Ozone depletion and climate change: impacts on UV radiation
Received
20th October 2014
, Accepted 20th October 2014
First published on 7th November 2014
Abstract
We assess the importance of factors that determine the intensity of UV radiation at the Earth's surface. Among these, atmospheric ozone, which absorbs UV radiation, is of considerable importance, but other constituents of the atmosphere, as well as certain consequences of climate change, can also be major influences. Further, we assess the variations of UV radiation observed in the past and present, and provide projections for the future. Of particular interest are methods to measure or estimate UV radiation at the Earth's surface. These are needed for scientific understanding and, when they are sufficiently sensitive, they can serve as monitors of the effectiveness of the Montreal Protocol and its amendments. Also assessed are several aspects of UV radiation related to biological effects and health. The implications for ozone and UV radiation from two types of geoengineering methods that have been proposed to combat climate change are also discussed. In addition to ozone effects, the UV changes in the last two decades, derived from measurements, have been influenced by changes in aerosols, clouds, surface reflectivity, and, possibly, by solar activity. The positive trends of UV radiation observed after the mid-1990s over northern mid-latitudes are mainly due to decreases in clouds and aerosols. Despite some indications from measurements at a few stations, no statistically significant decreases in UV-B radiation attributable to the beginning of the ozone recovery have yet been detected. Projections for erythemal irradiance (UVery) suggest the following changes by the end of the 21st century (2090–2100) relative to the present time (2010–2020): (1) Ozone recovery (due to decreasing ozone-depleting substances and increasing greenhouse gases) would cause decreases in UVery, which will be highest (up to 40%) over Antarctica. Decreases would be small (less than 10%) outside the southern Polar Regions. A possible decline of solar activity during the 21st century might affect UV-B radiation at the surface indirectly through changes induced in stratospheric ozone. (2) The projected changes in cloud cover would lead to relatively small effects (less than 3%), except at northern high latitudes where increases in cloud cover could lead to decreases in UVery by up to 7%. (3) Reductions in reflectivity due to the melting of sea-ice in the Arctic would lead to decreases of UVery by up to 10%, while at the margins of the Antarctic the decreases would be smaller (2–3%). The melting of the sea-ice would expose the ocean surface formerly covered by ice to UV-B radiation up to 10 times stronger than before. (4) The expected improvement of air-quality and reductions of aerosols over the most populated areas of the northern hemisphere may result in 10–20% increases in UVery, except over China where even larger increases are projected. The projected aerosol effect for the southern hemisphere is generally very small. Aerosols are possibly the most important factor for future UV levels over heavily populated areas, but their projected effects are the most uncertain.
Introduction
For the purposes of the current assessment (2010–2014), which addresses the negative and positive effects of solar UV radiation on humans, terrestrial and aquatic ecosystems, materials, and air quality (see companion papers), we assess the short- and long-term changes in ambient UV radiation at the Earth's surface resulting from changes in atmospheric ozone and climate. The effects of ozone on climate and climate on ozone are also discussed. Absorption by ozone is the dominant factor controlling the levels of surface UV-B (280–315 nm) radiation for cloud-free and low-aerosol conditions. With the continuing success of the amended and adjusted Montreal Protocol in reducing the concentrations of ozone depleting substances (ODSs), the focus is now on the detection of possible decreases in UV-B radiation in response to the first signs of recovery of the ozone layer. Changes in the climate caused by the increasing concentrations of greenhouse gases may also affect the UV radiation at the Earth's surface indirectly, as detailed below.
Current status of atmospheric ozone
Since the last assessment of ozone depletion1 efforts to quantify the geographic and temporal variability of ozone have continued through ground- and satellite-based measurements. This extension of the observation of ozone by four years has increased the statistical confidence in the estimated long-term changes in total ozone column (TOC). There are indications that the global ozone layer is beginning to recover from the depletion caused by ODSs. However, the variability of the atmosphere, the uncertainty of measurements, and the influence of climate change prevent unequivocal attribution of the observed increases in ozone since 2000 to decreases in ODSs.2,3
Ozone at mid-latitudes and the tropics
The present (2008–2012) mean values of ozone relative to the 1964–1980 mean values are smaller by ∼3.5% in the Northern Hemisphere mid-latitudes (35° N–60° N) and by ∼6% in the southern hemisphere mid-latitudes (35° S–60° S). In the tropics (20° S–20° N), no significant changes have occurred in total ozone over this period. The observed average changes in total ozone over time, relative to the 1998–2008 mean values, in different latitude bands are shown in Fig. 1.
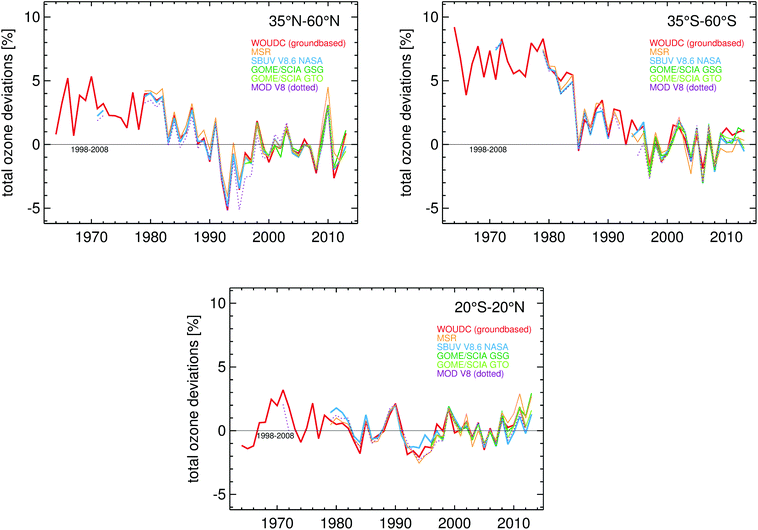 |
| Fig. 1 Total column ozone annual mean deviations relative to the 1998–2008 mean for different latitude bands as derived from different ground- and space-based datasets. Adapted from WMO 2015.2 | |
Following the decline in total ozone between the 1960s and 1990s, the levels of total ozone outside the polar regions have stopped decreasing since the late 1990s, consistent with the slow decline of ODSs over the same period.4 Several datasets indicate that total ozone has increased by ∼1% since 2000 in the latitude band 60° S–60° N in response to stratospheric ozone recovery. However, there is disagreement about the magnitude and statistical significance of this increase.2 Presumably any increase in ozone would have resulted in a corresponding decrease in the surface UV-B radiation at the Earth's surface, by analogy with the increases in UV-B radiation observed for the ozone decline.5
The amounts of total ozone are subject to large year-to-year variability caused by variations in atmospheric circulation. Examples include the unusually high values in 2010 and low values in 2011 in the northern hemisphere mid and high latitudes. Because of these large variations, the relatively small increases in total ozone expected after the recent decline of ODSs are still not statistically significant.6,7 Without the removal of these circulation effects, the attribution of ozone recovery to decreases in ODSs would not be detectable even in Antarctica before the period of 2017–2021.8,9 The separation of recent changes of ozone into the contributions by ODSs, greenhouse gases (GHG), and natural low-frequency variability remains challenging. For example, after removing the variations caused by the solar variability from satellite data for total ozone, the residuals averaged over the band 60° S–60° N show a decline of about 5% between 1980 and 2000, followed by a partial recovery after about 2000.10 However, significant sporadic reductions in total ozone have been observed in geographically localised areas at high latitudes in South America11 and in northern high- and mid-latitudes,12 showing that the ozone depletion problem is not yet fully solved.
Analysis of the variability of extreme values in the longest time series of total ozone (Arosa, Switzerland, starting in the 1920s) has revealed an increasing frequency of both low-ozone and high-ozone events, which dominate trends in the 1970s and 1980s. After the removal of the extreme events from the original time series, the overall downward trend in the period 1970–1990 is reduced from 2.4% per decade to 0.9% per decade.13,14 However, since the extremes are relative to the long-term climatology, the values that were removed also include small values that have been caused by the increase in ODSs during that period. A similar study for Sonnblick, Austria, over a shorter period (1994–2011), revealed a decline in the frequency of low-ozone events and an increase in high-ozone events.15
In the northern hemisphere, the increase in tropospheric ozone from precursors (CO, NOx, and hydrocarbons) since pre-industrial times nearly equals the decrease in stratospheric ozone from increases in ODSs. In the southern hemisphere, the decrease in stratospheric ozone dominates the total ozone column. Currently, the impact of increasing N2O, a source gas that leads to the formation of reactive nitrogen in the stratosphere, on ozone almost cancels the globally averaged increases from climate change effects16 (see discussion below).
Our present understanding is that the present levels of global total ozone are still less than the mean levels in the period 1960–1980. Over most latitudes, total ozone values have stabilized after the mid-1990s, but the year-to-year variability has increased relative to the period before the mid-1990s, precluding the unequivocal detection of possible increases expected from the observed decline of ODSs concentrations.
Ozone at high latitudes.
Over the high latitudes (63°–90°) of both hemispheres, ozone depletion continues to occur during winter and spring. Compared to the average values before 1980, the 2010–2013 mean total ozone is lower by ∼27% in the southern hemisphere in October and by ∼10% in the northern hemisphere in March.2
The Antarctic ozone hole has continued to appear each spring. The evolution of total ozone in Antarctica over the last decade has been significantly affected by variations in stratospheric temperature and circulation, which have masked the effect of the decreases in ODSs since the early 2000s. With an accurate account of circulation-induced changes, a small (3–8%) increase in total ozone over Antarctica during the last decade is now apparent.17–19 Even without accounting for these circulation effects, reductions in the severity of ozone depletion in Antarctica since the 1990s are now becoming clear.2 However, uncertainties in methods and measurements preclude a definite conclusion that the recent increases of ozone in Antarctica are due to declining concentrations of ODSs alone.2
Any reversal of total ozone trends is not yet apparent in the Arctic spring, where the largest ever ozone loss was observed in 2011.20,21 The concentration of ozone in the Arctic stratosphere during the spring of 2011 was the lowest since satellite records began in 1979. The minimum daily average column ozone (297 Dobson Units (DU)) was observed in March 2011.22 This value is 18 DU below the previous record-low observed in March 2000, and 100 DU (25%) below the average for 1979–1988. At some locations and times, the amounts of total ozone observed between February and April 2011 were more than 50% below the climatological mean.23 The fraction of the Arctic vortex area with total ozone below 275 DU is typically near zero for March, but reached nearly 45% in March 2011.12 In that year, the minimum total ozone in spring was continuously below 250 DU for about 27 days, and values between 220 and 230 DU were observed for about one week in late March,12 leading to increases in UV-B radiation, as discussed later.
This large chemically-mediated loss of ozone in the Arctic was the result of an unusually prolonged cold period in the lower stratosphere and an anomalously strong Arctic vortex, which weakened the transport of ozone from middle latitudes24 and facilitated the formation of polar stratospheric clouds (PSCs). These clouds provide surfaces for the heterogeneous reactions that activate stratospheric chlorine, which in turn destroys ozone in catalytic cycles. Temperatures below the threshold for the formation of PSCs of about −77° C occurred between December 2010 and early April 2011. Over 80% of the ozone present in January from about 18 to 20 km altitude had been chemically destroyed by late March, which is roughly twice that in the previous record-setting winters of 1996 and 2005.12 The anomalies for 2011 due to chemistry and transport stand out as extreme events, greater than 2σ (standard deviation), while the total anomaly was nearly 3σ.24 The amount of ozone loss and the chemistry of the Arctic stratosphere in the spring of 2011 was remarkably similar to that commonly observed in Antarctica, justifying the conclusion that there was an Arctic ozone hole in 2011.25
There are indications that this Arctic ozone depletion event contributed to the smaller total ozone values recorded at mid-latitude locations. Measurements at 34 European stations revealed that the total ozone over Western Europe from late March to late April 2011 was 15%–25% less than the mean value for this period over the last decade.26
There is no indication that the extreme meteorological conditions that led to the loss of ozone in the Arctic in 2011 were driven by climate change.21 Severe ozone depletion, such as occurred in 2011, or even worse, could possibly happen over the next decades under similar conditions of long-lasting cold stratospheric temperatures.27 The effect of these large reductions of ozone on surface UV-B radiation is discussed later.
Effects of depletion and recovery of ozone on climate
Changes in stratospheric ozone influence the climate both directly through radiative effects and indirectly by affecting stratospheric and tropospheric circulation.2 Ozone depletion was the dominant driver of the globally averaged cooling that occurred in the lower stratosphere during the last part of the 20th century,28 but no statistically significant temperature change has occurred there since the mid-1990s.29,30 Episodic warming over this period has occurred due to aerosols after major volcanic eruptions.30 Cooling of the stratosphere due to ozone depletion over Antarctica is, in turn, the dominant driver of circulation changes in the southern hemisphere troposphere during summer (see also ref. 31 and 32). According to model simulations, these changes have led to changes in surface wind patterns, pole-ward shifting of the midlatitude maximum of precipitation33 and increases of moisture in the subtropics.33–38 Opposite effects for the southern hemisphere circulation and climate would be expected for the future from the projected recovery of stratospheric ozone. However, increases in GHGs would compensate partly for these ozone recovery-induced effects on climate.39–44 For a more detailed discussion on the effects of ozone depletion and recovery on climate see the WMO Scientific Assessment of Ozone Depletion: 2014.2
Indirect effects of climate change on surface UV radiation
Climate change may have indirectly influenced the levels of UV radiation in the past by altering the amounts of ozone, UV-absorbing tropospheric gases, aerosols, and clouds in the atmosphere. These influences will likely continue into the future.2,45 Future changes in the reflectivity of the Earth's surface, either due to the melting of sea-ice and ice-caps at high latitudes46 or due to reduced snow-cover, may also be important. Cooling of the stratosphere resulting from increased concentrations of CO2 and other GHGs will lead to greater concentrations of ozone in the future because the destruction rates of ozone in the cooler middle and upper stratosphere, outside the Polar Regions, will decrease. However, at high latitudes, where temperatures in the lower stratosphere may drop below the threshold for the formation of PSCs, heterogeneous chemistry on the surfaces of these clouds in the presence of chlorine can potentially lead to a rapid loss of ozone. An example of these processes is the annually recurring springtime Antarctic ozone-hole. For the Arctic, chemistry-climate models (CCM) suggest that while in the near future there is a chance of low springtime ozone in individual years, there is no indication of a formation of regular Arctic ozone holes.47 Although a much wider area may be susceptible to heterogeneous processes later this century, the projected smaller concentrations of chlorine by that time are expected to moderate the potential for loss of ozone.
Increasing concentrations of GHGs will increase the strength of the primary large-scale transport and overturning of the upper atmosphere (the Brewer–Dobson circulation), leading to decreases of ozone in the tropics and increases outside the tropics. Emissions of CH4 and N2O would also affect the evolution of global stratospheric ozone, particularly in the second half of the 21st century, when concentrations of ODSs are expected to be small.2 The increases of ozone outside the tropics caused by rising concentrations of GHGs will be partly offset by additional chemical destruction arising from anthropogenic emissions of N2O.48
Clouds respond to climate-forcing mechanisms in multiple ways, and the feedback of clouds can be positive or negative. Climate change is projected to reduce the amount of clouds in the future over most of the tropics and mid-latitudes, with mostly reductions in the amount of low clouds.45 Changes in clouds in the marine boundary layer are most uncertain. Over higher latitudes (>50°), increases in the fraction of cloud cover and optical depth are projected. This would increase the amount of solar UV radiation scattered back to space and, therefore, reduce the UV radiation reaching Earth's surface. Furthermore, clouds play a critical role in the climate system, since they can increase the planetary albedo, thereby counteracting global warming, but they can also contribute to warming of the troposphere through absorption of infrared radiation emitted from the surface.
