and greenhouse gas-driven wind trends with observational data, Science, 2013, 339, 563–567 CrossRef CAS PubMed.
- H. Paeth and F. Pollinger, Enhanced evidence in climate models for changes in extratropical atmospheric circulation, Tellus Ser. A, 2010, 62, 647–660 Search PubMed.
- I. R. Young, S. Zieger and A. V. Babanin, Global trends in wind speed and wave height, Science, 2011, 332, 451–455 CrossRef CAS PubMed.
- N. P. Gillett and D. W. J. Thompson, Simulation of recent Southern Hemisphere climate change, Science, 2003, 302, 273–275 CrossRef CAS PubMed.
- G. J. Marshall, Trends in the southern annular mode from observations and reanalyses, J. Clim., 2003, 16, 4134–4143 CrossRef.
- G. J. Marshall, S. Di Battista, S. S. Naik and M. Thamban, Analysis of a regional change in the sign of the SAM-temperature relationship in Antarctica, Clim. Dyn., 2011, 36, 277–287 CrossRef.
- S. M. Kang, L. M. Polvani, J. C. Fyfe and M. Sigmond, Impact of polar ozone depletion on subtropical precipitation, Science, 2011, 332, 951–954 CrossRef CAS PubMed.
- N. Gruber, Warming up, turning sour, losing breath: ocean biogeochemistry under global change, Philos. Trans. R. Soc. London, Ser. A, 2011, 369, 1980–1996 CrossRef CAS PubMed.
- T. Ito, M. Woloszyn and M. Mazloff, Anthropogenic carbon dioxide transport in the Southern Ocean driven by Ekman flow, Nature, 2010, 463, 80–U85 CrossRef CAS PubMed.
- A. Lenton, F. Codron, L. Bopp, N. Metzl, P. Cadule, A. Tagliabue and J. Le Sommer, Stratospheric ozone depletion reduces ocean carbon uptake and enhances ocean acidification, Geophys. Res. Lett., 2009, 36 Search PubMed.
- D. W. Waugh, F. Primeau, T. DeVries and M. Holzer, Recent changes in the ventilation of the southern oceans, Science, 2013, 339, 568–570 CrossRef CAS PubMed.
- A. Burke and L. F. Robinson, The southern ocean's role in carbon exchange during the last deglaciation, Science, 2012, 335, 557–561 CrossRef CAS PubMed.
- K. A. Rose, E. L. Sikes, T. P. Guilderson, P. Shane, T. M. Hill, R. Zahn and H. J. Spero, Upper-ocean-to-atmosphere radiocarbon offsets imply fast deglacial carbon dioxide release, Nature, 2010, 466, 1093–1097 CrossRef CAS PubMed.
- D. M. Sigman, M. P. Hain and G. H. Haug, The polar ocean and glacial cycles in atmospheric CO2 concentration, Nature, 2010, 466, 47–55 CrossRef CAS PubMed.
- T. Tschumi, F. Joos, M. Gehlen and C. Heinze, Deep ocean ventilation, carbon isotopes, marine sedimentation and the deglacial CO2 rise, Clim. Past, 2011, 7, 771–800 CrossRef.
- S. A. Robinson and D. J. Erickson, Not just about sunburn - the ozone hole's profound effect on climate has significant implications for Southern Hemisphere ecosystems, Global Change Biol., 2014 DOI:10.1111/gcb.12739.
- R. Gangsto, F. Joos and M. Gehlen, Sensitivity of pelagic calcification to ocean acidification, Biogeosciences, 2011, 8, 433–458 CrossRef CAS.
- N. E. Cantin, A. L. Cohen, K. B. Karnauskas, A. M. Tarrant and D. C. McCorkle, Ocean warming slows coral growth in the central Red Sea, Science, 2010, 329, 322–325 CrossRef CAS PubMed.
- C. Hauri, N. Gruber, A. M. P. McDonnell and M. Vogt, The intensity, duration, and severity of low aragonite saturation state events on the California continental shelf, Geophys. Res. Lett., 2013, 40, 3424–3428 CrossRef.
- J. M. Pandolfi, S. R. Connolly, D. J. Marshall and A. L. Cohen, Projecting coral reef futures under global warming and ocean acidification, Science, 2011, 333, 418–422 CrossRef CAS PubMed.
- S. Sinutok, R. Hill, M. A. Doblin, R. Wuhrer and P. J. Ralph, Warmer more acidic conditions cause decreased productivity and calcification in subtropical coral reef sediment-dwelling calcifiers, Limnol. Oceanogr., 2011, 56, 1200–1212 CrossRef CAS.
- J. M. Shick, K. Iglic, M. L. Wells, C. G. Trick, J. Doyle and W. C. Dunlap, Responses to iron limitation in two colonies of Stylophora pistillata exposed to high temperature: Implications for coral bleaching, Limnol. Oceanogr., 2011, 56, 813–828 CrossRef CAS.
- C. Laufkoetter, M. Vogt and N. Gruber, Long-term trends in ocean plankton production and particle export between 1960–2006, Biogeosciences, 2013, 10, 7373–7393 CrossRef.
- R. P. Allan, Human influence on rainfall, Nature, 2011, 470, 344–341 CrossRef CAS PubMed.
- S. B. Feldstein, Subtropical rainfall and the Antarctic ozone hole, Science, 2011, 332, 925–926 CrossRef CAS PubMed.
- S.-K. Min, X. Zhang, F. W. Zwiers and G. C. Hegerl, Human contribution to more-intense precipitation extremes, Nature, 2011, 470, 378–379 CrossRef CAS PubMed.
- P. Pall, T. Aina, D. A. Stone, P. A. Stott, T. Nozawa, A. G. J. Hilberts, D. Lohmann and M. R. Allen, Anthropogenic greenhouse gas contribution to flood risk in England and Wales in autumn 2000, Nature, 2011, 470, 382–384 CrossRef CAS PubMed.
- W. Cai, M. Lengaigne, S. Borlace, M. Collins, T. Cowan, M. J. McPhaden, A. Timmermann, S. Power, J. Brown, C. Menkes, A. Ngari, E. M. Vincent and M. J. Widlansky, More extreme swings of the South Pacific convergence zone due to greenhouse warming, Nature, 2012, 488, 365 CrossRef CAS PubMed.
- D. Coumou and S. Rahmstorf, A decade of weather extremes, Nat. Clim. Change, 2012, 2, 491–496 Search PubMed.
- P. Kopparla, E. M. Fischer, C. Hannay and R. Knutti, Improved simulation of extreme precipitation in a high-resolution atmosphere model, Geophys. Res. Lett., 2013, 40, 5803–5808 CrossRef.
- A. M. Bass, M. I. Bird, M. J. Liddell and P. N. Nelson, Fluvial dynamics of dissolved and particulate organic carbon during periodic discharge events in a steep tropical rainforest catchment, Limnol. Oceanogr., 2011, 56, 2282–2292 CrossRef CAS.
- S. Haaland, D. Hongve, H. Laudon, G. Riise and R. D. Vogt, Quantifying the drivers of the increasing colored organic matter in boreal surface waters, Environ. Sci. Technol., 2010, 44, 2975–2980 CrossRef CAS PubMed.
- C. A. Stedmon, R. M. W. Amon, A. J. Rinehart and S. A. Walker, The supply and characteristics of colored dissolved organic matter (CDOM) in the Arctic Ocean: Pan Arctic trends and differences, Mar. Chem., 2011, 124, 108–118 CrossRef CAS PubMed.
- J. Zhang, J. Hudson, R. Neal, J. Sereda, T. Clair, M. Turner, D. Jeffries, P. Dillon, L. Molot, K. Somers and R. Hesslein, Long-term patterns of dissolved organic carbon in lakes across eastern Canada: Evidence of a pronounced climate effect, Limnol. Oceanogr., 2010, 55, 30–42 CrossRef CAS.
- B. B. Barnes, C. Hu, J. P. S. E. Cannizzaro, S. E. P. Craig, P. Hallock, D. L. Jones, J. C. N. Lehrter, N. Melo, B. A. Schaeffer and R. Zepp, Estimation of diffuse attenuation of ultraviolet light in optically shallow Florida Keys waters from MODIS measurements, Remote Sens. Environ., 2014, 140, 519–532 CrossRef PubMed.