Reductions in the fraction of ice and snow cover, as well as changes in their characteristics (e.g., thickness of ice, depth of snow) may influence the exposure of ecosystems to solar UV radiation. This is mainly through: (a) less UV radiation reaching the Earth's surface due to reduced surface reflectivity (see “Surface reflectivity”, below) leading to less exposure; and/or (b) greater exposure to UV radiation for systems formerly under the ice or snow if that protective cover diminishes. The complete removal of ice would lead to a much greater exposure to UV-B radiation, because the transmittance of UV-B radiation through the existing snow-covered ice is much smaller than 1%.49 Recent observations in the Arctic suggest that the summer melt season starts earlier, the winter freeze occurs later, the area of the ice has decreased, and more ice is failing to last through the summer.50–53 Under such conditions, it has been estimated that over the course of one melt season nearly 40% more solar radiation would enter the ocean system.54 In recent years the extent of the northern ocean's ice cover has declined, with large interannual variability;55 while in Antarctica the sea-ice has been expanding since the 1980s.51
The combined direct or indirect effects of these climate change-related factors are likely to influence the levels of solar UV radiation in the future and modulate the effects of the projected recovery of ozone. This interaction, which depends on latitude and on the emissions of GHGs, increases the complexity of assessing the future levels of solar UV radiation at the Earth's surface. Projections for these factors by climate models can be used to estimate the UV radiation in the future; however, with large uncertainty, as discussed later.
Other factors affecting UV radiation
As UV radiation propagates through the atmosphere, in addition to being affected by ozone, it is modified through absorption and scattering by atmospheric constituents, including aerosols and clouds, and by reflections on the Earth's surface. The effects of the most important factors are discussed in the following sections based on established knowledge and on new findings. The effects are discussed in order to assess the relative importance of these factors on the UV irradiance that reaches the surface in the context of ozone and climate changes. In addition to UV-B irradiance, the erythemally weighted irradiance (UVery) and the UV Index (UVI), both defined below in “Biological effects of UV radiation”, are discussed in the following as these quantities appear frequently in the cited literature.
Aerosols
Aerosols (particles suspended in the atmosphere) interact with solar photons and thus can have a significant effect on the atmospheric transmission of solar radiation (see also Madronich et al.56). These particles may be natural (e.g., wind-generated dust and sea salt), anthropogenic (e.g., sulfate, soot, and organic particles), or a mixture of both. The particles scatter and absorb sunlight, with relative probabilities that are complex functions of their size, shape, and chemical composition. They have important effects on air quality and climate, and a considerable body of knowledge has been developed on their sources, properties, and sinks.57,58 Observational methods include the evaluation of trends in visibility,59–64in situ determination of size-resolved chemical and thermodynamic properties,65–68 and remote global-scale detection from ground-based networks and satellite platforms. The AERONET network provides total (scattering + absorption) aerosol optical depth, τ, at a wavelength of 340 nm as well as several visible wavelengths; but absorption optical depths, τabs, are only available at wavelengths of 440 nm and longer.69,70 Satellite-based instruments measuring aerosols include the MISR, MODIS and CALIOP.71–73 Global climatologies of aerosols have been developed based largely on satellite observations.74,75
Many observations have documented reductions in ground-level UV irradiance in the presence of aerosols.5,76–78 Reductions range from a few percent or less in non-polluted locations, such as New Zealand,79,80 to over 50% in polluted cities, such as Mexico City,81 and can be more than 90% for biomass burning aerosols, such as in Russia in 2010.82 The reductions are typically greater at UV than at visible wavelengths, implying that the aerosol optical depth (AOD) is larger at these wavelengths as well. Quantitative effects depend on aerosol type, and enhancements compared with clear-skies may even occur in some conditions, such as in bright scattering hazes.83 Extrapolation from visible wavelengths is often based on a simple power model for AOD:
where
α, the Ångström exponent, parameterizes the strength of the wavelength dependence and has typical values between 0.5 and 2.0 at visible wavelengths.
69 Extrapolation to UV wavelengths is often a reasonable approximation for scattering but less so for absorption, which is more dependent on chemical composition. Some aerosols (
e.g., sea salt, sulfate, and nitrate) have negligible absorption at visible as well as at UV-A (315–400 nm) and UV-B wavelengths. For dust and soot, the absorption spectrum is sufficiently broad that UV properties can be estimated by extrapolation from visible wavelengths. However, for organic aerosols the state of knowledge is extremely poor, as these particles, depending on their origin and environmental conditions (
e.g., humidity), have highly variable chemical composition. So called “brown carbon,” which is mostly composed of combustion-derived organic aerosols, is now recognized as a significant contributor to climate radiative forcing due to its absorption of solar radiation at visible wavelengths,
84 and is likely to have even larger effects on UV spectra. Measurements on organic particles derived from the burning of biomass show Ångström exponents for absorption as high as 6–7 when extrapolated into the UV range,
85,86 and UV mass absorption coefficients in the range 1–10 m
2 g
−1, with the latter value approaching that of black carbon.
The total AOD includes both scattering and absorption, but it is predominately the absorption that is most important in reducing the intensity of UV irradiance at the Earth's surface. For example, decreases in AOD account for 4.2% of the UV-A irradiance increase at Thessaloniki during 1998–2006, while the additional 2% increase can only be explained if the absorption efficiency of aerosols has also decreased over that period.87 The relative importance of scattering is defined by the single scattering albedo (SSA),
so that the scattering component is
ωO ×
τ while the absorption component is (1 −
ωO) ×
τ. Based on radiative transfer model calculations (TUV, http://acd.ucar.edu/TUV) with typical input parameters (TOC: 300 DU, solar zenith angle (SZA): 20°, surface reflectivity: 0.05),
Fig. 2 illustrates how clear-sky surface erythemal irradiance (UV
ery) depends on the total AOD and on SSA, for different target orientations. With non-absorbing aerosols (
ωO = 1), reductions are small and enhancements can even occur for vertically oriented cylindrical surfaces. In contrast, strongly absorbing aerosols (
ωO = 0.6) cause significant reductions in radiation regardless of the target geometry.
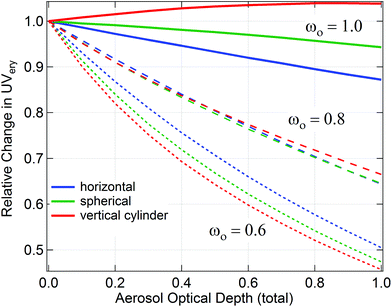 |
| Fig. 2 Variation of UVery with aerosol optical depth, for different values of the single scattering albedo, ωO, and different receiver geometries (horizontal, spherical, and vertical cylinder). | |
Direct measurements of ωO at UV wavelengths are difficult because the absorption by aerosols co-occurs with scattering, and with absorption by gases, especially ozone, but also NO2, or SO2 at UV-B wavelengths. Fig. 3 presents a summary of such studies. Values below 400 nm cluster near ωO ≈ 0.8–0.9, which is below the typical visible values of about 0.9 or larger. The different results are almost certainly due to different aerosol types present, and more recent studies noted the strong absorption of UV radiation in biomass-burning aerosols.82,85,88
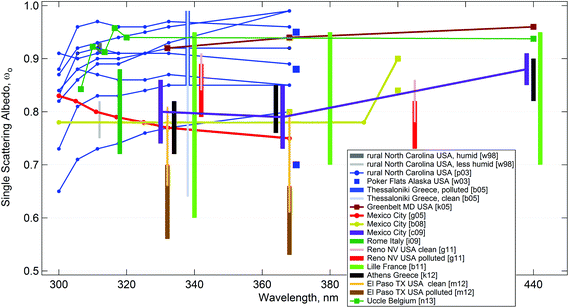 |
| Fig. 3 Summary of available measurements of aerosol single scattering albedo, ωO, at ultraviolet wavelengths from various sources: w98;89 p03;90 w03;91 g05;92 b05;93 b08;94 c09;95 i09;96 g11;97 b11;98 m12;99 k05;280 k12;281 n13.282 | |
A likely explanation for this enhanced absorption of UV radiation is the presence of organic material in the particles. A major new insight of the past decade is that organic aerosols (OA) are more abundant than previously thought, often exceeding the concentrations of sulfate aerosols.65,100,101 They have both natural and anthropogenic sources, the largest being the photo-oxidation of hydrocarbons emitted by vegetation, but other major sources include the combustion of biomass, and the production and use of fossil fuels. Many UV-absorbing organic chromophores have been identified in collected aerosol and rain samples, including conjugated carbonyls and nitrates,102 nitroaromatics,103,104 and organic peroxides.105 Laboratory-generated OA, such as from smog chamber simulations of the oxidation of biogenic hydrocarbons, are found to absorb below 400 nm but not necessarily at visible wavelengths.106 The atmospheric aging of OA also appears to increase UV absorption,107 although photo-bleaching has also been reported.108 Therefore, OA have the potential to induce large variations in surface UV radiation, but quantification is still very uncertain and caution should be used when estimating changes in UV radiation in regions where large concentrations of these organic particles are found.
Trends in aerosols over the past few decades have been derived from the analysis of surface radiation network data and satellite-based observations. Decreases in aerosols have occurred over the US and much of Europe109,110 and the associated brightening has been detected at visible and UV wavelengths.111–113 However, increases in aerosols have occurred in South and East Asia.74,114 In some cases, the mass concentrations of aerosols have decreased but the AOD has still increased due to a shift in the size distribution of the aerosol particles.115 Historical (1850–2000) reconstructions of anthropogenic and biomass burning aerosols have been derived summarising the known historical changes.116
The future trends of aerosols are of great interest to climate studies, and scenarios spanning a large range of uncertainties have been developed.117,118 Global emissions of sulfate may already have peaked two decades ago and may now be decreasing, while those of fossil fuel black and organic carbon are expected to peak in the next few decades. The evaluation of the effects on UV radiation is relatively straightforward for sulfate and black carbon, but is highly uncertain for organics, especially if these absorb UV radiation as discussed above.
In many cases, it is difficult to separate anthropogenic from natural influences, for example, changes in dust or sea salt from winds affected by climate change, or changes in biogenic aerosols following deforestation or other major changes in land use. This adds uncertainty to the future projections because of the challenges in modeling the complex interactions.
Clouds
Clouds play an important role in modifying the solar UV radiation that is received at the Earth's surface, generally leading to attenuation, but in some conditions to enhancement. A recent example of the latter is from measurements in Spain, which have shown that clouds can enhance UVery by up to 22%.119 Reductions of irradiance depend on cloud cover, depth and type (water/ice), and can be moderated by surface reflectivity, particularly when the latter is high (e.g., snow, ice), leading to increased irradiance at the surface through multiple scattering of radiation (see the discussion in the next section). The effects of clouds are more pronounced in the visible than in UV wavelengths. Even under skies covered completely with clouds, the UVI can still be high: maximum UVI values close to 10 and average values of about 3 were measured at an urban location in Brazil (19.9° S; 858 m altitude) under overcast conditions, predominantly of cumulus clouds.120 Typical noon UVI values at this location under cloud-free skies are about 8.3. Cirrus clouds are formed of relatively large ice crystals, which efficiently scatter the solar radiation towards the ground, with only small losses compared with the clear-sky case. According to model calculations based on the global estimates of the optical depth of cirrus clouds averaged over the period of 1984–2007, surface UV-B radiation has been attenuated on average by up to ∼2% compared to clear skies.121
In the Arctic region, clouds could be affected by the loss of sea-ice. Based on the satellite data for the period of 2000–2010, a 1% decrease in sea-ice cover leads to a 0.36–0.47% increase in cloud cover, suggesting that a further decline in sea-ice cover would result in an even cloudier Arctic.122 Due to the complexity of the processes involved, it is difficult to simulate the impact on UV radiation from the combined reduction in sea-ice and increase in clouds.
The spatial and temporal inhomogeneity of clouds makes it difficult to accurately assess and quantify their effects on radiation. Thus, empirical parameterizations are often used to describe their effect, such as the cloud modification factor (CMF), which is defined as the ratio between the measured surface irradiance to the corresponding clear-sky value. It describes the average effect of clouds, implicitly taking into account the optical thickness of clouds (COT). A clear exponential dependence between the CMF derived from UV measurements and the COT from a Cimel sun-photometer was found; for COT between 10 and 50 the CMF ranged from 0.7 to 0.25.123
Since our last assessment124 there has been little new information on how clouds affect the solar UV radiation received at the Earth's surface. Experimental evidence is constrained by the uncertainty in the measurements and by the complexity of the cloud characteristics. Climate change is projected to alter the amount of clouds over particular areas and, as the ozone layer recovers, clouds will have an important role in controlling the future levels of solar UV radiation that would be available for the ecosystems.
Surface reflectivity
The reflectivity of Earth's surface, usually referred to as “surface albedo”, is defined as the ratio of the reflected to the incident amount of radiation. The radiation reflected upwards from the surface undergoes subsequent scattering by air molecules and particles, resulting in enhancement of the irradiance at the surface. These effects are greater when the surface is covered by a highly reflecting material, such as snow or ice. Most materials have low UV reflectivity, with only snow and ice having UV reflectivity greater than 0.5, whereas many surfaces have relatively high reflectivity in the visible. Consequently, the effects of ice and snow are more readily apparent for radiation in the UV than in the visible region. The sea-surface also reflects radiation impinging on the water, but less effectively compared to snow and ice. The roughness of surfaces also affects their reflectivity. The fraction of reflected radiation generally increases with the angle of incidence of the photons; hence it depends on season and latitude. In the Arctic and Antarctica, this effect becomes more important due to the larger SZAs and the prevalence of diffuse radiation in these regions. Spectral measurements of UV irradiance at Ny Ålesund, Svalbard, (79° N) revealed an enhancement in clear-sky irradiance at 320 nm of about 15% between two sites affected differently by the reflectivity of the snow-covered surface and the partially ice-covered ocean. The effect was doubled under overcast conditions.125 The deposition of black carbon aerosols on snow or ice may substantially reduce the reflectivity, resulting in weaker solar irradiance at the surface. For example, during the snow-melt period the reflectivity at Sodankylä, Finland, decreased from 0.65 to 0.45 at 330 nm and from 0.72 to 0.53 at 450 nm, partly due to the deposition of black and organic carbon.126
In mountainous regions, the reflection of radiation may occur both on the surface (usually covered by snow) and from the top of clouds located below the altitude of the site, while multiple reflections may occur between snow-covered surfaces and the base of the clouds. These complex processes can result in considerable enhancement of the incident irradiance. At Sonnblick, Austria, clouds below the observatory increased the average reflectivity by 0.28 ± 0.15, leading to an increase in the irradiance of between 2% and 14% in most (∼75%) of the investigated cases. Compared to snow-free conditions, an enhancement of 22% in irradiance at 305 nm arose from a mean reflectivity of 0.68 under clear-sky conditions. The analysis of total sky images revealed that an enhancement could also be observed when the solar disk was obstructed by clouds or under overcast skies.127
In the context of climate change, reductions in the surface reflectivity due to melting snow or ice would result in a reduction of irradiance over land, but to an enhancement of the irradiance received at and under the sea-surface in regions where sea-ice disappears. This situation may occur, for example, in the Arctic during the summer period in the 2030s.46 Model simulations for several scenarios suggest that the snow depth in April on Arctic sea-ice will decrease over the 21st century. This is due mainly to the loss of sea-ice area in autumn and, to a lesser extent, in winter,128 which results in a smaller snow accumulation. When the snow depth becomes shallower, the reflectivity is reduced and, in turn, the UV radiation at the surface is also reduced, while more radiation is transmitted through the ice below the snow.
The sea-ice cover of the Chukchi and Beaufort Seas in the Arctic is currently undergoing a fundamental shift from multiyear ice to first-year ice, which is generally thinner and more spatially heterogeneous with a more complex pattern of reflection and transmission of solar radiation.54,129 When the annual Arctic sea-ice starts melting, it becomes less reflective than old ice, leading to a reduction in UV irradiance at the surface. In Antarctica, the sea ice is generally expanding rather than shrinking. This expansion is not uniform, but varies regionally. Glaciers in western Antarctica have become thinner130 and the loss of ice sheets is projected for the future in this region.131,132
Reductions in surface reflectivity are expected to play an important role for the levels of UV radiation in the future over areas that, in the past, were covered by ice or snow, such as the high and polar latitudes and the high mountains. Implications can be expected for ecosystems in these areas, either from the reduced (mainly terrestrial systems) or from the enhanced (mainly aquatic systems) exposure to UV radiation. Compared to other regions, except for smaller, high-reflecting areas (e.g., salt lakes affected by dust deposition), the changes in reflectivity are likely to be small and thus unlikely to have a significant effect on UV.
Solar activity
Solar activity, particularly the 11-year solar cycle, influences UV-B radiation that penetrates to the surface of the Earth, mainly through changes induced in stratospheric ozone, rather than directly due to increased solar emission. In the upper stratosphere, solar activity affects the photochemical production of ozone by UV-C (200–280 nm), while in the lower stratosphere it affects ozone predominantly by changing the atmospheric circulation.133 This latter effect is the most important for UV radiation at the Earth's surface because it occurs in the layer where ozone is abundant.
Recent observations from the SIM and SOLSTICE instruments onboard the SORCE satellite revealed an ‘exceptionally’ low minimum in the solar activity, with larger reductions in the emitted UV-C radiation during the declining phase of the 11-year solar cycle (2004–2008) than at the same phase of previous solar cycles.134 These reductions were about 8 times larger than expected by semi-empirical models.135,136 The inconsistencies of these observations with the perception of variations in solar irradiance from earlier measurements and models have been assessed recently,137 along with the relevant implications for the variability of stratospheric and total ozone. The inclusion of these new observations of solar variability in photochemical models and in CCMs revealed decreases of ozone in the upper stratosphere and mesosphere (related to photochemical processes). This would allow the penetration of more UV-B radiation to lower altitudes in the stratosphere and below. However, it has been suggested that the penetration of UV-C in the lower stratosphere will enhance the ozone production there (self-healing effect) and, in turn, reduce UV-B radiation penetration to the Earth's surface.136,138 The net effect on UV-B has not yet been quantified.
Recent studies139–143 suggest that solar activity may evolve into a declining phase in the course of the 21st century resulting in weaker emission of solar UV radiation. CCM simulations for the future showed that such strong reductions in UV-C radiation would lead to a significant decrease in the production of stratospheric ozone from the photolysis of oxygen. This would slow down the recovery of stratospheric ozone by more than 10 years or even cancel it,144 leading to greater levels of UV-B radiation at the ground for as long as the concentrations of stratospheric ozone remain small. These effects are most pronounced in the region between about 40° S and 40° N, where UV-B radiation is already high, and would be likely reinforced by the projected strengthening of the Brewer–Dobson circulation.
The detection of the effects of solar activity on the surface UV radiation measurements is difficult, as they are masked by stronger natural variations due to other factors. An average decrease of about 1.8 ± 1.0% in the ground-level irradiance from solar maximum to solar minimum for the UV-A and 2.4 ± 1.9% for the 400–600 nm spectral band was reported by correlating 17 years of spectral solar irradiance measurements at the South Pole with the 10.7 cm solar radio flux (indicative of the 11-year solar activity).145 As the effects appear to be too large to originate directly from differences in the radiation emitted by the Sun, it was suggested that these decreases are partly due to a small variation in atmospheric attenuation with the solar cycle, with the greatest attenuation occurring at the solar minimum. However, there is no experimental proof for this suggestion.
Although the direct influence of solar activity on the UV radiation at the surface is small, the indirect effects, through changes in the production of ozone, could be more important. If a substantial solar minimum occurs in the future, it may influence global climate and the ozone layer, and could lead to increases in UV-B radiation at the surface.
UV radiation changes and trends derived from measurements
Measurements of UV radiation
Ground-based.
With a few exceptions,146,147 the coordinated measurements of UV radiation from the ground started in the late 1980s after the discovery of the ozone hole.148 It is therefore not possible to directly assess the changes in UV radiation for the entire period between the 1960s (i.e. the time before concentrations of ODSs in the atmosphere became important) and the present. Several ground-based networks now provide data records in excess of 20 years with instruments deployed in the U.S.,149,150 Canada,151,152 South America,153,154 Europe,113,155–158 New Zealand,80,159 Australia,160 the Arctic,161 and Antarctica.162 Relatively few measurements have been performed historically in Africa, the Middle East, and Asia, but several programs have recently been established in Nepal,163 Thailand,164,165 and China.166 By the mid-1990s, the technology of UV radiation measurements with spectroradiometers had already reached a level of accuracy that would allow the detection of changes in UV of a few percent at stations with appropriate quality control protocols.167 A European project has been completed that aimed to provide traceable solar UV irradiance measurements with an uncertainty of less than 2% (http://projects.pmodwrc.ch/env03/). However, the methods developed in this project have not yet been implemented in operational UV monitoring.
Recently, array spectrometers have been adapted for spectral irradiance measurements in the UV. These instruments have been used to quantify the effects of solar UV-B radiation in terrestrial ecosystems (see Bornman et al.31). However, being single monochromators, they are susceptible to stray light problems in the UV-B.168,169 Efforts have been made to determine the uncertainty of this type of instrument.170,171 Because of their large uncertainties compared to scanning spectroradiometers, these instruments are not yet widely used in UV monitoring programs.
Spectroradiometric measurements of UV irradiance on a flat, horizontal surface are the most common and are generally considered the most accurate method to quantify UV radiation. However, this geometry is not the most appropriate to gauge the exposure levels of humans and most animals because the anatomical distribution of UV exposure is highly heterogeneous, poorly correlated to surface irradiance, and, in the case of humans, influenced by factors such as posture, orientation to the sun (see Fig. 2), skin complexion, clothing, and other sun-related protective behavior.172 In principle, the most accurate method to quantify human exposure is to measure the incident solar spectral radiance distribution (which may originate from the Sun, the sky, or radiation scattered upward from the ground) and integrate this distribution over all exposed parts of the human body.173 The development of a system capable of measuring sky radiance at different zenith and azimuth angles within seconds rather than minutes enables new possibilities to study the spectral influence of fast changing cloud conditions without the disadvantages of scanning instruments.174 This method is still under development and has not yet been reported for exposure studies. In an alternative approach,172 measured global-horizontal, direct-normal, diffuse-horizontal and upwelling irradiance were combined with a three-dimensional numerical model of the human body to calculate exposure. An important conclusion of this study, as well as of Seckmeyer et al.,173 was that the contribution of diffuse UV radiation to total sun exposure is larger than commonly expected, explaining almost 80% of the cumulative annual exposure dose.
Satellite based.
The estimation of UV irradiance at the ground has been repeatedly undertaken from various satellite-borne sensors.175–177 As these products are mainly derived by models fed with measured or estimated radiation-related parameters, they are associated with relatively large uncertainties, which vary according to location, season, atmospheric situation and the characteristics of the satellite instrument. Known sources of errors that affect the accuracy of the derived surface UV irradiance include: absorption and scattering by tropospheric aerosols, inhomogeneities of clouds, assumptions or estimations of the surface reflectivity, variability of altitude within the sub-satellite pixel, various modeling parameterizations, and the inability of current satellites to distinguish between clouds and surfaces covered with snow and/or ice. It is important to note that ground-based observations are point measurements while satellite observations are representative for a pixel of several square kilometers. This difference must be taken into account when comparing satellite- and ground-based measurements; particularly when the ground-based instrument is located in a non-homogenous area (e.g., mountains).
In the previous assessment124 it was noted that, although satellites have the advantage of near global coverage, satellite-borne instruments cannot adequately probe the boundary layer (approximately the lowest 1–2 km) of the atmosphere. Therefore, they tend to overestimate UV radiation when absorbing aerosols are present,176 particularly under clear skies. In a recent study in Santiago, Chile, a city with heavy air pollution and complex surrounding topography, this effect was quantified, reporting an average overestimation of UVI by about 46% from the Total Ozone Mapping Spectrometer (TOMS) for the period 1995–2007, and by about 47% from the Ozone Monitoring Instrument (OMI) for the period 2005–2007.153 These results were qualitatively confirmed by two other studies.177,178 Similar results were found for four locations in Thailand,164 with average biases between 40% and 60%. Smaller biases were reported for locations with smaller aerosol concentrations. In France, UVI derived from OMI and the Global Ozone Monitoring Experiment, (GOME-2) was found to be larger by about 6% during 2008–2009,179 while in Southern Spain, for the period of 2004–2008, the average bias was about 12%, rising to 19% for days with large aerosol optical depth (>0.25 at 440 nm).180 The UVI derived from TOMS has been also compared with ground-based data at 27 stations of the USDA network, showing results consistent with the above studies, with an average positive bias in the satellite estimates of the order of 15% over all sites. Under clear skies, the biases can be either negative (up to 3.4%) or positive (up to ∼24%), depending on the amounts of tropospheric aerosols and UV-absorbing air pollutants.181
A comparison of the satellite retrieval of UV irradiance from OMI with UV spectra measured at six Austrian sites with altitudes ranging between ∼600 and ∼3100 m concluded that the satellite estimates were significantly smaller (average ratio 0.89, range 0.6–1.35) for most stations due to erroneous correction for the effects of clouds.182 In contrast, under cloud-free conditions, the satellite data were closer to the ground-based measurements, but they cannot distinguish between mountain and valley sites due to the large variability in altitude within a short horizontal distance, smaller than the size of a pixel. The main deficiencies in the satellite retrieval algorithm arose from the incorrect determination of the effective surface altitude and albedo due to the complex topography.
Such deficiencies have been taken into consideration in the Semi-Analytical Cloud Retrieval Algorithm (SACURA), in which the background spectral albedo is properly specified and cloud parameters are derived from the infrared sensors of the satellite.183 Comparisons of estimates based on satellite data and radiative transfer modelling with observations at two locations in Belgium revealed a good agreement, with correlation coefficients of 0.88 and 0.91 for UV-B and UV-A irradiance, respectively.
Variations of UV radiation in time and space
UV radiation at the Earth's surface varies with the season, time of day, latitude, and altitude. It is also affected by the absorption and scattering processes from atmospheric constituents, as discussed above.
Latitudinal variations in annual doses of UV-B and UV-A have been assessed with high-resolution measurements from ground-based spectroradiometers that comply with the quality standards of the Network for the Detection of Atmospheric Composition Change (NDACC)184 (Fig. 4). For all sites, the annual averages were derived from at least 10 years over periods where trends in irradiance were small. As expected, doses of UV-B and UV-A are generally largest close to the equator and smallest at high latitudes. Doses at high-altitude sites (South Pole, Mauna Loa, Boulder and Summit) are larger than for sites located at similar latitudes but at sea level.5 This is most obvious when comparing data from Barrow (71.3° N; 8 m altitude) and Summit (72.6° N; 3202 m altitude), where the annual doses of UV-B and UV-A at the latter site are about 58% and 83% larger due to higher elevation and different surface reflectivity, respectively. Surface reflectivity in the order of 0.98 also contributes to the relatively large doses at the South Pole and Summit, while attenuation of UV radiation by aerosols is responsible for the relatively low dose at Tokyo. Latitudinal gradients are stronger in the UV-B than the UV-A region, partly because photons travel a longer path through the atmosphere for the lower solar elevations prevailing at higher latitudes, allowing a greater absorption of UV-B radiation by ozone. Another factor contributing to the differences in gradients of UV-B and UV-A is the relatively small ozone column in the tropics. As a consequence, the ratio of the annual dose of UV-B/UV-A is roughly 0.03 close to the equator, 0.02 at mid-latitude sites and less than 0.02 at high latitudes (Fig. 4, lower panel). It is interesting to note that the UV-B/UV-A ratios are not very different in polluted locations, such as Tokyo, compared with clean-air sites, suggesting that the optical depth of aerosols in the UV-B is not very different from that in the UV-A region, and/or that the wavelength dependence of the single scattering albedo throughout the UV region is small (see Fig. 3).
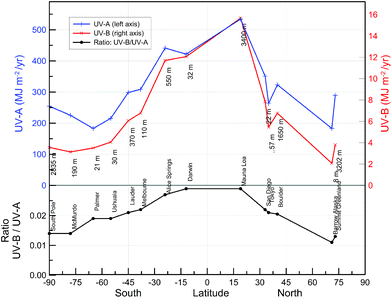 |
| Fig. 4 Latitudinal variation of UV-A (315–400 nm) and UV-B (280–315 nm) annual dose (top) and the ratio of UV-B/UV-A dose (bottom). | |
It has long been known that mid-latitude UV levels in the summer are larger in the southern than in the northern hemisphere.185 Factors contributing to this disparity include the smaller Sun-Earth distance during the southern-hemisphere summer, plus smaller ozone columns and less attenuation by aerosols in southern latitudes. However, for annual doses, the hemispherical differences are relatively small (Fig. 4, upper panel).
Satellite observations indicate that the greatest UV levels at the surface of the Earth occur in the Altiplano region of Peru, Bolivia, Chile, and Argentina, where the UVI in summer may exceed 20.186 The large UV levels in this region can be attributed to small SZA, overhead Sun, small total ozone, high elevation (hence less aerosol and unrestricted horizon), and minimum Earth-Sun separation in the austral summer. These findings have recently been confirmed by ground-based spectroradiometric measurements at the Chajnantor Plateau (23° S, 67° W; 5100 m altitude) of the Atacama Desert in Northern Chile.187 The measured UVI peaked at a value of 20 under broken cloud conditions and was 18 for clear skies. Very high UVI values were reported for locations in Tibet in 2008–2010.188 The measured monthly mean UVI in July was 14.5 in Tingri (28.7° N; 4335 m altitude) and 12.9 in Lhasa (29.7° N; 3683 m altitude), with a peak value of 20.6 in Tingri. Extremely high UV levels (UVI of up to 19 for clear sky and up to 22 under broken cloud conditions) were also measured at sea-level in the tropical Pacific (3.6° S, 85° W), when total ozone was 234 DU.189 These values are more than double those that are common at northern mid-latitudes in summer.190 More recently, UVI values higher than 40 have been reported at Licancabur, Bolivia (5916 m altitude).191 However, these data are inconsistent with the satellite-derived ozone columns. Further work is needed to verify the result.