- S. Bekki, A. Rap, V. Poulain, S. Dhomse, M. Marchand, F. Lefevre, P. M. Forster, S. Szopa and M. P. Chipperfield, Climate impact of stratospheric ozone recovery, Geophys. Res. Lett., 2013, 40, 2796–2800 CrossRef CAS.
- J. E. Halofsky, M. A. Hemstrom, D. R. Conklin, J. S. Halofsky, B. K. Kerns and D. Bachelet, Assessing potential climate change effects on vegetation using a linked model approach, Ecol. Modell., 2013, 266, 131–143 CrossRef PubMed.
- T. Hickler, K. Vohland, J. Feehan, P. A. Miller, B. Smith, L. Costa, T. Giesecke, S. Fronzek, T. R. Carter, W. Cramer, I. Kuehn and M. T. Sykes, Projecting the future distribution of European potential natural vegetation zones with a generalized, tree species-based dynamic vegetation model, Global Ecol. Biogeogr., 2012, 21, 50–63 CrossRef PubMed.
- H. W. Polley, V. L. Jin and P. A. Fay, CO2-caused change in plant species composition rivals the shift in vegetation between mid-grass and tallgrass prairies, Global Change Biol., 2012, 18, 700–710 CrossRef PubMed.
- E. A. Davidson, A. C. de Araujo, P. Artaxo, J. K. Balch, I. F. Brown, M. M. C. Bustamante, M. T. Coe, R. S. DeFries, M. Keller, M. Longo, J. W. Munger, W. Schroeder, B. S. Soares-Filho, C. M. Souza and S. C. Wofsy, The Amazon basin in transition (vol 481, pg 321, 2012), Nature, 2012, 483, 232–232 CrossRef CAS.
- M. Gottfried, H. Pauli, A. Futschik, M. Akhalkatsi, P. Barancok, J. L. Benito Alonso, G. Coldea, J. Dick, B. Erschbamer, M. R. Fernandez Calzado, G. Kazakis, J. Krajci, P. Larsson, M. Mallaun, O. Michelsen, D. Moiseev, P. Moiseev, U. Molau, A. Merzouki, L. Nagy, G. Nakhutsrishvili, B. Pedersen, G. Pelino, M. Puscas, G. Rossi, A. Stanisci, J.-P. Theurillat, M. Tomaselli, L. Villar, P. Vittoz, I. Vogiatzakis and G. Grabherr, Continent-wide response of mountain vegetation to climate change, Nat. Clim. Change, 2012, 2, 111–115 CrossRef.
- C. Beer, M. Reichstein, E. Tomelleri, P. Ciais, M. Jung, N. Carvalhais, C. Roedenbeck, M. A. Arain, D. Baldocchi, G. B. Bonan, A. Bondeau, A. Cescatti, G. Lasslop, A. Lindroth, M. Lomas, S. Luyssaert, H. Margolis, K. W. Oleson, O. Roupsard, E. Veenendaal, N. Viovy, C. Williams, F. I. Woodward and D. Papale, Terrestrial Gross Carbon Dioxide Uptake: Global Distribution and Covariation with Climate, Science, 2010, 329, 834–838 CrossRef CAS PubMed.
- B. Choat, S. Jansen, T. J. Brodribb, H. Cochard, S. Delzon, R. Bhaskar, S. J. Bucci, T. S. Feild, S. M. Gleason, U. G. Hacke, A. L. Jacobsen, F. Lens, H. Maherali, J. Martinez-Vilalta, S. Mayr, M. Mencuccini, P. J. Mitchell, A. Nardini, J. Pittermann, R. B. Pratt, J. S. Sperry, M. Westoby, I. J. Wright and A. E. Zanne, Global convergence in the vulnerability of forests to drought, Nature, 2012, 491, 752–755 CAS.
- C. R. Schwalm, C. A. Williams, K. Schaefer, D. Baldocchi, T. A. Black, A. H. Goldstein, B. E. Law, W. C. Oechel, U. Kyaw Tha Paw and R. L. Scott, Reduction in carbon uptake during turn of the century drought in western North America, Nat. Geosci., 2012, 5, 551–556 CrossRef CAS.
- M. S. Zhao and S. W. Running, Drought-Induced reduction in global terrestrial net primary production from 2000 through 2009, Science, 2010, 329, 940–943 CrossRef CAS PubMed.
- J. F. Bornman, P. W. Barnes, S. A. Robinson, C. L. Ballaré, S. D. Flinte and M. M. Caldwell, Solar ultraviolet radiation and ozone depletion-driven climate change: effects on terrestrial ecosystems, Photochem. Photobiol. Sci., 2015, 14 10.1039/c4pp90034k , this issue.
- J. Bodin, V. Badeau, E. Bruno, C. Cluzeau, J.-M. Moisselin, G.-R. Walther and J.-L. Dupouey, Shifts of forest species along an elevational gradient in Southeast France: climate change or stand maturation?, J. Veg. Sci., 2013, 24, 269–283 CrossRef PubMed.
- J. J. Wargent and B. R. Jordan, From ozone depletion to agriculture: understanding the role of UV radiation in sustainable crop production, New Phytol., 2013, 197, 1058–1076 CrossRef CAS PubMed.
- D. S. Ward, S. Kloster, N. M. Mahowald, B. M. Rogers, J. T. Randerson and P. G. Hess, The changing radiative forcing of fires: global model estimates for past, present and future, Atmos. Chem. Phys., 2012, 12, 10857–10886 CrossRef CAS.
- D. A. King, D. M. Bachelet and A. J. Symstad, Climate change and fire effects on a prairie-woodland ecotone: projecting species range shifts with a dynamic global vegetation model, Ecol. Evol., 2013, 3, 5076–5097 CrossRef PubMed.
- T. Dittmar, C. E. de Rezende, M. Manecki, J. Niggemann, A. R. C. Ovalle, A. Stubbins and M. C. Bernardes, Continuous flux of dissolved black carbon from a vanished tropical forest biome, Nat. Geosci., 2012, 5, 618–622 CrossRef CAS.
- R. Jaffe, Y. Ding, J. Niggemann, A. V. Vahatalo, A. Stubbins, R. G. M. Spencer, J. Campbell and T. Dittmar, Global charcoal mobilization from soils via dissolution and riverine transport to the oceans, Science, 2013, 340, 345–347 CrossRef CAS PubMed.
- C. A. Masiello and P. Louchouarn, Fire in the ocean, Science, 2013, 340, 287–288 CrossRef CAS PubMed.
- A. Stubbins, J. Niggemann and T. Dittmar, Photo-lability of deep ocean dissolved black carbon, Biogeosciences, 2012, 9, 1661–1670 CrossRef CAS.
- B. W. Brook, E. C. Ellis, M. P. Perring, A. W. Mackay and L. Blomqvist, Does the terrestrial biosphere have planetary tipping points?, Trends Ecol. Evol., 2013, 28, 396–401 CrossRef PubMed.
- M. S. Bret-Harte, M. C. Mack, G. R. Shaver, D. C. Huebner, M. Johnston, C. A. Mojica, C. Pizano and J. A. Reiskind, The response of Arctic vegetation and soils following an unusually severe tundra fire, Philos. Trans. R. Soc. London, B., 2013, 368 Search PubMed.
- F. Lloret, A. Escudero, J. Maria Iriondo, J. Martinez-Vilalta and F. Valladares, Extreme climatic events and vegetation: the role of stabilizing processes, Global Change Biol., 2012, 18, 797–805 CrossRef PubMed.
- A. L. S. Swann, I. Y. Fung and J. C. H. Chiang, Mid-latitude afforestation shifts general circulation and tropical precipitation, Proc. Natl. Acad. Sci. U. S. A., 2012, 109, 712–716 CrossRef CAS PubMed.
- G. F. Clark, J. S. Stark, E. L. Johnston, J. W. Runcie, P. M. Goldsworthy, B. Raymond and M. J. Riddle, Light-driven tipping points in polar ecosystems, Global Change Biol., 2013, 19, 3749–3761 CrossRef PubMed.