During the Arctic spring of 2011, when total ozone was extremely small, greatly increased levels of UV radiation were recorded at thirteen Arctic and sub-Arctic ground stations. Measurements of the noontime UVI during the low-ozone episode exceeded the climatological mean by up to 77% at locations in Alaska, Canada, and Greenland, and by up to 161% in Scandinavia.161 The cumulative UV dose integrated over the duration of the low-ozone period increased by 40–50% at several sites in the Arctic and Scandinavia, and exceeded the climatological mean by more than 3σ at seven sites and by more than 4σ at two sites. Despite these large relative enhancements, absolute UV anomalies remained small (less than 0.5 UVI units at the western-hemisphere sites) or moderate (1.0 and 2.2 UVI units at the Scandinavian sites) because the low-ozone episode occurred at a time when the Sun was still low in the sky. In Alaska, Canada, and Greenland, the UV increases can be explained by low ozone, but at the Scandinavian sites they were caused by a combination of low ozone and the absence of clouds. Despite the low absolute levels of UV radiation at northern polar latitudes, biological systems during this Arctic event may have been exposed to greater UV than usually experienced.
The small ozone values observed in 2011 in the Arctic propagated to midlatitudes and, as a consequence, ozone columns over Western Europe were 15–25% below the long-term mean between late March and late April 2011.26 Model assessments suggest that noontime erythemal doses on clear-sky days were larger than usual by about 25% during the affected period. However, such increases have not been confirmed by measurement, so far.
Furthermore, it was found from ground-based and satellite observations that the interannual variability in springtime ozone in the Arctic was correlated with ozone in the summer and explained 20–40% of the summer UV variability at some locations.192 Particularly for spring 2011, it was estimated that the massive ozone depletion in the Arctic increased the March–August cumulative erythemal clear-sky UV dose in the northern hemisphere outside the tropics by 3–4% compared to the climatological mean, with about 75% of the increase accumulated after the breakup of the polar vortex.
Unusually large UV levels were observed between 11 and 30 November 2009 over the southern tip of South America (∼55° S) when the center of the Antarctic vortex became stagnant just south of South America for a three-week period, leading to ozone columns continuously more than 2σ below average. Ground-based measurements for three stations located in this region showed UVI values of 10 to 14, which, for clear-skies, typically only occur at latitudes lower than 40° in the northern hemisphere.11 The analysis of 30 years of satellite observations revealed that this event was unique for the latitude belt of 52° to 56° S.
In August 2011, southern Australia was affected by ozone-poor air originating from tropical latitudes, resulting in measured UVery levels of up to 40% greater than normal.160 This is an example where meteorological factors have produced an anomalous reduction of ozone on an almost continental scale and for a longer duration than previously observed.
The combined effect of all the factors discussed previously may result in very high levels of UV radiation at mid and low latitudes, primarily at high-altitude locations. These high-UV episodes will continue to occur in the future during low-ozone periods, irrespective of the recovery of ozone.
Observed long-term changes in UV radiation
In view of the expected rebound of stratospheric ozone depletion and recovery to levels before the 1980s, an important question is whether this change is reflected in the trends of UV radiation measurements. At most locations, any trends are currently still below the detection threshold imposed by instrument uncertainties and variabilities due to factors other than ozone, such as changes in aerosols, clouds and surface reflectivity. For example, over the period of the peak ozone depletion between the 1980s and 1990s, ozone and cloud effects contributed equally to the UVI increases over populated areas of the northern mid-latitudes.193
Long-term changes in UV radiation can be estimated both from space and the ground. Satellite observations have large uncertainties as discussed above. Changes in UV radiation at different spectral bands over the period of 1979 to 2008 have been derived from a series of polar orbiting satellite instruments for the latitude range of 55° S to 55° N,194 and the results were summarized in the last assessment.124,195 A similar study for the time period of 1997–2010 based on the measurements of three satellites (TOMS/Nimbus 7, TOMS/Earth Probe and OMI)196 has qualitatively confirmed the earlier work by Herman.194 Over this later time period, the derived linear trends in erythemal irradiance (same for UVI) ranged between 0 and +5% per decade between 50° S and 50° N. These positive trends are significant at the 95% level with the exception of the equatorial zone and winter months of both hemispheres. The largest increases were observed during the spring and summer at mid-latitudes of the Southern Hemisphere, where the largest decrease of ozone was observed (Fig. 5). However, most of the UVery changes due to ozone occurred during the 1980s and the early 1990s; therefore, the calculated linear trends do not necessarily reflect a tendency in ozone that would continue into the future. At high-latitudes, the satellite-based estimates of surface UV radiation can be too low by up to 50%, when high albedo from snow and ice cover is misinterpreted as clouds.197 These systematic errors can also affect UV trend assessments. For example, it is difficult to quantify the changes in UV radiation from space measurements over high-latitude locations that are affected by sea-ice variability.194 For these reasons the trends discussed above were derived only for latitudes lower than 55°.
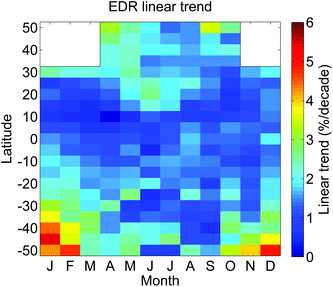 |
| Fig. 5 Monthly linear trends (%/decade) of erythemal irradiance derived from TOMS and OMI data from 1979 to 2010 according to ref. 196. All trends are positive. The data are zonally averaged for every 5° of latitude from 50° S to 50° N and mostly represent changes that occurred in the first half of this period, when ozone depletion was progressing. Adapted from Ialongo et al., 2011.196 | |
The combined effect of the surface reflectivity (RS), clouds, water haze, and aerosols on UV-A radiation, which is not affected by ozone, can be inferred by the, so-called, “Lambertian equivalent reflectivity” (LER). This represents the equivalent scene reflectivity, as seen from space, after the removal of Rayleigh scattering effects.198 The atmospheric transparency T is approximately T = (100 − LER)/(100 − RS), where LER and RS are expressed in percent. Because the reflectivity of most surfaces is small (typically 2–4% over land), a decrease of LER will lead to an increase in T by approximately the same amount. The LER at 340 nm during the past 33 years (1979–2011) has recently been analyzed globally199 and changes in the surface irradiance at 340 nm, but without accounting for the effects of local air pollution sources, can be inferred from this study. Between 1979 and 2011, most of the decreases in LER (resulting in an increase of surface radiation) occurred over land, with the largest wide-spread decreases taking place over the US (0.97% per decade), Brazil (0.9% per decade), and Europe (1.4–1.9% per decade). Over India, southern China, and Indochina, LER has increased by 1–1.5% per decade (Fig. 6). A trend that is twice as large was observed on the west coast of South America, but there was almost no change over most of Australia. These trends can be translated into downward trends in UV-A radiation, caused only by changes in cloud cover and, partly, in aerosols. In the ocean region near the Antarctic Peninsula (160° W–50° W), LER has decreased strongly (>2% per decade), probably due to changes in clouds and sea-ice. Neither of these studies took into account the effects of absorbing aerosols in the estimates of irradiance. Therefore, these trend estimates may not accurately reflect the changes for regions where the concentrations of absorbing aerosols and air pollutants have changed over time, such as in urban areas.
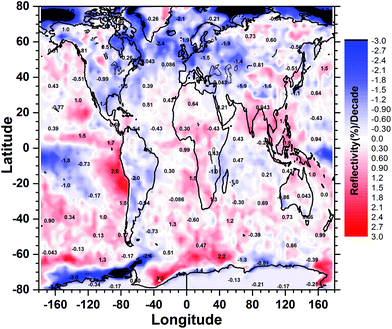 |
| Fig. 6 Trends in LER (combined reflectivity from clouds, aerosols, and the surface) as seen from space. Trends in LER smaller than 0.3% per decade are not statistically significant from zero. Negative trends in LER lead to positive trends in UV-A by approximately the same amount. Trends in LER outside the Polar Regions are caused by clouds and not aerosols. Adapted from Herman et al., 2013.199 | |
The variability of the solar UV irradiance at 305 and 325 nm between 1990 and 2011 has been assessed from ground-based measurements at twelve sites in Canada, Europe, and Japan (latitudes between 25° N to 60° N).200 For these sites at least, this period can be divided into three sub-periods that are characterised by different physical processes. UV radiation decreased during the first period (1991–1994), greatly affected by stratospheric aerosols from the Pinatubo volcanic eruption in 1991. The volcanic stratospheric aerosol layer had two effects: it induced the destruction of ozone through heterogeneous chemical reactions201 and reduced the path-length of UV-B solar radiation through the ozone layer for small solar elevations due to increased scattering by the aerosols.202 Both mechanisms led to an increase in UV-B at the surface after the eruption in 1991, which became smaller in the next years as the aerosol effect decayed. The second period (1995–2006) was characterized by a 1.4% per decade increase in total ozone, coinciding with a significant decline of the aerosol optical depth over the regions of study. This “brightening” effect (increase of the atmospheric transparency T) more than offset the effect of the increase in ozone, resulting in positive UV trends of 9.4% per decade at 305 nm and 8.8% per decade at 325 nm. The third period (2007–2011) showed statistically significant evidence of a slowdown or turning point in the upward trends in UV-B radiation over Canada, Europe, and Japan. These results are consistent with the decrease in LER seen from satellites (Fig. 6) and observations of surface shortwave (300–3000 nm) solar irradiance, which indicate that the brightening effect, which started in the late 1980s and is mostly attributed to changes in cloud cover and aerosols, has slowed down during the last few years or is no longer progressing.45,203–205 However, since some of the sites discussed above200 are located in urban areas that are affected by air pollution, the results cannot be simply applied to a global scale or to sites located at higher altitudes, where aerosols may evolve differently or remain constant.206
Several recent studies that have reported positive trends in UV radiation at European sites caused by a reduction in cloud cover are consistent with satellite observations (Fig. 6). A statistically significant increasing trend of 6.6% per decade in daily maximum UVI from 1993 to 2008 was found for Reading, United Kingdom, presumably caused by a reduction in midday clouds, since ozone remained constant.207 An increase in the erythemal dose of 5.5 ± 1.0% (1σ) per decade was found for April–October in the period of 1976–2008 from ground-based UV measurements at Belsk, Poland (51° N).155 At both locations, the total ozone levels had stopped declining in the mid-1990s, and the observed increases in UV radiation were attributed to decreasing attenuation by clouds. In such locations, which are dominated by cloudy weather, any effects from changing aerosols cannot be detected as the optical depth of clouds is much greater than that of aerosols. Similarly, the trend of increasing spectral UV irradiance at Hoher Sonnblick (Austrian Alps, 3106 m altitude) was also attributed to decreasing attenuation by clouds (5.8% per decade during summer), which was confirmed by the synoptic observations of clouds and measurements of the duration of sunshine. Spectral irradiance at 315 nm was found to increase between 1997 and 2011 from 9.3% per decade at SZA = 45° (spring–summer) to 14.2% per decade at SZA = 65° (whole year).208 Because ozone has been increasing by 1.9 ± 1.3% per decade over this period, the increase of irradiance at 305 nm was smaller (between 5.1% and 7.9%) and not statistically significant.
Trends in the monthly average UVI at Barrow, Alaska (71.3° N), calculated from spectral UV measurements, between 1991 and 2011 were not statistically significant, except for October (−14% per decade).209 This large trend was attributed to decreasing surface reflectivity as the onset of snow cover in autumn has been delayed at this site with a statistically significant trend of 13.6 days per decade. This study emphasizes the importance of climate factors on long-term changes in UV radiation.
After removing the annual variability, the UV irradiance at 305 nm was found to decrease with an average rate of 3.9% per decade for 1991–2011 over four northern hemisphere high-latitude stations (Barrow, Sodankylä, Jokioinen, and Churchill), whereas no significant change was found for irradiance at 325 nm, which is only slightly affected by ozone.210 For the three southern stations examined (Ushuaia, Palmer, and Syowa) no significant changes for either wavelength were found.
The above studies indicate that factors other than ozone have dominated the changes in UV radiation during the last two decades at many sites. They also indicate that UV-B irradiance has stopped increasing at mid-latitude locations in response to the slowdown of the ozone decline.
Simulations of historic changes in UV radiation
As mentioned in our previous assessment,124,195 changes in UV radiation over timescales of centuries to a few decades can be estimated using various proxies or simulated variations of factors that may directly or indirectly affect the solar UV radiation at the Earth's surface (see also Bornman et al.31). Although such estimates have large uncertainties, they are useful in assessing qualitatively the causes of the variations in UV radiation that may have occurred in the past.
A modelling study211 suggests that levels of UV-B radiation in the year 2000 were 2–8% lower than in 1850 over the northern hemisphere and the tropics, and higher by 4% and 30%, respectively, over the mid- and high-latitudes of the southern hemisphere. At most locations outside the tropics, the UV-B changes were caused by changes in tropospheric ozone, except for the northern hemisphere mid-latitudes where changes in tropospheric ozone and aerosols are equally important. These increases in tropospheric ozone in the northern midlatitudes counteracted the increase of UV-B radiation due to stratospheric ozone depletion in the 1900s.
Artificial neural networks trained with measured erythemal irradiance, duration of sunshine, and a combination of measured and modeled total column ozone, were used to reconstruct the daily erythemal dose for Potsdam, Germany from 1901 to 1999 (Fig. 7).212 A positive, statistically significant trend was found for the first half of the 20th century, in line with the observed negative trend in cloud cover. Since 1950, the trend in annual UVery was negative until the mid-1980s, when it turned positive again. However, for both these latter periods, the trends were not statistically significant. These estimates do not include potential effects from aerosols, which decreased substantially in the second part of the 20th century (as discussed above), and would likely result in further increasing of UVery in the 1980s and 1990s. Furthermore, the uncertainty in the ozone data before 1949, and implicitly in UVery, is higher because it has been obtained by a CCM.
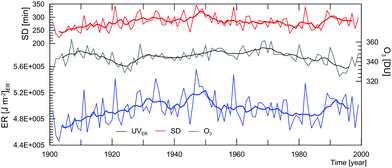 |
| Fig. 7 Reconstructed annual total of UVery time series (blue line, left-hand scale, lower graph, “ER”), mean annual total ozone in DU (gray line, right-hand scale) and mean annual sunshine duration (SD) in minutes per day (red line, left-hand scale, upper graph) for Potsdam, Germany, 1901 until 1999 (thin lines, annual values, thick lines 7-year running averages). Adapted from Junk et al., 2012.212 | |
In a study for Australia, the UVI under cloud-free conditions was calculated with a radiative transfer model over a 50-year period (1959–2009) based on measured meteorological parameters.213 After the 1990s, an overall increase in annual mean UVI of 2–6% relative to the 1970–1980 levels was reported for all latitudes in that country.
UV radiation under the water
The amount of UV radiation under the water surface of the ocean, lakes, and rivers depend on the available radiation field reaching the water surface and additionally on the transparency of the water body. The first is determined by the absorption and scattering processes of solar radiation in the atmosphere, as discussed above, and, at high latitudes, on the amount of ice over the water and the amount of snow over the ice. The transparency of water depends on the concentrations of dissolved and particulate material in the water, with chromophoric dissolved organic matter (CDOM) being the dominant attenuator of solar UV radiation.214,215,32 The attenuation of solar UV radiation penetrating into the water column (quantified with the diffuse attenuation coefficient Kd, m−1) can be measured directly by radiometers or spectroradiometers, but such monitoring programs are sparse.215,216 Recently, measurements of the spectral shape of the surface reflectance from the MODIS satellite have been used successfully to derive globally the diffuse attenuation of coastal waters.216
In some areas, the penetration depth of solar radiation into the water is large, such as in the South Pacific Gyre, where the irradiance at 305, 325, 340 and 380 nm was reduced to 10% of the initial values at 28, 42, 59 and 110 m of depth, respectively.217 These constitute the greatest depths of penetration ever reported for oceanic waters and are comparable with those measured in the clearest fresh waters.218 In contrast, a large attenuation of the solar irradiance was measured in 2004 in the Mackenzie Delta Lakes, Canada, with different gradients in renewal rate of water, concentration of dissolved organic carbon (DOC), and composition of dissolved organic matter (DOM). However, because these lakes are shallow, UV-B and UV-A radiation is still able to penetrate the top 19% and 31% of water columns, respectively.219 Thus, the climate change effects on the composition of DOM may significantly alter the UV radiation environment in such circumpolar delta lakes. The influence of inputs of DOM from rivers into the Arctic Ocean can now be inferred from the analysis of satellite data (see Erickson et al.32).
In Polar Regions, sea-ice prevents a large fraction of UV radiation from reaching the (liquid) ocean surface, while a few centimeters of snow over the ice almost completely blocks the transmission of UV-B radiation.49,220 Solar radiation under “first-year” ice in the upper ocean is spatially heterogeneous and depends on wavelength, thickness of ice, and the area and geometric distribution of melt ponds and bare ice surfaces.129 Although there is an exponential decay in transmission or radiation through the ice sheet, it was reported that the transmission of radiation in the water under the ice can increase with depth when bare and melt-pond sea-ice surfaces are interspersed close to the observation site.129 Projections based on earth-system models and radiative transfer calculations suggest that, compared to the 1950s, up to 10 times more UV-B radiation will enter large parts of the Arctic Ocean by 2100, mainly because of the partial disappearance of sea-ice.221
The future evolution of sea-ice and its snow cover is linked to changes in climate and will likely lead to increases in UV-B radiation reaching the ocean surface beneath the ice. The complex radiation field beneath the first-year sea-ice during the melt-season has significant implications for biological production, biogeochemical processes, and the heat balance of sea-ice and under-ice ocean waters. The effects of UV penetration into the water column, as well as the modification of the ratio UV-B/UV-A by CDOM in the water column, are discussed further in Erickson et al.32
Projections of UV radiation: causes and health effects
Projected changes back to the 1960s and out to the 2090s relative to the present
Surface UV radiation in the future will be influenced by: increases in stratospheric ozone due to reduction in ODSs; changes in ozone and cloud cover induced by increasing concentrations of GHGs; changes in tropospheric UV-absorbing aerosols; and decreases in surface reflectivity at high latitudes and high altitudes. Simulations of these UV radiation levels are usually derived from radiative transfer model calculations that use input parameters estimated by climate models.
In our previous assessment,124,195 we reported estimates from model projections222 suggesting that, by about 2050, the UVery would decrease relative to that in 1980 by 2–10% at mid-latitudes, and by up to 20% at northern and 50% at southern high latitudes, mainly due to the recovery of stratospheric ozone and to changes in cloud cover. In the tropics, UVery was projected to be higher by less than 2%. We also compared model projections between 2100 and 1960 to estimate the effects of climate change on surface UV, because the ozone depletion started after 1960 and ozone recovery would have been completed by 2100. By the end of the 21st century UVery was projected to: (a) remain below 1960 levels due to changes in clouds and GHG-induced transport of ozone at mid-latitudes, (b) decrease at high latitudes (particularly in the Arctic) by 5–10% due to changes in clouds, and (c) increase in the tropics by 3–8% due to decreases in clouds and ozone, induced by GHGs.
However, these projections did not consider changes in aerosols and surface reflectivity. Similar results were reported by another simulation223 that accounted also for the effects of changing albedo and cloud cover. By assuming typical aerosol optical depth and single scattering albedo values over Europe, in addition to projections of ozone, small reductions in erythemal and the vitamin D-effective daily doses were predicted for 2006–2100,224 and were attributed to the recovery of stratospheric ozone and partially to a reduction in the optical depth of aerosols. A recent modelling study focussing on the Arctic Ocean221 projected reductions in UV-B irradiance by the end of the 21st century relative to the levels in the 1950s over a large fraction of the area. Under clear skies, UV-A irradiance is projected to decrease on average by 4–7% (depending on scenario and season), entirely driven by decreases in surface reflectivity, while UV-B is projected to decrease on average by 10–18%, mainly due to the projected ozone recovery. Under all skies, these effects are modulated by clouds, leading to changes in the monthly mean noontime UVI from +15% to −38%, depending on the location and season. Increases in irradiance were found only during August for the latitude band 55–65° N, caused by the projected decrease in cloud cover.
In this assessment, we provide updated estimates of the projections of the previous assessment,124 taking into consideration effects from most factors affecting the UV radiation at the Earth's surface. This analysis is based on recent projections of cloud cover, ozone, surface reflectivity, and aerosols for the period of 1955–2100 by different Earth-System models that were included in the fifth phase of the Climate Model Intercomparison Project (CMIP-5),225 and for the Representative Concentration Pathways (RCP) emissions scenario 4.5. For the ozone projections, the ensemble mean of the CESM1(WACCM) model,226,227 which includes interactive chemistry, was used. These projections have also been used in the fifth IPCC Assessment Report.45 Changes in annually averaged noon UVI due to changes in these factors are shown separately in Fig. 8 between the past (1955–1965 mean) and the present (2010–2020 mean) and between the present and the future (2085–2095 mean).
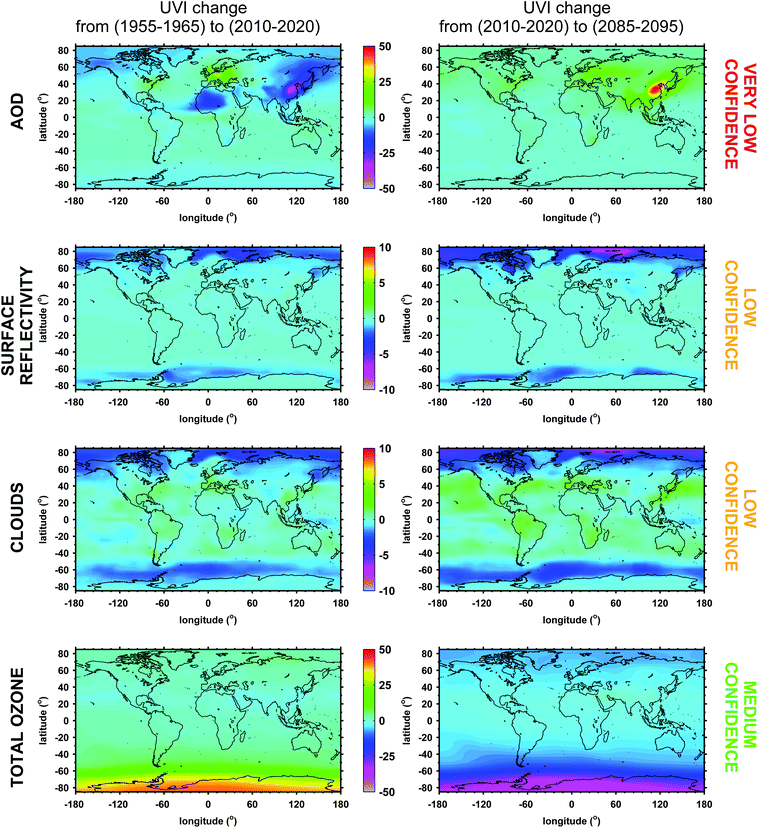 |
| Fig. 8 Simulated annually averaged percentage changes in noontime UVI (or erythemally-weighted UV irradiance) relative to the “present” (i.e. 2010–2020). The left column shows simulated changes since 1955–1965. The right column shows the simulated changes expected from the present to the period 2085–2095. Effects of aerosols, surface reflectivity, cloud cover and total ozone on UVI are shown in each row, with our assessment of the confidence in UVI projections. Note the two different color-scales. | |
Separating the effects of changes in surface reflectivity, aerosols and clouds on UV radiation is potentially challenging because of the interactive influence of these factors on irradiance. Despite these inter-connected effects, the largest changes in reflectivity are projected for high and polar latitudes due to the melting of ice or snow, while the largest changes in aerosols are projected for mid-latitudes and the tropics, particularly over regions with strong anthropogenic activities.
Effects of changes in aerosols.
The future evolution of aerosols and their radiative effects depend on emissions scenarios that may differ from the actual development, both in terms of amount and composition of aerosols. Current trends in air pollution (hence in aerosols) show large regional differences;228 at some regions decreasing (Europe and North America) and at others increasing (Asia). For all emissions scenarios associated with the RCP, aerosols are expected to decrease significantly in the second half of the 21st century globally,229 and particularly over Asia as a result of measures for improvement of air quality,117,118 even though the air pollution there is presently increasing.228
However, even if these scenarios were realistic, there would still be large uncertainties in the simulations of UV radiation due to poor knowledge of the spectral absorption efficiency of aerosols (i.e. of SSA) and its wavelength dependence. Most climate models use input parameters that are appropriate for less absorbing aerosols, which result in an underestimation of their effect on UV radiation. The UVI simulations shown here are based on projected aerosol optical depth values from the earth-system models. Because of the lack of specific SSA predictions, climatological values for SSA75 were used, assuming no change with time, which may also be unrealistic. Finally, over areas dominated by clouds, the projected effects on UVI from changes in aerosols are more uncertain, particularly for large SZAs, for highly absorbing aerosols, and for highly reflective surfaces.
High levels of air pollution in the 1950s and 1960s over some urban areas in Eastern Europe should have resulted in less UV radiation at that time; the simulations show that estimated improvement of air quality since then110 yielded increases in the UVI of up to 40% in the 2010s. Unfortunately, no high-quality direct measurements of UV radiation exist for that period, and neither have the reconstructed data series (see previous discussion) been forced with aerosol data to show this effect. However, measurements in Moscow revealed an ∼4% increase in UV-A radiation from 1981 to 2003 due to the reduction of aerosols.5 In contrast to central Europe, UV radiation may have decreased in eastern Asia (by ∼25%), due to increases in air pollution.230 The decreases in UV (by ∼15%) over north-west Africa are not statistically significant and are probably caused by the large differences among the model projections. By the end of the 21st century, the improvement of air-quality over most of the populated areas of the northern hemisphere may result in small increases in UVI compared to the 2010s by 10–20%, except over China where much larger increases are projected. The projected aerosol effect in the southern hemisphere is generally very small, because sources of aerosols are weaker compared to the northern hemisphere, and aerosols originate mostly from the ocean, while the fraction of land with important anthropogenic activities is very small. Consequently, the assumed changes in aerosol amount with time are generally very small there.110
In our assessment, estimates of the characteristics of aerosols in the past and understanding the effects of changes of aerosols in the future are highly uncertain at present. Although we have tools to carry out the UV calculations, knowledge of the input parameters to the RT models, and a complete understanding of the interactions between the various effects is still lacking. Therefore, the simulated changes in UV radiation shown in Fig. 8 are associated with significant uncertainties, and particularly for the potential aerosol effects, are only illustrative. Despite the uncertainties, it is likely that, outside the polar regions, changes in aerosols and their properties in the future will be more important for the levels of UV at the surface than those from changes in ozone.
Effects of changes in surface reflectivity.
As discussed previously, surface reflectivity is projected to decrease between the 1960s and the end of the 21st century over areas that were covered by sea-ice and snow earlier in this period, whereas in other areas the expected effects, mainly from changes in land-use, would be much smaller. The projected effects on UV radiation are therefore significant only over high and polar latitudes.
Over the Arctic, large reductions in reflectivity due to sea-ice melting have already occurred; hence the simulated UVI is ∼5% lower than in the 1960s, over and close to areas covered by sea-ice. These effects are most pronounced in the summer and autumn, when the sea-ice disappears over large areas. This phenomenon is projected to continue through the end of the 21st century,54 resulting in decreases in UVI with respect to the present by up to ∼10%. In Antarctica, ice cover has generally increased slightly (as discussed above), while a small fraction of sea-ice has been lost in localized regions (mainly over the Weddell Sea), leading to small decreases in annual average UVI of less than ∼2%. Small decreases are projected also for the future since Antarctica will be still covered by snow and ice by the end of the 21st century. However, other factors (e.g., ozone, clouds, and aerosols) that might be different from the present would likely modulate the reflectivity effect.
Effects of changes in clouds.
The effects of clouds on climate are significant and complex; and their representation in climate models continues to be a challenge. Many cloud processes, including aerosol-cloud processes, occur at scales smaller than those resolved in large-scale climate models. Therefore, general circulation models typically use parameterizations to represent a range of cloud properties. A recent assessment reports considerable improvements in the ability of models to account for effects from clouds.231
For the UV projections presented here, the effect of clouds on UVI was estimated through the CMF calculated from projections of all-sky and clear-sky total solar radiation by the Earth system models. Extrapolation of the CMF from visible to ultraviolet wavelengths is based on empirical relationships. Changes in the CMF between the two periods shown are directly translated into changes in UVI due to clouds. The modification of solar radiation by clouds depends on aerosols in the underlying layers and on surface reflectivity, as both lead to increasing multiple scattering of radiation. These factors are implicitly taken into account in the total radiation projections, but their effect on the derived CMF is small.
The projected changes in noontime UVI due to clouds are mostly negative. Cloud cover is projected to increase over the Arctic Ocean due to increased evaporation as sea-ice declines. Therefore, the highest decreases in UVI are simulated for latitudes north of 60° N, and are up to 4% from the 1960s to the 2010s, and about double this between the 2010s and the 2090s. Pole-ward of ∼60° S, reductions of up to 3% in the UVI have been projected for both periods, mainly over the ocean. At all other latitudes the projected changes are very small, ranging between −2% and +2%.
Effects of changes in ozone.
Depletion of ozone led to increases in UV-B radiation during the 1980s and 1990s, and the recovery of ozone will likely lead to reductions of UV-B relative to present levels. According to state-of-the-art simulations by CCMs, it is likely that, by the early-2030s, total ozone columns at mid-latitudes will exceed 1980 values.2 The projected increases in total ozone are due to declining concentrations of ODSs and increases in the concentration of greenhouse and other source gases. Declining ODSs, stratospheric cooling, the possible strengthening of the Brewer–Dobson circulation and other factors are likely to result in a “super-recovery” of mid-latitude ozone columns, after 2040 to 2060, i.e. to levels greater than observed in the 1960s, leading to smaller levels of UV-B.