- M. Ribas-Ribas, A. Gomez-Parra and J. M. Forja, Air-sea CO2 fluxes in the north-eastern shelf of the Gulf of Cadiz (southwest Iberian Peninsula), Mar. Chem., 2011, 123, 56–66 CrossRef CAS PubMed.
- E. H. Shadwick, H. Thomas, K. Azetsu-Scott, B. J. W. Greenan, E. Head and E. Horne, Seasonal variability of dissolved inorganic carbon and surface water pCO2 in the Scotian Shelf region of the Northwestern Atlantic, Mar. Chem., 2011, 124, 23–37 CrossRef CAS PubMed.
- D.-P. Häder, E. W. Helbling, C. E. Williamson and R. C. Worrest, Effects on aquatic ecosystems and interactions with climate change, Photochem. Photobiol. Sci., 2011, 10, 242–260 Search PubMed.
- R. G. Zepp, D. J. Erickson III, N. D. Paul and B. Sulzberger, Effects of solar UV radiation and climate change on biogeochemical cycling: interactions and feedbacks, Photochem. Photobiol. Sci., 2011, 10, 261–279 CAS.
-
N. B. Nelson and D. A. Siegel, The global distribution and dynamics of chromophoric dissolved organic matter, in Annual Review of Marine Science, ed. C. A. Carlson and S. J. Giovannoni, Annual Reviews, Palo Alto, 2013, vol. 5, pp. 447–476 Search PubMed.
- R. G. M. Spencer, G. R. Aiken, M. M. Dornblaser, K. D. Butler, R. M. Holmes, G. Fiske, P. J. Mann and A. Stubbins, Chromophoric dissolved organic matter export from U.S. rivers, Geophys. Res. Lett., 2013, 40, 1575–1579 CrossRef CAS.
- A. Bricaud, A. M. Ciotti and B. Gentili, Spatial-temporal variations in phytoplankton
size and colored detrital matter absorption at global and regional scales, as derived from twelve years of SeaWiFS data (1998–2009), Global Biogeochem. Cycles, 2012, 26 Search PubMed.
- M. Gonsior, P. Schmitt-Kopplin and D. Bastviken, Depth-dependent molecular composition and photo-reactivity of dissolved organic matter in a boreal lake under winter and summer conditions, Biogeosciences, 2013, 10, 6945–6956 CrossRef CAS.
- M. A. Granskog, C. A. Stedmon, P. A. Dodd, R. M. W. Amon, A. K. Pavlov, L. de Steur and E. Hansen, Characteristics of colored dissolved organic matter (CDOM) in the Arctic outflow in the Fram Strait: Assessing the changes and fate of terrigenous CDOM in the Arctic Ocean, J. Geophys. Res.: Oceans, 2012, 117 Search PubMed.
- J. R. Helms, A. Stubbins, E. M. Perdue, N. W. Green, H. Chen and K. Mopper, Photochemical bleaching of oceanic dissolved organic matter and its effect on absorption spectral slope and fluorescence, Mar. Chem., 2013, 155, 81–91 CrossRef CAS PubMed.
- G. C. Shank, R. G. Zepp, A. Vahatalo, R. Lee and E. Bartels, Photobleaching kinetics of chromophoric dissolved organic matter derived from mangrove leaf litter and floating Sargassum colonies, Mar. Chem., 2010, 119, 162–171 CrossRef CAS PubMed.
- C. M. Swan, N. B. Nelson, D. A. Siegel and T. S. Kostadinov, The effect of surface irradiance on the absorption spectrum of chromophoric dissolved organic matter in the global ocean, Deep-Sea Res., Part I, 2012, 63, 52–64 CrossRef CAS PubMed.
- Y. Yamashita, Y. Nosaka, K. Suzuki, H. Ogawa, K. Takahashi and H. Saito, Photobleaching as a factor controlling spectral characteristics of chromophoric dissolved organic matter in open ocean, Biogeosciences, 2013, 10, 7207–7217 CrossRef CAS.
- L. Bracchini, A. M. Dattilo, V. Hull, S. A. Loiselle, L. Nannicini, M. P. Picchi, M. Ricci, C. Santinelli, A. Seritti, A. Tognazzi and C. Rossi, Spatial and seasonal changes in optical properties of autochthonous and allochthonous chromophoric dissolved organic matter in a stratified mountain lake, Photochem. Photobiol. Sci., 2010, 9, 304–314 CAS.
- V. E. Villafane, A. T. Banaszak, S. D. Guendulain-Garcia, S. M. Strauch, S. R. Halac and E. W. Helbling, Influence of seasonal variables associated with climate change on photochemical diurnal cycles of marine phytoplankton from Patagonia (Argentina), Limnol. Oceanogr., 2013, 58, 203–214 CrossRef.
- D. G. Boyce, M. R. Lewis and B. Worm, Global phytoplankton decline over the past century, Nature, 2010, 466, 591–596 CrossRef CAS PubMed.
- W. J. Cai, L. Q. Chen, B. S. Chen, Z. Y. Gao, S. H. Lee, J. F. Chen, D. Pierrot, K. Sullivan, Y. C. Wang, X. P. Hu, W. J. Huang, Y. H. Zhang, S. Q. Xu, A. Murata, J. M. Grebmeier, E. P. Jones and H. S. Zhang, Decrease in the CO2 uptake capacity in an ice-free Arctic Ocean basin, Science, 2010, 329, 556–559 CrossRef CAS PubMed.
- C. G. Fichot, K. Kaiser, S. B. Hooker, R. M. W. Amon, M. Babin, S. Belanger, S. A. Walker and R. Benner, Pan-Arctic distributions of continental runoff in the Arctic Ocean, Sci. Rep., 2013, 3 Search PubMed.
- M. A. Granskog, Changes in spectral slopes of colored dissolved organic matter absorption with mixing and removal in a terrestrially dominated marine system (Hudson Bay, Canada), Mar. Chem., 2012, 134, 10–17 CrossRef PubMed.
- H. E. Reader and W. L. Miller, Variability of carbon monoxide and carbon dioxide apparent quantum yield spectra in three coastal estuaries of the South Atlantic Bight, Biogeosci. Discuss., 2012, 9, 6927–6985 Search PubMed.
- N. B. Nelson, D. A. Siegel, C. A. Carlson and C. M. Swan, Tracing global biogeochemical cycles and meridional overturning circulation using chromophoric dissolved organic matter, Geophys. Res. Lett., 2010, 37 Search PubMed.
- P. Falkowski, Ocean
Science: The power of plankton, Nature, 2012, 483, S17–S20 CrossRef CAS PubMed.
- M. Hofmann and H.-J. Schellnhuber, Oceanic acidification affects marine carbon pump and triggers extended marine oxygen holes, Proc. Natl. Acad. Sci. U. S. A., 2009, 106, 3017–3022 CrossRef CAS PubMed.
- M. Hofmann and H. J. Schellnhuber, Ocean acidification: a millennial challenge, Energy Environ. Sci., 2010, 3, 1883–1896 CAS.
- C. L. Ballaré, M. M. Caldwell, S. D. Flint, A. Robinson and J. F. Bornman, Effects of solar ultraviolet radiation on terrestrial ecosystems. Patterns, mechanisms, and interactions with climate change, Photochem. Photobiol. Sci., 2011, 10, 226–241 Search PubMed.
- A. T. Austin, Has water limited our imagination for arid land biogeochemistry?, Trends Ecol. Evol., 2011, 26, 229–235 CrossRef PubMed.
- L. A. Brandt, J. Y. King, S. E. Hobbie, D. G. Milchunas and R. L. Sinsabaugh, The Role of Photodegradation in Surface Litter Decomposition Across a Grassland Ecosystem Precipitation Gradient, Ecosystems, 2010, 13, 765–781 CrossRef CAS.
- J. A. Hatala, M. Detto, O. Sonnentag, S. J. Deverel, J. Verfaillie and D. D. Baldocchi, Greenhouse gas (CO2, CH4, H2O) fluxes from drained and flooded agricultural peatlands in the Sacramento-San Joaquin Delta, Agric. Ecosyst. Environ., 2012, 150, 1–18 CrossRef CAS PubMed.
- S. Rutledge, D. Campbell, D. Baldocchi and L. Schipper, Photodegradation leads to increased carbon dioxide losses from terrestrial organic matter, Global Change Biol., 2010, 16, 3065–3074 Search PubMed.
- B. Bond-Lamberty and A. Thomson, Temperature-associated increases in the global soil respiration record, Nature, 2010, 464, 579–U132 CrossRef CAS PubMed.
- S. Ma, D. D. Baldocchi, J. A. Hatala, M. Detto and J. Curiel Yuste, Are rain-induced ecosystem respiration pulses enhanced by legacies of antecedent photodegradation in semi-arid environments?, Agric. Forest Meteorol., 2012, 154, 203–213 CrossRef PubMed.
- W. K. Smith, W. Gao, H. Steltzer, M. D. Wallenstein and R. Tree, Moisture availability influences the effect of ultraviolet-B radiation on leaf litter decomposition, Global Change Biol., 2010, 16, 484–495 CrossRef PubMed.
- A. T. Austin and C. L. Ballaré, Dual role of lignin in plant litter decomposition in terrestrial ecosystems, Proc. Natl. Acad. Sci. U. S. A., 2010, 107, 4618–4622 CrossRef CAS PubMed.
- R. M. Cory, B. C. Crump, J. A. Dobkowski and G. W. Kling, Surface exposure to sunlight stimulates CO2 release from permafrost soil carbon in the Arctic, Proc. Natl. Acad. Sci. U. S. A., 2013, 110, 3429–3434 CrossRef CAS PubMed.
- X. Feng, K. M. Hills, A. J. Simpson, J. K. Whalen and M. J. Simpson, The role of biodegradation and photo-oxidation in the transformation of terrigenous organic matter, Org. Geochem., 2011, 42, 262–274 CrossRef CAS PubMed.
- J. Frouz, T. Cajthaml and O. Mudrak, The effect of lignin photodegradation on decomposability of Calamagrostis epigeios grass litter, Biodegradation, 2011, 22, 1247–1254 CrossRef CAS PubMed.
- J. Y. King, L. A. Brandt and E. C. Adair, Shedding light on plant litter decomposition: advances, implications and new directions in understanding the role of photodegradation, Biogeochemistry, 2012, 111, 57–81 CrossRef.
- H. Lee, T. Rahn and H. L. Throop, An accounting of C-based trace gas release during abiotic plant litter degradation, Global Change Biol., 2012, 18, 1185–1195 CrossRef PubMed.
- L. M. Mayer, K. R. Thornton, L. L. Schick, J. D. Jastrow and J. W. Harden, Photodissolution of soil organic matter, Geoderma, 2012, 170, 314–321 CrossRef CAS PubMed.
- B. Foereid, J. Bellarby, W. Meier-Augenstein and H. Kemp, Does light exposure make plant litter more degradable?, Plant Soil, 2010, 333, 275–285 CrossRef CAS PubMed.
- H. A. L. Henry, K. Brizgys and C. B. Field, Litter decomposition in a
California annual grassland: Interactions between photodegradation and litter layer thickness, Ecosystems, 2008, 11, 545–554 CrossRef CAS PubMed.
- B. Sulzberger and E. Durisch-Kaiser, Chemical characterization of dissolved organic matter (DOM): A prerequisite for understanding UV-induced changes of DOM absorption properties and bioavailability, Aquat. Sci., 2009, 71, 104–126 CrossRef CAS PubMed.
- M. C. Mack, M. S. Bret-Harte, T. N. Hollingsworth, R. R. Jandt, E. A. G. Schuur, G. R. Shaver and D. L. Verbyla, Carbon loss from an unprecedented Arctic tundra wildfire, Nature, 2011, 475, 489–492 CrossRef CAS PubMed.
- M. Fernanda Adame, S. F. Wright, A. Grinham, K. Lobb, C. E. Reymond and C. E. Lovelock, Terrestrial-marine connectivity: Patterns of terrestrial soil carbon deposition in coastal sediments determined by analysis of glomalin related soil protein, Limnol. Oceanogr., 2012, 57, 1492–1502 CrossRef.
- C. G. Fichot and R. Benner, The spectral slope coefficient of chromophoric dissolved organic matter (S275–295) as a tracer of terrigenous dissolved organic carbon in river-influenced ocean margins, Limnol. Oceanogr., 2012, 57, 1453–1466 CrossRef CAS.
- M. Gonsior, B. M. Peake, W. T. Cooper, D. C. Podgorski, J. D'Andrilli, T. Dittmar and W. J. Cooper, Characterization of dissolved organic matter across the Subtropical Convergence off the South Island, New Zealand, Mar. Chem., 2011, 123, 99–110 CrossRef CAS PubMed.
- R. T. Letscher, D. A. Hansell and D. Kadko, Rapid removal of terrigenous dissolved organic carbon over the Eurasian shelves of the Arctic Ocean, Mar. Chem., 2011, 123, 78–87 CrossRef CAS PubMed.
- C. J. Mundy, M. Gosselin, M. Starr and C. Michel, Riverine export and the effects of circulation on dissolved organic carbon in the Hudson Bay system, Canada, Limnol. Oceanogr., 2010, 55, 315–323 CrossRef CAS.
- B. A. Bergamaschi, D. P. Krabbenhoft, G. R. Aiken, E. Patino, D. G. Rumbold and W. H. Orem, Tidally Driven Export of Dissolved Organic Carbon, Total Mercury, and Methylmercury from a Mangrove-Dominated Estuary, Environ. Sci. Technol., 2012, 46, 1371–1378 CrossRef CAS PubMed.
- D. Olefeldt, K. J. Devito and M. R. Turetsky, Sources and fate of terrestrial dissolved organic carbon in lakes of a Boreal Plains region recently affected by wildfire, Biogeosciences, 2013, 10, 6247–6265 CAS.
- A. Stubbins, R. G. M. Spencer, H. Chen, P. G. Hatcher, K. Mopper, P. J. Hernes, V. L. Mwamba, A. M. Mangangu, J. N. Wabakanghanzi and J. Six, Illuminated darkness: Molecular signatures of Congo River dissolved organic matter and its photochemical alteration as revealed by ultrahigh precision mass spectrometry, Limnol. Oceanogr., 2010, 55, 1467–1477 CAS.
- E. S. Boyle, N. Guerriero, A. Thiallet, R. Del Vecchio and N. V. Blough, Optical properties of humic substances and CDOM: Relation to structure, Environ. Sci. Technol., 2009, 43, 2262–2268 CrossRef CAS.
- L. Jorgensen, C. A. Stedmon, T. Kragh, S. Markager, M. Middelboe and M. Sondergaard, Global trends in the fluorescence characteristics and distribution of marine dissolved organic matter, Mar. Chem., 2011, 126, 139–148 CrossRef CAS PubMed.
- J. F. Rontani, N. Zabeti and S. G. Wakeham, Degradation of particulate organic matter in the equatorial Pacific Ocean: Biotic or abiotic?, Limnol. Oceanogr., 2011, 56, 333–349 CrossRef CAS.
- E. M. White, D. J. Kieber, J. Sherrard, W. L. Miller and K. Mopper, Carbon dioxide and carbon monoxide photoproduction quantum yields in the Delaware Estuary, Mar. Chem., 2010, 118, 11–21 CrossRef CAS PubMed.
- H. Aarnos, P. Ylostalo and A. V. Vahatalo, Seasonal phototransformation of dissolved organic matter to ammonium, dissolved inorganic carbon, and labile substrates supporting bacterial biomass across the Baltic Sea, J. Geophys. Res., 2012, 117 DOI:10.1029/2010jg001633.
- R. M. Cory and L. A. Kaplan, Biological lability of streamwater fluorescent dissolved organic matter, Limnol. Oceanogr., 2012, 57, 1347–1360 CrossRef CAS.
- C. Romera-Castillo, M. Nieto-Cid, C. G. Castro, C. Marrase, J. Largier, E. D. Barton and X. A. Alvarez-Salgado, Fluorescence: Absorption coefficient ratio - Tracing photochemical and microbial degradation processes affecting coloured dissolved organic matter in a coastal system, Mar. Chem., 2011, 125, 26–38 CrossRef CAS PubMed.