The future levels of ozone will greatly depend on future emissions of GHGs into the atmosphere, but also on influences from possible volcanic eruptions. Simulations indicate that the differences between GHG scenarios become important only in the latter half of the 21st century, and are largest in the northern mid-latitudes.2 Improved understanding of the effects of the Mt. Pinatubo eruption on stratospheric ozone suggests that a major volcanic eruption in the near future (while atmospheric chlorine levels from ODSs remain elevated) would result in lower levels of stratospheric ozone over much of the globe that would persist for several years.232 In the Arctic, the evolution of springtime ozone in the future is uncertain because it is still debated whether changes in ozone will be driven by increases in PSCs from stratospheric cooling or by decreases in PCSs from stratospheric warming due to increases in planetary wave activity.47
Despite these open issues, the simulations and predictions of total ozone are more certain than the evolution of the other factors discussed above. Compared to the levels in the 1960s the UVI levels in the 2010s are higher only at southern high and polar latitudes where the ozone hole continues to form during the austral spring, and forces the annually averaged UVI to be up to 70% higher. Increases in UVI everywhere else are very small or close to zero. The pattern for the future is a near-complete reversal, as UVI is projected to decrease over Antarctica in the 2090s by up to 40% compared to the 2010s. Decreases in the UVI are projected for the rest of the mid-latitude areas ranging between 5% and 10%. In the tropics, the changes are very small (±2–4%).
Overall effects.
From the above discussion of individual factors that will affect the levels of UV radiation by 2100, it appears that the ozone will continue to be the dominant factor over Antarctica, while clouds and surface reflectivity will dominate the changes over the Arctic. The effects of the aerosols, although highly uncertain, are potentially very important, and will probably dominate future changes in both the UV-B and UV-A radiation in highly populated regions. Because the largest potential effects are also the most uncertain, we do not attempt to combine the four panels to show an overall effect.
In our last assessment, it was projected, on the basis of models available at that time, that there would be increases in UV at low latitudes by 2100 (where the UV is already high). The present assessment does not support that general statement; Fig. 8 shows a more complex picture and any projected increases (due mainly to reductions in cloud) are smaller than provisionally projected.
Effect of the Montreal Protocol on UV radiation
The amended and adjusted Montreal Protocol continues to be successful in reducing emissions and atmospheric abundances of most controlled ODSs, and has been hailed as the most effective environmental treaty ever. As a result of its success, the concentrations of most of the man-made chemicals that led to ozone depletion are declining, and ozone is judged to be on a path towards recovery.2 Despite their long atmospheric lifetimes, by 2012, the total combined abundance of anthropogenic ODSs in the troposphere had decreased by nearly 10% from the peak value in 1993. New estimates of the contributions of specific substances or groups of substances to the decline in tropospheric chlorine and bromine are now available.2
Several attempts have been made to quantify the success of the amended and adjusted Montreal Protocol by comparing the environmental implications of ozone differences in the future “world expected” with those in the future “world avoided” by its successful implementation. A recent model simulation, that included the effects of coupling with the deep ocean,233 showed that without the Montreal Protocol, ozone concentrations would have continued to decline, with an acceleration of that decline in the latter part of this century. In 2070, the stratospheric ozone layer would have collapsed to less than 100 DU worldwide and the peak UVI would have reached values greater than 35 in the tropics; at the sunlit northern polar cap UVI values would have been in the range 5–15, which are similar to or larger than the values found in the subtropics and tropics in 2000. Such an enhancement of UV irradiance at the surface is beyond anything that modern ecosystems have presumably experienced.
Another simulation223 that accounted also for the effects of climate change (e.g., changing albedo and cloud cover), reported the geographical distribution of changes in UVery that would occur without the implementation of the Montreal Protocol. For the no-Montreal Protocol simulation, dramatic increases in erythemal irradiance between the 1970s and 2100 were calculated, with 5-fold increases over populated areas, corresponding to summer UVI in excess of 50.
An early attempt to quantify the health effects concluded that the implementation of the Montreal Protocol has been hugely beneficial to avoid the health risks, such as skin cancer, which are associated with high UV radiation, while there is only a small increase in health risks, such as vitamin D deficiency, that are associated with low UV radiation.234Fig. 9 shows the projected changes in ozone and UVI that would have occurred in the “world avoided” case.234,235 The plots compare only latitudes 50° N and 50° S, but similar patterns are seen for other latitudes. The rate of ozone decline would have accelerated markedly after 2040, reaching minimum values of approximately 100 DU, similar to the lowest values seen during the most severe Antarctic ozone hole, by 2070. By that time, peak UVI values at mid latitudes would have been approximately 3 times as high as in the period prior to the onset of ozone depletion. The present differences in peak UVI values between the northern and southern hemispheres would have persisted and amplified by the 2060s. Summer-winter contrasts in UVI would have been amplified in absolute terms (differences), but reduced in relative terms (ratios), which may have had important implications for vitamin D production.
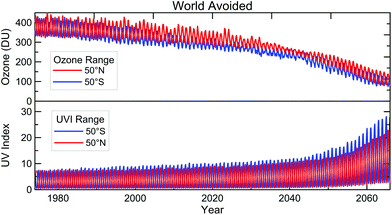 |
| Fig. 9 Simulations of the “world avoided” total ozone (top panel) and UVI (bottom panel) between 1974 and 2075 for latitudes 50° N and 50° S. | |
The effects on projected skin cancer rates were further estimated in a later study that also included the effects of projected future changes in cloud cover.236 The study showed that, due to the decreases in ozone over the latter part of the 20th century, the incidence of skin cancer would rise by approximately 4% around the mid-21st century, but with large geographical differences. Of the regions tested, the largest increases (170–200 cases per million) were projected for the Australian region, but since the skin cancer models used as inputs were developed for the Netherlands and did not take account of behavioral changes in sun-exposure, their relevance to other regions is questionable.
Van Dijk et al.236 predicted that, without the Montreal Protocol, there would have been much larger increases in rates of skin cancer. Even by as early as the year 2030, 2 million cases of skin-cancer would have been prevented yearly, which is 14% fewer skin-cancer cases per year (see Lucas et al.237 for further details). This assumes no changes in human behavior with regard to sun-exposure. However, because there is a time delay of several decades between peak UV and subsequent diagnosis, the increase in year 2030 is attributable mainly to the relatively small ozone depletion that was present around the turn of the century (see Fig. 9). Further studies investigating the health effects early in the 22nd century would give a more realistic assessment of the true benefits of the Montreal Protocol.
Biological effects of UV radiation
The damaging or beneficial biological effects of UV radiation have a unique dependence on wavelength, which is quantified by weighting functions, also called “action spectra”.195,238 Action spectra typically increase by several orders of magnitude towards shorter wavelengths in the UV-B region (see examples in Fig. 10). Because of this wavelength sensitivity, biological effects depend strongly on the spectrum of the incident radiation. The shape of the spectrum depends on the amount of atmospheric ozone and the path of solar radiation through the ozone, which is a strong function of SZA. To quantify a biological effect, the solar irradiance spectrum at Earth's surface is multiplied with the action spectrum for this effect, and the result is integrated over wavelength to derive the biologically effective UV irradiance (UVEFF).
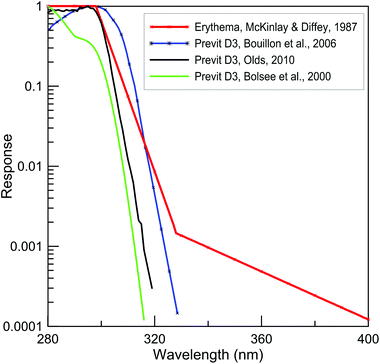 |
| Fig. 10 Biological action spectra for erythema and production of previtamin D3. | |
Sensitivity of biologically effective UV radiation to changes of ozone
The sensitivity of UV radiation to changes of ozone depends greatly on wavelength. Because every biological effect has a unique dependence on wavelength, to quantify the changes in biologically effective UV radiation due to changes in ozone, these wavelength dependencies should be taken into account. The relationship between change in total ozone column (TOC) and change in biologically effective UV irradiance can be quantified in terms of the Radiation Amplification Factor (RAF). For small (<10%) changes in ozone the RAF is simply the relative fractional change in effective UV irradiance with fractional change in total column ozone:
RAF = −(ΔUVEFF/UVEFF)/(ΔTOC/TOC), |
where ΔUVEFF and ΔTOC are the respective changes in UVEFF and TOC. For example, RAF = 1.5 means that a 1% decrease in ozone will lead to a 1.5% increase in biologically effective UV radiation. For larger (>10%) changes in ozone, the power form239 is more appropriate:
UVEFF+/UVEFF− = (TOC−/TOC+)RAF, |
where the subscripts (+ and −) refer to the cases with larger or smaller values of ozone, respectively.
Biological effects that are dominated by UV-B wavelengths have larger RAFs than effects where the contribution from the longer wavelengths is significant. As an example, we illustrate this for the case of vitamin D production by UV radiation, because this topic has received prominence in recent years, and the action spectrum for previtamin D3 production is controversial.240Table 1 shows the RAFs for three suggested action spectra for previtamin D3 production (Fig. 10) compared with the RAF for erythema for typical conditions in January and July. The previtamin D3-related action spectra are for illustrative purposes only and although the accuracy of the currently-accepted spectrum241 has been called into question,240 we are not in a position to advocate any change to it. A comprehensive list of RAFs and action spectra for a large variety of biological effects was included in the previous assessment report.124
Table 1 RAFs for action spectra calculated on the basis of daily integrals for latitude 30° N. This is an update of Table 1 in McKenzie et al.195
Effect |
RAF Jan (290 DU) |
RAF July (305 DU) |
Reference |
Erythema (CIE, standard reference) |
1.1 |
1.2 |
CIE;242 McKinley and Diffey;243 Webb et al.244 |
Previtamin D3 (CIE) |
1.7 |
1.4 |
Bouillon et al.241 |
Previtamin D3 (CIE truncated to 315 nm) |
1.8 |
1.5 |
Bouillon et al.241 |
Previtamin D3 |
1.7 |
1.4 |
Olds245 |
Previtamin D3 |
2.6 |
2.2 |
Bolsee et al.246 |
RAFs also depend to some degree on factors other than SZA and ozone that alter the shape of the solar spectrum, such as extinction by aerosols or the vertical distribution of ozone in the atmosphere.247 Limitations of the RAF and its application to other action spectra have been discussed in more detail elsewhere.239
New numerical parameterizations have recently been developed to calculate the biologically effective irradiance as a function of TOC and solar elevation angle for commonly used action spectra.194 Furthermore, a new method has been devised to calculate RAFs from measurements of UVery and TOC during times when UVery is also affected by clouds and aerosols.248
While the basic understanding of the sensitivity of UV radiation to changes in ozone has not changed since the last assessment, additional studies are now available that corroborate the magnitude of this sensitivity and allow refinement of the values of action spectra.
The action spectrum for erythema
There have been slight variations in the definition of the action spectrum for erythema since it was first introduced.243 The action spectrum for erythema was standardized by the Commission Internationale de l’Éclairage (CIE) in 1987249 and updated in 1998.242 Deviations in erythemal irradiance resulting from the two versions of the action spectrum are less than 0.5% for SZAs <40° and increase to around 2% at 85° SZA244 and the RAF changes by less than 0.02 (Fig. 11). Even though the differences are small, this change is important due to the large number of studies and time series that have been based on the CIE 1987 action spectrum. In accordance with Webb et al.,244 we recommend that the standard action spectrum for erythema recommended by CIE242 should be used in the future.
 |
| Fig. 11 Ratio of radiation amplification factors (RAF) calculated for the CIE 1998 and CIE 1987 erythema action spectra. The figure is based on model spectra that were calculated for different solar zenith angles and total column ozone250 and subsequently weighted with either the CIE 1998 or the CIE 1987 erythema action spectrum. | |
Measurements of personal exposure to UV radiation
To date, the most widely used method for personal UV exposure studies is to equip volunteers with small dosimeters attached to various parts of the body. Dosimeters may be based on photoresponsive films, which change their transmission upon exposure to UV radiation,251–253 DNA molecules in cultures of immobilized spores with a spectral response corresponding to erythema,189,254,255 and photodetectors that convert UV radiation into signals of voltage or current.256–258 The ability to convert doses absorbed by polysulphone (PS) badges into biologically effective solar UV exposure was assessed,259 taking as an example two relevant effects for human skin: induction of erythema and production of previtamin D3. Comparisons of doses derived from PS badges positioned horizontally and at different inclination angles (to simulate various anatomic sites of the human body), revealed larger deviations at large solar zenith angles and/or for highly reflective surfaces.260
More recently, electronic dosimeters have become available, and their use is becoming more widespread.261–263 The accuracy of measurements of two types of personal dosimeters, namely PS films and electronic ultraviolet (EUV) dosimeters using an aluminum gallium nitride (Al27Ga73N) photodetector, have recently been assessed.262 PS dosimeters showed mean absolute deviations of 26% relative to a reference spectroradiometer, with a maximum deviation of 44%. Since the PS doses were derived using a single calibration curve, further experimental investigation of these dosimeters is needed to better assess their accuracy. The calibrated EUV dosimeters showed mean absolute deviations of 15% (maximum 33%), which were partly caused by small, but significant sensitivities to visible radiation (i.e. stray light). It was concluded that calibrating UV sensors by direct comparison with a reference instrument leads to reliable results and that these simple devices are useful to estimate personal UV exposures. They should not be used, however, as an inexpensive replacement for meteorological grade instruments.
Inexpensive, non-scientific instruments for UVI measurements have recently become available. These sensors are part of watches, portable weather stations, and handheld UV meters. The accuracy of several of these devices has been assessed.264 While the measurements of some test devices agreed with those of a reference spectroradiometer to within 20%, some instruments overestimated the UVI by up to a factor of three and hence did not provide trustworthy results.
Health-related exposure to UV radiation
Exposure to sunlight, specifically the UV radiation, has both positive and negative health effects, as discussed in Lucas et al.237 Despite the positive effects, it is excessive sun-exposure that has been of greater concern, because of its adverse effects to humans, terrestrial and aquatic ecosystems, materials, and air quality (see companion papers31,32,56,237,265,266). Quantification of exposure of humans to UV radiation is complex as many factors are involved, including the natural variations of radiation, the orientation of the exposed parts in conjunction with the time and location, behavioral aspects, clothing, as well as effects of reflections on the surroundings.253
This complexity is confirmed by recent exposure studies using personal dosimeters, which include: seafarers of merchant vessels,189 farmers at a mid-latitude site,256 urban dwellers engaging in typical outdoor activities such as shopping, walking, cycling, and sightseeing,257 professional cyclists,254 young (age 9–12) skiers,255 vineyard workers,252 and people engaged in activities such as walking, sitting, and lying.258 From measurements with personal electronic UV dosimeters in New Zealand over a few weeks (outside the peak summer period),261,267 it was shown that cumulative doses received by dosimeters worn on the wrist were typically less than 2% of the available ambient doses, and that the equivalent full body exposure is less than 1% of the ambient. This implies that the people wearing the dosimeters were probably indoors for about 95% of the time. These studies suggest that personal exposure to UV radiation is better approximated by dosimeters and diaries than by measurements of the ambient UV irradiance. Furthermore, the translation of traditional global-horizontal irradiance measurements into exposure levels relevant to humans therefore depends critically on information on behavior and location.