- R. M. DeConto, S. Galeotti, M. Pagani, D. Tracy, K. Schaefer, T. Zhang, D. Pollard and D. J. Beerling, Past extreme warming events linked to massive carbon release from thawing permafrost (vol 484, pg 87, 2012), Nature, 2012, 490, 292–292 CrossRef CAS.
- J. B. Fellman, E. Hood and R. G. M. Spencer, Fluorescence spectroscopy opens new windows into dissolved organic matter dynamics in freshwater ecosystems: A review, Limnol. Oceanogr., 2010, 55, 2452–2462 CrossRef CAS.
- B. G. Pautler, G. C. Woods, A. Dubnick, A. J. Simpson, M. J. Sharp, S. J. Fitzsimons and M. J. Simpson, Molecular characterization of dissolved organic matter in glacial ice: Coupling natural abundance H-1 NMR and fluorescence spectroscopy, Environ. Sci. Technol., 2012, 46, 3753–3761 CrossRef CAS PubMed.
- C. M. Moore, M. M. Mills, K. R. Arrigo, I. Berman-Frank, L. Bopp, P. W. Boyd, E. D. Galbraith, R. J. Geider, C. Guieu, S. L. Jaccard, T. D. Jickells, J. La Roche, T. M. Lenton, N. M. Mahowald, E. Maranon, I. Marinov, J. K. Moore, T. Nakatsuka, A. Oschlies, M. A. Saito, T. F. Thingstad, A. Tsuda and O. Ulloa, Processes and patterns of oceanic nutrient limitation, Nat. Geosci., 2013, 6, 701–710 CrossRef CAS.
- P. Regnier, P. Friedlingstein, P. Ciais, F. T. Mackenzie, N. Gruber, I. A. Janssens, G. G. Laruelle, R. Lauerwald, S. Luyssaert, A. J. Andersson, S. Arndt, C. Arnosti, A. V. Borges, A. W. Dale, A. Gallego-Sala, Y. Godderis, N. Goossens, J. Hartmann, C. Heinze, T. Ilyina, F. Joos, D. E. LaRowe, J. Leifeld, F. J. R. Meysman, G. Munhoven, P. A. Raymond, R. Spahni, P. Suntharalingam and M. Thullner, Anthropogenic perturbation of the carbon fluxes from land to ocean, Nat. Geosci., 2013, 6, 597–607 CrossRef CAS.
- I. Valiela, L. Camilli, T. Stone, A. Giblin, J. Crusius, S. Fox, C. Barth-Jensen, R. O. Monteiro, J. Tucker, P. Martinetto and C. Harris, Increased rainfall remarkably freshens estuarine and coastal waters on the Pacific coast of Panama: Magnitude and likely effects on upwelling and nutrient supply, Global Planet. Change, 2012, 92–93, 130–137 CrossRef PubMed.
- L. Tranvik, Carbon cycling in the Arctic, Science, 2014, 345, 870 CrossRef CAS PubMed.
- K. S. Johnson, S. C. Riser and D. M. Karl, Nitrate supply from deep to near-surface waters of the North Pacific subtropical gyre, Nature, 2010, 465, 1062–1065 CrossRef CAS PubMed.
- A. L. King and K. A. Barbeau, Dissolved iron and macronutrient distributions in the southern California Current System, J. Geophys. Res. Oceans, 2011, 116 Search PubMed.
- A. L. King, K. N. Buck and K. A. Barbeau, Quasi-Lagrangian drifter studies of iron speciation and cycling off Point Conception, California, Mar. Chem., 2012, 128, 1–12 CrossRef PubMed.
- A. Lelong, E. Bucciarelli, H. Hegaret and P. Soudant, Iron and copper limitations differently affect growth rates and photosynthetic and physiological parameters of the marine diatom Pseudonitzschia delicatissima, Limnol. Oceanogr., 2013, 58, 613–623 CAS.
- L. Aristilde, Y. Xu and F. M. M. Morel, Weak organic ligands enhance zinc uptake in marine phytoplankton, Environ. Sci. Technol., 2012, 46, 5438–5445 CrossRef CAS PubMed.
- F. M. M. Morel, A. B. Kustka and Y. Shaked, The role of unchelated Fe in the iron nutrition of phytoplankton, Limnol. Oceanogr., 2008, 53, 400–404 CrossRef CAS.
- Y. Xu, D. Shi, L. Aristilde and F. M. M. Morel, The effect of pH on the uptake of zinc and cadmium in marine phytoplankton: Possible role of weak complexes, Limnol. Oceanogr., 2012, 57, 293–304 CAS.
- M. I. Heller and P. L. Croot, Superoxide decay as a probe for speciation changes during dust dissolution in Tropical Atlantic surface waters near Cape Verde, Mar. Chem., 2011, 126, 37–55 CrossRef CAS PubMed.
- H. J. Venables, A. Clarke and M. P. Meredith, Wintertime controls on summer stratification and productivity at the western Antarctic Peninsula, Limnol. Oceanogr., 2013, 58, 1035–1047 CrossRef.
- C. Schlosser, C. L. De La Rocha, P. Streu and P. L. Croot, Solubility of iron in the southern ocean, Limnol. Oceanogr., 2012, 57, 684–697 CAS.
- P. Borer, B. Sulzberger, S. J. Hug, S. M. Kraemer and R. Kretzschmar, Photoreductive Dissolution of Iron(III) (Hydr)oxides in the Absence and Presence of Organic ligands: Experimental Studies and Kinetic Modeling, Environ. Sci. Technol., 2009, 43, 1864–1870 CrossRef CAS.
- K. Kim, W. Choi, M. R. Hoffmann, H.-I. Yoon and B.-K. Park, Photoreductive dissolution of iron oxides trapped in ice and its environmental implications, Environ. Sci. Technol., 2010, 44, 4142–4148 CrossRef CAS PubMed.
- D. Shi, Y. Xu, B. M. Hopkinson and F. M. M. Morel, Effect of ocean acidification on iron availability to marine phytoplankton, Science, 2010, 327, 676–679 CrossRef CAS PubMed.
- D. Shi, S. A. Kranz, J.-M. Kim and F. M. M. Morel, Ocean acidification slows nitrogen fixation and growth in the dominant diazotroph Trichodesmium under low-iron conditions, Proc. Natl. Acad. Sci. U. S. A., 2012, 109, E3094–E3100 CrossRef CAS PubMed.
- E. J. Dlugokencky, E. G. Nisbet, R. Fisher and D. Lowry, Global atmospheric methane: Budget, changes and dangers, Philos. Trans. R. Soc. London, Ser. A, 2011, 369, 2058–2072 CrossRef CAS PubMed.
- C. D. Holmes, M. J. Prather, O. A. Sovde and G. Myhre, Future methane, hydroxyl, and their uncertainties: key climate and emission parameters for future predictions, Atmos. Chem. Phys., 2013, 13, 285–302 Search PubMed.
- V. Naik, A. Voulgarakis, A. M. Fiore, L. W. Horowitz, J. F. Lamarque, M. Lin, M. J. Prather, P. J. Young, D. Bergmann, P. J. Cameron-Smith, I. Cionni, W. J. Collins, S. B. Dalsoren, R. Doherty, V. Eyring, G. Faluvegi, G. A. Folberth, B. Josse, Y. H. Lee, I. A. MacKenzie, T. Nagashima, T. P. C. van Noije, D. A. Plummer, M. Righi, S. T. Rumbold, R. Skeie, D. T. Shindell, D. S. Stevenson, S. Strode, K. Sudo, S. Szopa and G. Zeng, Preindustrial to present-day changes in tropospheric hydroxyl radical and methane lifetime from the Atmospheric Chemistry and Climate Model Intercomparison Project (ACCMIP), Atmos. Chem. Phys., 2013, 13, 5277–5298 Search PubMed.
- S. A. Montzka, E. J. Dlugokencky and J. H. Butler, Non-CO2 greenhouse gases and climate change, Nature, 2011, 476, 43–50 CrossRef CAS PubMed.
- S. Houweling, M. Krol, P. Bergamaschi, C. Frankenberg, E. J. Dlugokencky, I. Morino, J. Notholt, V. Sherlock, D. Wunch, V. Beck, C. Gerbig, H. Chen, E. A. Kort, T. Röckmann and I. Aben, A multi-year methane inversion using SCIAMACHY, accounting for systematic errors using TCCON measurements, Atmos. Chem. Phys. Discuss., 2013, 13, 28117–28171 CrossRef.
- A. N. Ross, M. J. Wooster, H. Boesch and R. Parker, First satellite measurements of carbon dioxide and methane emission ratios in wildfire plumes, Geophys. Res. Lett., 2013, 40, 4098–4102 CrossRef CAS.
- A. Vasileva and K. Moiseenko, Methane emissions from 2000 to 2011 wildfires in Northeast Eurasia estimated with MODIS burned area data, Atmos. Environ., 2013, 71, 115–121 CrossRef CAS PubMed.
- J. Worden, Z. Jiang, D. B. A. Jones, M. Alvarado, K. Bowman, C. Frankenberg, E. A. Kort, S. S. Kulawik, M. Lee, J. Liu, V. Payne, K. Wecht and H. Worden, El Niño, the 2006 Indonesian peat fires, and the distribution of atmospheric methane, Geophys. Res. Lett., 2013, 40, 4938–4943 CAS.
- Y. Zhang, D. Chu, Y. Li, L. Wang and Y. Wu, Effect of elevated UV-B radiation on CH4 emissions from the stands of Spartina alterniflora and Phragmites australis in a coastal salt marsh, Aquat. Bot., 2013, 111, 150–156 CrossRef CAS PubMed.
- S. K. Morsky, J. K. Haapala, R. Rinnan, S. Saarnio, H. Suokanerva, K. Latola, E. Kyro, J. Silvola, T. Holopainen and P. J. Martikainen, Minor long-term effects of ultraviolet-B radiation on methane dynamics of a subarctic fen in Northern Finland, Biogeochemistry, 2012, 108, 233–243 CrossRef.
- E. A. Kort, S. C. Wofsy, B. C. Daube, M. Diao, J. W. Elkins, R. S. Gao, E. J. Hintsa, D. F. Hurst, R. Jimenez, F. L. Moore, J. R. Spackman and M. A. Zondlo, Atmospheric observations of Arctic Ocean methane emissions up to 82o north, Nat. Geosci., 2012, 5, 318–321 CrossRef CAS.
- N. Shakhova, I. Semiletov, A. Salyuk, V. Yusupov, D. Kosmach and O. Gustafsson, Extensive methane venting to the atmosphere from sediments of the East Siberian Arctic Shelf, Science, 2010, 327, 1246–1250 CrossRef CAS PubMed.
- O. S. Pokrovsky, L. S. Shirokova, S. N. Kirpotin, S. P. Kulizhsky and S. N. Vorobiev, Impact of western Siberia heat wave 2012 on greenhouse gases and trace metal concentration in thaw lakes of discontinuous permafrost zone, Biogeosciences, 2013, 10, 5349–5365 CAS.
- C. G. Fichot and W. L. Miller, An approach to quantify depth-resolved marine photochemical fluxes using remote sensing: Application to carbon monoxide (CO) photoproduction, Remote Sens. Environ., 2010, 114, 1363–1377 CrossRef PubMed.
- G. Song, H. Xie, S. Belanger, E. Leymarie and M. Babin, Spectrally resolved efficiencies of carbon monoxide (CO) photoproduction in the western Canadian Arctic: Particles versus solutes, Biogeosciences, 2013, 10, 3731–3748 Search PubMed.
- A. Stubbins, C. S. Law, G. Uher and R. C. Upstill-Goddard, Carbon monoxide apparent quantum yields and photoproduction in the Tyne estuary, Biogeosciences, 2011, 8, 703–713 CrossRef CAS.
- G. P. Yang, C. Y. Ren, X. L. Lu, C. Y. Liu and H. B. Ding, Distribution, flux, and photoproduction of carbon monoxide in the East China Sea and Yellow Sea in spring, J. Geophys. Res.: Oceans, 2011, 116 Search PubMed.
- Y. Zhang and H. X. Xie, The sources and sinks of carbon monoxide in the St. Lawrence estuarine system, Deep-Sea Res., Part II, 2012, 81–84, 114–123 CrossRef CAS PubMed.
- C. E. Schmidt and B. G. Heikes, Aqueous carbon monoxide cycling in a fjord-like estuary, Estuaries Coasts, 2014, 37, 751–762 CrossRef CAS.
- H. Xie, S. Belanger, G. Song, R. Benner, A. Taalba, M. Blais, J. E. Tremblay and M. Babin, Photoproduction of ammonium in the southeastern Beaufort Sea and its biogeochemical implications, Biogeosciences, 2012, 9, 3047–3061 CAS.
- L. Derendorp, J. B. Quist, R. Holzinger and T. Rockmann, Emissions of H2 and CO from leaf litter of Sequoiadendron giganteum, and their dependence on UV radiation and temperature, Atmos. Environ., 2011, 45, 7520–7524 CrossRef CAS PubMed.
- D. Bruhn, K. R. Albert, T. N. Mikkelsen and P. Ambus, UV-induced carbon monoxide emission from living vegetation, Biogeosciences, 2013, 10, 7877–7882 CrossRef CAS.
- S. Madronich, M. Shao, S. R. Wilson, K. R. Solomon, J. Longstrethe and X. Tang, Changes in air quality and tropospheric composition due to depletion of stratospheric ozone and interactions with changing climate: Implications for human and environmental health, Photochem. Photobiol. Sci., 2015, 14 10.1039/c4pp90037e , this issue.
- M. J. Prather and J. Hsu, Coupling of nitrous oxide and methane by global atmospheric chemistry, Science, 2010, 330, 952–954 CrossRef CAS PubMed.
- K. Pilegaard, Processes regulating nitric oxide emissions from soils, Philos. Trans. R. Soc. London, B, 2013, 368 Search PubMed.
- R. Oswald, T. Behrendt, M. Ermel, D. Wu, H. Su, Y. Cheng, C. Breuninger, A. Moravek, E. Mougin, C. Delon, B. Loubet, A. Pommerening-Roeser, M. Soergel, U. Poeschl, T. Hoffmann, M. O. Andreae, F. X. Meixner and I. Trebs, HONO emissions from soil bacteria as a major source of atmospheric reactive nitrogen, Science, 2013, 341, 1233–1235 CrossRef CAS PubMed.
- H. Su, Y. Cheng, R. Oswald, T. Behrendt, I. Trebs, F. X. Meixner, M. O. Andreae, P. Cheng, Y. Zhang and U. Poeschl, Soil nitrite as a source of atmospheric HONO and OH radicals, Science, 2011, 333, 1616–1618 CrossRef CAS PubMed.
- S. Morin, J. Erbland, J. Savarino, F. Domine, J. Bock, U. Friess, H. W. Jacobi, H. Sihler and J. M. F. Martins, An isotopic view on the connection between photolytic emissions of NOx from the Arctic snowpack and its oxidation by reactive halogens, J. Geophys. Res., [Atmos.], 2012, 117 Search PubMed.
- N. K. Richards and B. J. Finlayson-Pitts, Production of gas phase NO2 and halogens from the photochemical oxidation of aqueous mixtures of sea salt and nitrate ions at room temperature, Environ. Sci. Technol., 2012, 46, 10447–10454 CrossRef CAS PubMed.
- J. R. Brown, J. C. Blankinship, A. Niboyet, K. J. van Groenigen, P. Dijkstra, X. Le Roux, P. W. Leadley and B. A. Hungate, Effects of multiple global change treatments on soil N2O fluxes, Biogeochemistry, 2012, 109, 85–100 CrossRef CAS PubMed.
- K. J. van Groenigen, C. W. Osenberg and B. A. Hungate, Increased soil emissions of potent greenhouse gases under increased atmospheric CO2, Nature, 2011, 475, 214–U121 CrossRef CAS PubMed.
- A. E. Santoro, C. Buchwald, M. R. McIlvin and K. L. Casciotti, Isotopic signature of N2O produced by marine ammonia-oxidizing Archaea, Science, 2011, 333, 1282–1285 CrossRef CAS PubMed.
- L. A. Codispoti, Interesting times for marine N2O, Science, 2010, 327, 1339–1340 CrossRef CAS PubMed.