Maximizing the benefits while minimizing the damage is a multifaceted problem in which many of the elements are important and need to be quantified.268 A recent attempt has been made to quantify the available ambient UV doses each month, including both beneficial and detrimental effects, in Northern Eurasia.269
As discussed above, problems arise when one uses UV measurements on a horizontal surface to perform risk-benefit assessments because they do not yield the actual doses people get while they are outdoors, as different parts of the body are exposed at different angles. More realistic UV doses for people who are outdoors engaged in a variety of different activities can be estimated from simple geometrical parameterizations.270
As part of further refinements, the importance of including the effects of clouds and aerosols in parameterizations to derive vitamin D-effective irradiance from erythemal irradiance was highlighted by Feister et al.190 They showed, from 4 years of measurements in Germany, that optically thick clouds can strongly modify the ratio between erythemal and vitamin D-effective irradiance, suggesting that the parameterizations derived for cloud-free conditions are not always applicable.
Similarly, the role of shade has been emphasised by Turnbull and Parisi271 who measured the spectral dependence of the ratio of diffuse to global UV radiation, and showed that under clear skies this ratio decreases with wavelength throughout most of the UV region for wavelengths greater than 300 nm. For example, for unpolluted conditions at SZA = 40°, the diffuse to global ratio decreases from ∼0.6 at 300 nm to ∼0.3 at 400 nm. This means that in the UV-B region, the protection offered is less than one would estimate from our perception of the visible solar radiation. To get protection sufficient for most purposes (e.g., Solar Protection Factor >30) from UV-B radiation in the shade, it is important to ensure most of the diffuse sky radiation is also blocked. Interestingly, because the wavelengths for previtamin D production are slightly shorter than for erythema, this implies that exposure to diffuse light, such as in the shade of trees or buildings, may slightly favor the production of vitamin D while minimizing the risk of erythema in non-covered skin.
The field of atmospheric UV research is plagued with difficulties in nomenclature. A recent report272 highlights some of the issues, taking vitamin-D synthesis, a beneficial effect, as a specific example. Terminologies for the standard vitamin-D dose (SDD) and the minimum vitamin-D dose – for daily sufficiency – (MDD) are proposed, analogous to the standard erythema dose (SED) and minimal erythema dose (MED) that are in common use for erythema. Note that the quantitative value of the MDD is not yet known; nor is there agreement on the recommended minimum levels of the status of vitamin-D (see Chapter 2). In the present literature, the SDD has confusingly been defined in terms of a physiological response. In recognition of the fact that currently accepted action spectra may be revised if new data become available, the continuation of spectrally resolved irradiance measurements will allow reprocessing of biologically effective irradiances and doses in the future.
Effects of geoengineering on ozone and UV radiation
Geoengineering – or “climate engineering” – refers to a broad set of methods and technologies that could be used to deliberately alter the climate system in order to alleviate the impacts of climate change. Solar Radiation Management (SRM) has been suggested as a means to counteract the warming from increasing GHG by reducing the amount of solar radiation absorbed by the Earth's surface. Carbon Dioxide Reduction (CDR) aims at reducing the future concentrations of CO2 by accelerating the natural removal of atmospheric CO2 or increasing the storage of carbon in reservoirs.45
Of those two geoengineering methods, only the SRM would directly influence the amount of UV radiation received at Earth's surface. Space reflectors, injection of aerosols in the stratosphere, or seeding of marine clouds would reduce the amount of UV radiation reaching the surface. In contrast, increasing of surface reflectivity by creating micro bubbles at the ocean surface, growing more reflective crops, or painting roofs and other built structures in light colors may lead to increased surface UV radiation through scattering of reflected radiation towards the ground.
The injection of sulfur dioxide into the stratosphere273 is one of the methods suggested to reduce the amount of solar radiation reaching Earth's surface, through increased scattering of solar radiation to space. However, it is known that stratospheric sulfate aerosols from volcanic eruptions and natural emissions deplete the stratospheric ozone, and similar effects should be expected from stratospheric aerosols introduced for SRM, leading ultimately to increases in the amount of UV-B radiation reaching the surface, which are larger than the reduction achieved from the SRM.
This is further supported by recent modelling studies274–276 suggesting that such interventions would lead to a general decrease in stratospheric ozone concentrations, mainly via changes in photolysis rates, tropical upwelling of ozone-poor air, and an increase in available surfaces for heterogeneous chemistry. Considering the role of very short-lived halogens in the stratosphere (e.g., Bry and Cly), increases in annual average UVery of up to 5% in mid and high latitudes were simulated for the 2040s due to the impact of stratospheric sulfur on ozone.275 Recently, it was projected that the increase in UV-B radiation at the surface due to ozone depletion could be offset in the 2040s by the screening due to the SRM aerosols in the tropics and mid-latitudes, while in polar regions the UV-B radiation would increase by 5% on average, with 12% peak increases during springtime.277
Other potential UV-related impacts of geoengineering have been investigated with models, revealing effects on cloud cover278 and rainfall patterns,279 both of which ultimately lead to changes in UV radiation at the Earth's surface. Model simulations showed that SRM, to counteract a 1% annual rise in atmospheric CO2, suppresses the increases in precipitation that would otherwise accompany the rising GHG, had geoengineering never been used. However, in some of these sensitivity studies extreme and perhaps unlikely scenarios279 have been assumed. For example, the effect of geoengineering with sulfate aerosols was investigated in a world with a different climate by first setting global CO2 levels at an extremely large level of 1120 parts per million (ppm) or four times the pre-industrial level. They found that global precipitation rates would increase by approximately 7% compared with pre-industrial times, but with high spatial and temporal variability. However, when they re-ran the models, with SRM geoengineering included, they found a 4.5% reduction in global precipitation. Again, there was a high degree of variability, but notably, decreases in precipitation rates on land and in oceans were much more similar.
The interactions and feedback of the suggested geoengineering methods with the natural variability of the atmospheric and surface reflectivity are not yet fully explored. The atmosphere is a complex system and any deliberate interventions should be treated with great care as they may have unanticipated adverse effects. Moreover, with the current observing systems, it would not be feasible to assess whether the small intended changes in solar radiation at Earth's surface from the implementation of geo-engineering would have actually occurred.
Gaps in knowledge
Simulations of surface UV radiation for the future are limited in accuracy due to difficulties in assessing the combined effects of clouds and aerosols that are expected to change. Over ice- and or snow-covered areas, these effects are even more complicated. Additional uncertainties for UV projections arise from the scenarios describing the evolution of the atmosphere in the future.
Significant changes in aerosol concentrations are expected in the future (both positive and negative, depending on the region). The effect of these aerosols on surface UV irradiance will depend strongly on their single scattering albedo. However, even for the aerosols that are present in the current atmosphere, this parameter is not well quantified in the UV-B region because of the confounding effects of ozone absorption. Better quantification of the SSA in the UV-B region over a wide range of aerosol types (possibly involving laboratory studies to avoid the effect of ozone) would increase our ability to model the effects of aerosol on the UV radiation at the Earth's surface.
To our current understanding, variations in solar activity lead to decreases in total ozone of up to 3% between the maximum and the minimum of the 11-year solar cycle. However, the relative contributions of solar activity-induced radiative and dynamical effects on ozone are not yet fully resolved. Moreover, there are still uncertainties in the measurements of solar spectral irradiance outside Earth's atmosphere.
Improvement in the understanding of these processes and the availability of higher quality information of the UV-related factors would strengthen our ability to effectively interpret ongoing changes and predict future changes in UV radiation.
Instruments at the ground measure UV irradiance directly and the results are therefore more accurate than the inversion results from satellite data, but the spatial coverage of surface observations is sparse and vast regions of the Earth (e.g., Africa, Siberia, the global oceans, particularly in the southern hemisphere) are not being monitored from the ground. In light of these limitations, robust assessments of long term changes in UV radiation must be based both on observations from space and from the ground.
Assessment of the long-term benefits of the Montreal Protocol requires input from both the atmospheric sciences and the health communities. To date, the health costs of non-implementation of the Montreal Protocol have been calculated only up to the year 2030. Because of the lag between UV exposure and the onset of diseases this is representative only of the changes due to ozone depletion up to the year 2000. This severely underestimates the true benefits of the Montreal Protocol because ozone changes are likely to have become much larger in the latter half of the 21st century (see Fig. 9).
There is incomplete characterisation of many action spectra of interest. Examples are the action spectrum for the formation of previtamin D3 from sunlight and the action spectrum for melanoma in human skin. These are required for quantitative assessments of the environmental effects of future changes in UV radiation, particularly those due to changes in ozone.
Acknowledgements
We acknowledge the contribution of Ilias Fountoulakis, PhD student at the Aristotle University of Thessaloniki, in the modelling of UV radiation projections. GB's contribution was supported by the U.S. National Science Foundation (grant ARC-1203250). KT's contribution was supported by the project FP7-SPACE-2012–313188. Richard McKenzie's participation was sponsored by the New Zealand Government's Ministry for the Environment, and supported through the Ministry of Business, Innovation and Employment's research contract C01X1008.
References
-
WMO
, (World Meteorological Organization): Scientific Assessment of Ozone Depletion: 2010, Global Ozone Research and Monitoring Project, Report No. 52, Geneva, Switzerland 2011, p. 438.
-
WMO
, (World Meteorological Organization): Scientific Assessment of Ozone Depletion: 2014, Global Ozone Research and Monitoring Project, Report No. 55, Geneva, Switzerland 2015.
- S. Tilmes, J. Fasullo, J.-F. Lamarque, D. R. Marsh, M. Mills, K. Alterskjær, H. Muri, J. E. Kristjánsson, O. Boucher, M. Schulz, J. N. S. Cole, C. L. Curry, A. Jones, J. Haywood, P. J. Irvine, D. Ji, J. C. Moore, D. B. Karam, B. Kravitz, P. J. Rasch, B. Singh, J.-H. Yoon, U. Niemeier, H. Schmidt, A. Robock, S. Yang and S. Watanabe, The hydrological impact of geoengineering in the Geoengineering Model Intercomparison Project (GeoMIP), J. Geophys. Res., [Atmos.], 2013, 118, 11,036–11,058 Search PubMed.
- R. S. Stolarski, A. R. Douglass, E. E. Remsberg, N. J. Livesey and J. C. Gille, Ozone temperature correlations in the upper stratosphere as a measure of chlorine content, J. Geophys. Res., [Atmos.], 2012, 117, D10305 CrossRef.
-
WMO
, (World Meteorological Organization): Scientific Assessment of Ozone Depletion: 2006, Global ozone Research and Monitoring Project, Report No. 50, Geneva, Switzerland 2007, p. 572.
- W. Chehade, M. Weber and J. P. Burrows, Total ozone trends and variability during 1979–2012 from merged data sets of various satellites, Atmos. Chem. Phys., 2014, 14, 7059–7074 CAS.
- M. Coldewey-Egbers, D. G. Loyola R, P. Braesicke, M. Dameris, M. van Roozendael, C. Lerot and W. Zimmer, A new health check of the ozone layer at global and regional scales, Geophys. Res. Lett., 2014, 41, 4363–4372 CrossRef CAS.
- B. Hassler, J. S. Daniel, B. J. Johnson, S. Solomon and S. J. Oltmans, An assessment of changing ozone loss rates at South Pole: Twenty-five years of ozonesonde measurements, J. Geophys. Res., [Atmos.], 2011, 116, D22301 CrossRef.
- B. Hassler, G. E. Bodeker, S. Solomon and P. J. Young, Changes in the polar vortex: Effects on Antarctic total ozone observations at various stations, Geophys. Res. Lett., 2011, 38, L01805 CrossRef.
- J. R. Ziemke and S. Chandra, Development of a climate record of tropospheric and stratospheric column ozone from satellite remote sensing: evidence of an early recovery of global stratospheric ozone, Atmos. Chem. Phys., 2012, 12, 5737–5753 CrossRef CAS.
- A. T. J. de Laat, R. J. van der A, M. A. F. Allaart, M. van Weele, G. C. Benitez, C. Casiccia, N. M. Paes Leme, E. Quel, J. Salvador and E. Wolfram, Extreme sunbathing: Three weeks of small total O3 columns and high UV radiation over the southern tip of South America during the 2009 Antarctic O3 hole season, Geophys. Res. Lett., 2010, 37, L14805 CrossRef.
- G. L. Manney, M. L. Santee, M. Rex, N. J. Livesey, M. C. Pitts, P. Veefkind, E. R. Nash, I. Wohltmann, R. Lehmann, L. Froidevaux, L. R. Poole, M. R. Schoeberl, D. P. Haffner, J. Davies, V. Dorokhov, H. Gernandt, B. Johnson, R. Kivi, E. Kyro, N. Larsen, P. F. Levelt, A. Makshtas, C. T. McElroy, H. Nakajima, M. C. Parrondo, D. W. Tarasick, P. von der Gathen, K. A. Walker and N. S. Zinoviev, Unprecedented Arctic ozone loss in 2011, Nature, 2011, 478, 469–475 CrossRef CAS PubMed.
- H. E. Rieder, J. Staehelin, J. A. Maeder, T. Peter, M. Ribatet, A. C. Davison, R. Stübi, P. Weihs and F. Holawe, Extreme events in total ozone over Arosa - Part 2: Fingerprints of atmospheric dynamics and chemistry and effects on mean values and long-term changes, Atmos. Chem. Phys., 2010, 10, 10033–10045 CrossRef CAS.
- H. E. Rieder, J. Staehelin, J. A. Maeder, T. Peter, M. Ribatet, A. C. Davison, R. Stübi, P. Weihs and F. Holawe, Extreme events in total ozone over Arosa - Part 1: Application of extreme value theory, Atmos. Chem. Phys., 2010, 10, 10021–10031 CAS.
- M. Fitzka, J. Hadzimustafic and S. Simic, Total ozone and Umkehr observations at Hoher Sonnblick 1994–2011: Climatology and extreme events, J. Geophys. Res., [Atmos.], 2014, 119, 739–752 CAS.
- M. C. Reader, D. A. Plummer, J. F. Scinocca and T. G. Shepherd, Contributions to twentieth century total column ozone change from halocarbons, tropospheric ozone precursors, and climate change, Geophys. Res. Lett., 2013, 40, 6276–6281 CrossRef CAS.
- M. Salby, E. A. Titova and L. Deschamps, Changes of the Antarctic ozone hole: Controlling mechanisms, seasonal predictability, and evolution, J. Geophys. Res., [Atmos.], 2012, 117, D10111 CrossRef.
- M. Salby, E. Titova and L. Deschamps, Rebound of Antarctic ozone, Geophys. Res. Lett., 2011, 38, L09702 CrossRef.
- J. Kuttippurath, F. Lefèvre, J. P. Pommereau, H. K. Roscoe, F. Goutail, A. Pazmiño and J. D. Shanklin, Antarctic ozone loss in 1979–2010: first sign of ozone recovery, Atmos. Chem. Phys., 2013, 13, 1625–1635 CrossRef.
- J. Kuttippurath, S. Godin-Beekmann, F. Lefèvre, G. Nikulin, M. L. Santee and L. Froidevaux, Record-breaking ozone loss in the Arctic winter 2010/2011: comparison with 1996/1997, Atmos. Chem. Phys., 2012, 12, 7073–7085 CAS.
- J. P. Pommereau, F. Goutail, F. Lefèvre, A. Pazmino, C. Adams, V. Dorokhov, P. Eriksen, R. Kivi, K. Stebel, X. Zhao and M. van Roozendael, Why unprecedented ozone loss in the Arctic in 2011? Is it related to climate change?, Atmos. Chem. Phys., 2013, 13, 5299–5308 CrossRef.