- J. T. Walker, C. A. Stow and C. Geron, Nitrous oxide emissions from the Gulf of Mexico hypoxic zone, Environ. Sci. Technol., 2010, 44, 1617–1623 CrossRef CAS PubMed.
- M. Voss, H. W. Bange, J. W. Dippner, J. J. Middelburg, J. P. Montoya and B. Ward, The marine nitrogen cycle: recent discoveries, uncertainties and the potential relevance of climate change, Philos. Trans. R. Soc. London, B, 2013, 368 Search PubMed.
- V. Kitidis, R. C. Upstill-Goddard and L. G. Anderson, Methane and nitrous oxide in surface water along the North-West Passage, Arctic Ocean, Mar. Chem., 2010, 121, 80–86 CrossRef CAS PubMed.
- K. Randall, M. Scarratt, M. Levasseur, S. Michaud, H. Xie and M. Gosselin, First measurements of nitrous oxide in Arctic sea ice, J. Geophys. Res.: Oceans, 2012, 117 Search PubMed.
- X. Jin and N. Gruber, Offsetting the radiative benefit of ocean iron fertilization by enhancing N2O emissions, Geophys. Res. Lett., 2003, 30 Search PubMed.
- E. Blei and M. R. Heal, Methyl bromide and methyl chloride fluxes from temperate forest litter, Atmos. Environ., 2011, 45, 1543–1547 CrossRef CAS PubMed.
- T. Fujimori, Y. Yoneyama, G. Taniai, M. Kurihara, H. Tamegai and S. Hashimoto, Methyl halide production by cultures of marine proteobacteria Erythrobacter and Pseudomonas and isolated bacteria from brackish water, Limnol. Oceanogr., 2012, 57, 154–162 CrossRef CAS.
- C. J. Hardacre and M. R. Heal, Characterization of methyl bromide and methyl chloride fluxes at temperate freshwater wetlands, J. Geophys. Res.: Atmos., 2013, 118, 977–991 CAS.
- M. K. Kurihara, M. Kimura, Y. Iwamoto, Y. Narita, A. Ooki, Y. J. Eum, A. Tsuda, K. Suzuki, Y. Tani, Y. Yokouchi, M. Uematsu and S. Hashimoto, Distributions of short-lived iodocarbons and biogenic trace gases in the open ocean and atmosphere in the western North Pacific, Mar. Chem., 2010, 118, 156–170 CrossRef CAS PubMed.
- C. Y. Lin and S. L. Manley, Bromoform production from seawater treated with bromoperoxidase, Limnol. Oceanogr., 2012, 57, 1857–1866 CrossRef CAS.
- R. Hossaini, M. P. Chipperfield, W. Feng, T. J. Breider, E. Atlas, S. A. Montzka, B. R. Miller, F. Moore and J. Elkins, The contribution of natural and anthropogenic very short-lived species to stratospheric bromine, Atmos. Chem. Phys., 2012, 12, 371–380 CrossRef CAS.
- A. Saiz-Lopez and R. von Glasow, Reactive halogen chemistry in the troposphere, Chem. Soc. Rev., 2012, 41, 6448–6472 RSC.
- A. Saiz-Lopez, J. F. Lamarque, D. E. Kinnison, S. Tilmes, C. Ordonez, J. J. Orlando, A. J. Conley, J. M. C. Plane, A. S. Mahajan, G. S. Santos, E. L. Atlas, D. R. Blake, S. P. Sander, S. Schauffler, A. M. Thompson and G. Brasseur, Estimating the climate significance of halogen-driven ozone loss in the tropical marine troposphere, Atmos. Chem. Phys., 2012, 12, 3939–3949 CrossRef CAS.
- A. Qureshi, N. J. O'Driscoll, M. MacLeod, Y.-M. Neuhold and K. Hungerbuehler, Photoreactions of mercury in surface ocean water: gross reaction kinetics and possible pathways, Environ. Sci. Technol., 2010, 44, 644–649 CrossRef CAS PubMed.
- R. Hossaini, H. Mantle, M. P. Chipperfield, S. A. Montzka, P. Hamer, E. Ziska, B. Quack, K. Krueger, S. Tegtmeier, E. Atlas, S. Sala, A. Engel, H. Boenisch, T. Keber, D. Oram, G. Mills, C. Ordonez, A. Saiz-Lopez, N. Warwick, Q. Liang, W. Feng, E. Moore, B. R. Miller, V. Marecal, N. A. D. Richards, M. Dorf and K. Pfeilsticker, Evaluating global emission inventories of biogenic bromocarbons, Atmos. Chem. Phys., 2013, 13, 11819–11838 CrossRef CAS.
- R. Hossaini, M. P. Chipperfield, S. Dhomse, C. Ordonez, A. Saiz-Lopez, N. L. Abraham, A. Archibald, P. Braesicke, P. Telford, N. Warwick, X. Yang and J. Pyle, Modelling future changes to the stratospheric source gas injection of biogenic bromocarbons, Geophys. Res. Lett., 2012, 39 Search PubMed.
-
N. M. Mahowald, Atmospheric Biogeochemistry, in Encyclopedia of Sustainability Science and Technology, Springer, 2012 Search PubMed.
- F. Lambert, J. S. Kug, R. J. Park, N. Mahowald, G. Winckler, A. Abe-Ouchi, R. O'Ishi, T. Takemura and J. H. Lee, The role of mineral-dust aerosols in polar temperature amplification, Nat. Clim. Change, 2013, 3, 487–491 CrossRef.
- Y. Ming, V. Ramaswamy and G. Chen, A model investigation of aerosol-induced changes in boreal winter extratropical circulation, J. Clim., 2011, 24, 6077–6091 CrossRef.
- J. M. Creamean, K. J. Suski, D. Rosenfeld, A. Cazorla, P. J. DeMott, R. C. Sullivan, A. B. White, F. M. Ralph, P. Minnis, J. M. Comstock, J. M. Tomlinson and K. A. Prather, Dust and biological aerosols from the Sahara and Asia influence precipitation in the Western U.S., Science, 2013, 339, 1572–1578 CrossRef CAS PubMed.
- M. Schulz, J. M. Prospero, A. R. Baker, F. Dentener, L. Ickes, P. S. Liss, N. M. Mahowald, S. Nickovic, C. P. Garcia-Pando, S. Rodriguez, M. Sarin, I. Tegen and R. A. Duce, Atmospheric transport and deposition of mineral dust to the ocean: Implications for research needs, Environ. Sci. Technol., 2012, 46, 10390–10404 CrossRef CAS PubMed.
- J. Mao, S. Fan, D. J. Jacob and K. R. Travis, Radical loss in the atmosphere from Cu-Fe redox coupling in aerosols, Atmos. Chem. Phys., 2013, 13, 509–519 CAS.
- M. Kulmala, T. Nieminen, R. Chellapermal, R. Makkonen, J. Bäck and K. Veli-Matti, Climate feedbacks linking the increasing atmospheric CO2 concentration, BVOC emissions, aerosols and clouds in forest ecosystems, Tree Physiol., 2013, 5, 489–508 CrossRef.
- S.-C. Hsu, C.-A. Huh, C.-Y. Lin, W.-N. Chen, N. M. Mahowald, S.-C. Liu, C. C. K. Chou, M.-C. Liang, C.-J. Tsai, F.-J. Lin, J.-P. Chen and Y.-T. Huang, Dust transport from non-East Asian sources to the North Pacific, Geophys. Res. Lett., 2012, 39 Search PubMed.
- M. Kanakidou, R. A. Duce, J. M. Prospero, A. R. Baker, C. Benitez-Nelson, F. J. Dentener, K. A. Hunter, P. S. Liss, N. Mahowald, G. S. Okin, M. Sarin, K. Tsigaridis, M. Uematsu, L. M. Zamora and T. Zhu, Atmospheric fluxes of organic N and P to the global ocean, Global Biogeochem. Cycles, 2012, 26 Search PubMed.
- D. Rothenber, N. M. Mahowald, K. Lindsay, D. Scott, K. Moore and P. Thornton, Volcano impacts on climate and biogeochemistry in a coupled carbon-climate model, Earth Syst. Dyn., 2012, 3, 121–136 CrossRef PubMed.
- H. Liang, Z. M. Chen, D. Huang, Y. Zhao and Z. Y. Li, Impacts of aerosols on the chemistry of atmospheric trace gases: a case study of peroxides and HO2 radicals, Atmos. Chem. Phys. Discuss., 2013, 13, 16549–16595 CrossRef.
- T. Gouin, J. M. Armitage, I. T. Cousins, D. C. G. Muir, C. A. Ng, L. Reid and S. Tao, Influence of global climate change on chemical fate and bioaccumulation: The role of multimedia models, Environ. Toxicol. Chem., 2013, 32, 20–31 CrossRef CAS PubMed.
- M. J. Hooper, G. T. Ankley, D. A. Cristol, L. A. Maryoung, P. D. Noyes and K. E. Pinkerton, Interactions between chemical and climate stressors: A role for mechanistic toxicology in assessing climate change risks, Environ. Toxicol. Chem., 2013, 32, 32–48 CrossRef CAS PubMed.
- J. Armitage, C. Quinn and F. Wania, Global climate change and contaminants—an overview of opportunities and priorities for modelling the potential implications for long-term human exposure to organic compounds in the Arctic, J. Environ. Monit., 2011, 13, 1352–1546 RSC.
- A. M. Grannas, C. Bogdal, K. J. Hageman, C. Halsall, T. Harner, H. Hung, R. Kallenborn, P. Klan, J. Klanova, R. W. Macdonald, T. Meyer and F. Wania, The role of the global cryosphere in the fate of organic contaminants, Atmos. Chem. Phys., 2013, 13, 3271–3305 CrossRef.
-
UNEP, Climate change and POPs: Predicting the impacts. Report of the UNEP/AMAP Expert Group, (Ed.: Secretariat of the Stockholm Convention), United Nations Environment Programme/Arctic Monitoring and Assessment program, Geneva, Switzerland, 2011.
- L. Carlos, D. O. Martire, M. C. Gonzalez, J. Gomis, A. Bernabeu, A. M. Amat and A. Arques, Photochemical fate of a mixture of emerging pollutants in the presence of humic substances, Water Res., 2012, 46, 4732–4740 CrossRef CAS PubMed.
- Y. Chen, H. Li, Z. P. Wang, H. J. Li, T. Tao and Y. G. Zuo, Photodegradation of selected beta-blockers in aqueous fulvic acid solutions: Kinetics, mechanism, and product analysis, Water Res., 2012, 46, 2965–2972 CrossRef CAS PubMed.
- J. J. Guerard and Y. P. Chin, Photodegradation of ormetoprim in aquaculture and stream-derived dissolved organic matter, J. Agric. Food Chem., 2012, 60, 9801–9806 CrossRef CAS PubMed.
- G. A. Rowland, A. R. Bausch and A. M. Grannas, Photochemical processing of aldrin and dieldrin in frozen aqueous solutions under arctic field conditions, Environ. Pollut., 2011, 159, 1076–1084 CrossRef CAS PubMed.
- M. B. Fisher, D. C. Love, R. Schuech and K. L. Nelson, Simulated sunlight action spectra for inactivation of MS2 and PRD1 bacteriophages in clear water, Environ. Sci. Technol., 2011, 45, 9249–9255 CrossRef CAS PubMed.
- K. G. McGuigan, R. M. Conroy, H. J. Mosler, M. du Preez, E. Ubomba-Jaswa and P. Fernandez-Ibanez, Solar water disinfection (SODIS): A review from bench-top to roof-top, J. Hazard. Mater., 2012, 235, 29–46 CrossRef PubMed.
- A. I. Silverman, B. M. Peterson, A. B. Boehm, K. McNeill and K. L. Nelson, Sunlight inactivation of human viruses and bacteriophages in coastal waters containing natural photosensitizers, Environ. Sci. Technol., 2013, 47, 1870–1878 CrossRef CAS PubMed.
- E. S. Corbitt, D. J. Jacob, C. D. Holmes, D. G. Streets and E. M. Sunderland, Global source-receptor relationships for mercury deposition under present-day and 2050 emissions scenarios, Environ. Sci. Technol., 2011, 45, 10477–10484 CrossRef CAS PubMed.
- D. P. Krabbenhoft and E. M. Sunderland, Global change and mercury, Science, 2013, 341, 1457–1458 CrossRef CAS PubMed.
- A. L. Andrady, A. Torikai, H. H. Redhwi, K. K. Pandey and P. Gies, Consequences of stratospheric ozone depletion and climate change on the use of materials, Photochem. Photobiol. Sci., 2015, 14 10.1039/c4pp90038c , this issue.
- N. F. Adegboyega, V. K. Sharma, K. Siskova, R. Zboril, M. Sohn, B. J. Schultz and S. Banerjee, Interactions of Aqueous Ag+ with Fulvic Acids: Mechanisms of Silver Nanoparticle Formation and Investigation of Stability, Environ. Sci. Technol., 2013, 47, 757–764 CrossRef CAS PubMed.
- W. C. Hou, B. Stuart, R. Howes and R. G. Zepp, Sunlight-driven reduction of silver ions by natural organic matter: formation and transformation of silver nanoparticles, Environ. Sci. Technol., 2013, 47, 7713–7721 CrossRef CAS PubMed.
- C. Kingston, R. Zepp, A. Andrady, D. Boverhof, R. Fehir, D. Hawkins, J. Roberts, P. Sayre, B. Shelton, Y. Sultan, V. Vejins and W. Wohlleben, Release characteristics of selected carbon nanotube polymer composites, Carbon, 2014, 68, 33–57 CrossRef CAS PubMed.
- A. P. Ballantyne, C. B. Alden, J. B. Miller, P. P. Tans and J. W. C. White, Increase in observed net carbon dioxide uptake by land and oceans during the past 50 years, Nature, 2012, 488, 70 CrossRef CAS PubMed.
- J. G. Canadell, C. Le Quere, M. R. Raupach, C. B. Field, E. T. Buitenhuis, P. Ciais, T. J. Conway, N. P. Gillett, R. A. Houghton and G. Marland, Contributions to accelerating atmospheric CO2 growth from economic activity, carbon intensity, and efficiency of natural sinks, Proc. Natl. Acad. Sci. U. S. A., 2007, 104, 18866–18870 CrossRef CAS PubMed.
- C. Le Quere, T. Takahashi, E. T. Buitenhuis, C. Roedenbeck and S. C. Sutherland, Impact of climate change and variability on the global oceanic sink of CO2, Global Biogeochem. Cycles, 2010, 24 Search PubMed.
- G. A. McKinley, A. R. Fay, T. Takahashi and N. Metzl, Convergence of atmospheric and North Atlantic carbon dioxide trends on multidecadal timescales, Nat. Geosci., 2011, 4, 606–610 CrossRef CAS.
- W. Knorr, Is the airborne fraction of anthropogenic CO2 emissions increasing?, Geophys. Res. Lett., 2009, 36 Search PubMed.
-
C. L. Sabine and T. Tanhua, Estimation of anthropogenic CO2 inventories in the ocean, in Annual Review of Marine Science, vol. 2, 2010, pp. 175–198 Search PubMed.
- I. Fountoulakis, A. F. Bais, K. Tourpali, K. Fragkos and S. Misios, Projected changes in solar UV radiation in the Arctic and sub-Arctic Oceans: Effects from changes in reflectivity, ice transmittance, clouds, and ozone, J. Geophys. Res., [Atmos.], 2014, 119, 8073–8090 Search PubMed.
- C. E. Williamson, R. G. Zepp, R. M. Lucas, S. Madronich, A. T. Austin, C. L. Ballaré, M. Norval, B. Sulzberger, A. F. Bais, R. L. McKenzie, S. A. Robinson, D. P. Hader, N. D. Paul and J. F. Bornman, Solar UV radiation in a changing climate, Nat. Clim. Change, 2014, 4, 434–441 CrossRef.
- R. A. Houghton, Balancing the global carbon budget, Annu. Rev. Earth Planet. Sci., 2007, 35, 313–347 CrossRef CAS.
|
This journal is © The Royal Society of Chemistry and Owner Societies 2015 |