- G. Bernhard, G. Manney, V. Fioletov, J.-U. Grooß, A. Heikkilä, B. Johnsen, T. Koskela, K. Lakkala, R. Müller, C. L. Myhre and M. Rex, Ozone and UV radiation, in: State of the Climate in 2011, Bull. Am. Meteorol. Soc., 2012, 93, S129–S132 Search PubMed.
- D. Balis, I. S. A. Isaksen, C. Zerefos, I. Zyrichidou, K. Eleftheratos, K. Tourpali, R. Bojkov, B. Rognerud, F. Stordal, O. A. Søvde and Y. Orsolini, Observed and modelled record ozone decline over the Arctic during winter/spring 2011, Geophys. Res. Lett., 2011, 38, L23801 CrossRef.
- I. S. A. Isaksen, C. Zerefos, W. C. Wang, D. Balis, K. Eleftheratos, B. Rognerud, F. Stordal, T. K. Berntsen, J. H. LaCasce, O. A. Søvde, D. Olivié, Y. J. Orsolini, I. Zyrichidou, M. Prather and O. N. E. Tuinder, Attribution of the Arctic ozone column deficit in March 2011, Geophys. Res. Lett., 2012, 39, L24810 CrossRef.
- R. R. Garcia, Atmospheric science: An Arctic ozone hole?, Nature, 2011, 478, 462–463 CrossRef CAS PubMed.
- B. Petkov, V. Vitale, C. Tomasi, A. M. Siani, G. Seckmeyer, A. Webb, A. R. D. Smedley, G. R. Casale, R. Werner, C. Lanconelli, M. Mazzola, A. Lupi, M. Busetto, H. Diémoz, F. Goutail, U. Köhler, B. T. Mendeva, W. Josefsson, D. Moore, M. L. Bartolomé, J. R. Moreta González, O. Mišaga, A. Dahlback, Z. Tóth, S. Varghese, H. De Backer, R. Stübi and K. Vaníček, Response of the ozone column over Europe to the 2011 Arctic ozone depletion event according to ground-based observations and assessment of the consequent variations in surface UV irradiance, Atmos. Environ., 2014, 85, 169–178 CrossRef CAS PubMed.
- B. M. Sinnhuber, G. Stiller, R. Ruhnke, T. von Clarmann, S. Kellmann and J. Aschmann, Arctic winter 2010/2011 at the brink of an ozone hole, Geophys. Res. Lett., 2011, 38, L24814 CrossRef.
-
D. L. Hartmann, A. M. G. K. Tank, M. Rusticucci, L. V. Alexander, S. Brönnimann, Y. Charabi, F. J. Dentener, E. J. Dlugokencky, D. R. Easterling, A. Kaplan, B. J. Soden, P. W. Thorne, M. Wild and P. M. Zhai, Observations: Atmosphere and Surface, in Climate Change 2013: The Physical Science Basis. Contribution of Working Group I to the Fifth Assessment Report of the Intergovernmental Panel on Climate Change, ed. T. F. Stocker, D. Qin, G.-K. Plattner, M. Tignor, S. K. Allen, J. Boschung, A. Nauels, Y. Xia and V. Bex and P. M. Midgley, Cambridge University Press, Cambridge, United Kingdom and New York, NY, U.S.A., 2013 Search PubMed.
- D. W. J. Thompson, D. J. Seidel, W. J. Randel, C.-Z. Zou, A. H. Butler, C. Mears, A. Osso, C. Long and R. Lin, The mystery of recent stratospheric temperature trends, Nature, 2012, 491, 692–697 CrossRef CAS PubMed.
- D. J. Seidel, N. P. Gillett, J. R. Lanzante, K. P. Shine and P. W. Thorne, Stratospheric temperature trends: our evolving understanding, Wiley Interdiscip. Rev. Climate Change, 2011, 2, 592–616 CrossRef.
- J. F. Bornman, P. W. Barnes, S. A. Robinson, C. L. Ballaré, S. D. Flint and M. M. Caldwell, Solar ultraviolet radiation and ozone depletion-driven climate change: effects on terrestrial ecosystems, Photochem. Photobiol. Sci., 2015, 14 10.1039/c4pp90034k , this issue.
- D. J. Erickson III, B. Sulzberger, R. Zepp, A. T. Austin and N. Paul, Effects of solar UV radiation and climate change on biogeochemical cycling: Interactions and feedbacks, Photochem. Photobiol. Sci., 2015, 14 10.1039/c4pp90036g , this issue.
- S. M. Kang, L. M. Polvani, J. C. Fyfe, S. W. Son, M. Sigmond and G. J. P. Correa, Modeling evidence that ozone depletion has impacted extreme precipitation in the austral summer, Geophys. Res. Lett., 2013, 40, 4054–4059 CrossRef.
- S. M. Kang, L. M. Polvani, J. C. Fyfe and M. Sigmond, Impact of polar ozone depletion on subtropical precipitation, Science, 2011, 332, 951–954 CrossRef CAS PubMed.
- R. D. Hudson, Measurements of the movement of the jet streams at mid-latitudes, in the Northern and Southern Hemispheres, 1979 to 2010, Atmos. Chem. Phys., 2012, 12, 7797–7808 CrossRef CAS.
- S.-W. Son, E. P. Gerber, J. Perlwitz, L. M. Polvani, N. Gillett, K.-H. Seo, V. Eyring, T. G. Shepherd, D. Waugh, H. Akiyoshi, J. Austin, A. Baumgaertner, S. Bekki, P. Braesicke, C. Brühl, N. Butchart, M. Chipperfield, D. Cugnet, M. Dameris, S. Dhomse, S. Frith, H. Garny, R. Garcia, S. C. Hardiman, P. Jöckel, J.-F. Lamarque, E. Mancini, M. Marchand, M. Michou, T. Nakamura, O. Morgenstern, G. Pitari, D. A. Plummer, J. Pyle, E. Rozanov, J. F. Scinocca, K. Shibata, D. Smale, H. Teyssèdre, W. Tian and Y. Yamashita, The impact of stratospheric ozone on the Southern Hemisphere circulation change: A multimodel assessment, J. Geophys. Res., [Atmos.], 2010, 115, D014271 CrossRef.
- D. W. Waugh, F. Primeau, T. DeVries and M. Holzer, Recent Changes in the Ventilation of the Southern Oceans, Science, 2013, 339, 568–570 CrossRef CAS PubMed.
- S. A. Robinson and D. J. Erickson III, Not just about sunburn – the ozone hole's profound effect on climate has significant implications for Southern Hemisphere ecosystems, Global Change Biol., 2014 DOI:10.1111/gcb.12739.
- J. M. Arblaster, G. A. Meehl and D. J. Karoly, Future climate change in the Southern Hemisphere: Competing effects of ozone and greenhouse gases, Geophys. Res. Lett., 2011, 38, L02701 CrossRef.
- A. Y. Karpechko, N. P. Gillett, L. J. Gray and M. Dall'Amico, Influence of ozone recovery and greenhouse gas increases on Southern Hemisphere circulation, J. Geophys. Res., [Atmos.], 2010, 115, D22117 CrossRef.
- L. M. Polvani, M. Previdi and C. Deser, Large cancellation, due to ozone recovery, of future Southern Hemisphere atmospheric circulation trends, Geophys. Res. Lett., 2011, 38, L04707 CrossRef.
- M. Sigmond, M. C. Reader, J. C. Fyfe and N. P. Gillett, Drivers of past and future Southern Ocean change: Stratospheric ozone versus greenhouse gas impacts, Geophys. Res. Lett., 2011, 38, L12601 CrossRef.
- K. L. Smith, L. M. Polvani and D. R. Marsh, Mitigation of 21st century Antarctic sea ice loss by stratospheric ozone recovery, Geophys. Res. Lett., 2012, 39, L20701 Search PubMed.
- C. McLandress, T. G. Shepherd, J. F. Scinocca, D. A. Plummer, M. Sigmond, A. I. Jonsson and M. C. Reader, Separating the Dynamical Effects of Climate Change and Ozone Depletion. Part II: Southern Hemisphere Troposphere, J. Clim., 2011, 24, 1850–1868 CrossRef.
-
IPCC, (Intergovernmental Panel on Climate Change): Working Group I Contribution to the IPCC Fifth Assessment Report Climate Change 2013: The Physical Science Basis Cambridge University Press, Cambridge, United Kingdom and New York, NY, U.S.A., Cambridge, UK, and New York, NY, 2013.
- M. Wang and J. E. Overland, A sea ice free summer Arctic within 30 years: An update from CMIP5 models, Geophys. Res. Lett., 2012, 39, L18501 Search PubMed.
- U. Langematz, S. Meul, K. Grunow, E. Romanowsky, S. Oberländer, J. Abalichin and A. Kubin, Future arctic temperature and ozone: The role of stratospheric composition changes, J. Geophys. Res., [Atmos.], 2014, 119, 2092–2112 CAS.
- R. W. Portmann, J. S. Daniel and A. R. Ravishankara, Stratospheric ozone depletion due to nitrous oxide: influences of other gases, Philos. Trans. R. Soc. London., Ser B, 2012, 367, 1256–1264 CrossRef CAS PubMed.
- R. Lei, Z. Zhang, I. Matero, B. Cheng, Q. Li and W. Huang, Reflection and transmission of irradiance by snow and sea ice in the central Arctic Ocean in summer 2010, Polar Res., 2012, 31, 17325 Search PubMed.
- J. C. Comiso, C. L. Parkinson, R. Gersten and L. Stock, Accelerated decline in the Arctic sea ice cover, Geophys. Res. Lett., 2008, 35, L01703 CrossRef.
- C. L. Parkinson and D. J. Cavalieri, Antarctic sea ice variability and trends, 1979–2010, Cryosphere, 2012, 6, 871–880 CrossRef PubMed.
- R. Kwok and D. A. Rothrock, Decline in Arctic sea ice thickness from submarine and ICESat records: 1958–2008, Geophys. Res. Lett., 2009, 36, L15501 CrossRef.
- T. Markus, J. C. Stroeve and J. Miller, Recent changes in Arctic sea ice melt onset, freezeup, and melt season length, J. Geophys. Res.: Oceans, 2009, 114, C12024 CrossRef.
- D. K. Perovich and C. Polashenski, Albedo evolution of seasonal Arctic sea ice, Geophys. Res. Lett., 2012, 39, L08501 CrossRef.
- J. Blunden and D. S. Arndt, State of the Climate in 2013, Bull. Am. Meteorol. Soc., 2014, 95, S1–S257 Search PubMed.
- S. Madronich, M. Shao, S. R. Wilson, K. R. Solomon, J. Longstrethe and X. Tang, Changes in air quality and tropospheric composition due to depletion of stratospheric ozone and interactions with changing climate: Implications for human and environmental health, Photochem. Photobiol. Sci., 2015, 14 10.1039/c4pp90037e , this issue.
-
IPCC, (Intergovernmental Panel on Climate Change): Climate Change 2007: The Physical Science Basis. Contribution of Working Group I to the Fourth Assessment Report of the Intergovernmental Panel on Climate Change, Cambridge University Press, Cambridge, United Kingdom and New York, NY, USA, Cambridge, UK, and New York, NY, 2007.
-
NARSTO, Particulate Matter Science for Policy Makers: A NARSTO Assessment, Cambridge, England, 2004 Search PubMed.
- Y. Chen and S. Xie, Temporal and spatial visibility trends in the Sichuan Basin, China, 1973 to 2010, Atmos. Res., 2012, 112, 25–34 CrossRef PubMed.
- J. Wu, X. Zhang, J. Hu and D. Zhao, Probability of different visibility grades in China over a 50-year period, Meteorol. Atmos. Phys., 2013, 122, 115–123 CrossRef PubMed.
- S. Sabetghadam, F. Ahmadi-Givi and Y. Golestani, Visibility trends in Tehran during 1958–2008, Atmos. Environ., 2012, 62, 512–520 CrossRef CAS PubMed.
- K. Wang, R. E. Dickinson and S. Liang, Clear Sky Visibility Has Decreased over Land Globally from 1973 to 2007, Science, 2009, 323, 1468–1470 CrossRef CAS PubMed.
- M. Doyle and S. Dorling, Visibility trends in the UK 1950–1997, Atmos. Environ., 2002, 36, 3161–3172 CrossRef CAS.
- C. Ruckstuhl, R. Philipona, K. Behrens, M. Collaud Coen, B. Dürr, A. Heimo, C. Mätzler, S. Nyeki, A. Ohmura, L. Vuilleumier, M. Weller, C. Wehrli and A. Zelenka, Aerosol and cloud effects on solar brightening and the recent rapid warming, Geophys. Res. Lett., 2008, 35, L12708 CrossRef.
- J. L. Jimenez, M. R. Canagaratna, N. M. Donahue, A. S. H. Prevot, Q. Zhang, J. H. Kroll, P. F. DeCarlo, J. D. Allan, H. Coe, N. L. Ng, A. C. Aiken, K. S. Docherty, I. M. Ulbrich, A. P. Grieshop, A. L. Robinson, J. Duplissy, J. D. Smith, K. R. Wilson, V. A. Lanz, C. Hueglin, Y. L. Sun, J. Tian, A. Laaksonen, T. Raatikainen, J. Rautiainen, P. Vaattovaara, M. Ehn, M. Kulmala, J. M. Tomlinson, D. R. Collins, M. J. Cubison, E. J. Dunlea, J. A. Huffman, T. B. Onasch, M. R. Alfarra, P. I. Williams, K. Bower, Y. Kondo, J. Schneider, F. Drewnick, S. Borrmann, S. Weimer, K. Demerjian, D. Salcedo, L. Cottrell, R. Griffin, A. Takami, T. Miyoshi, S. Hatakeyama, A. Shimono, J. Y. Sun, Y. M. Zhang, K. Dzepina, J. R. Kimmel, D. Sueper, J. T. Jayne, S. C. Herndon, A. M. Trimborn, L. R. Williams, E. C. Wood, A. M. Middlebrook, C. E. Kolb, U. Baltensperger and D. R. Worsnop, Evolution of organic aerosols in the atmosphere, Science, 2009, 326, 1525–1529 CrossRef CAS PubMed.
- A. Laskin, J. Laskin and S. A. Nizkorodov, Mass spectrometric approaches for chemical characterisation of atmospheric aerosols: critical review of the most recent advances, Environ. Chem., 2012, 9, 163–189 CrossRef CAS.
- M. Collaud Coen, E. Andrews, A. Asmi, U. Baltensperger, N. Bukowiecki, D. Day, M. Fiebig, A. M. Fjaeraa, H. Flentje, A. Hyvärinen, A. Jefferson, S. G. Jennings, G. Kouvarakis, H. Lihavainen, C. Lund Myhre, W. C. Malm, N. Mihapopoulos, J. V. Molenar, C. O'Dowd, J. A. Ogren, B. A. Schichtel, P. Sheridan, A. Virkkula, E. Weingartner, R. Weller and P. Laj, Aerosol decadal trends – Part 1: In situ optical measurements at GAW and IMPROVE stations, Atmos. Chem. Phys., 2013, 13, 869–894 Search PubMed.
- A. Cazorla, R. Bahadur, K. J. Suski, J. F. Cahill, D. Chand, B. Schmid, V. Ramanathan and K. A. Prather, Relating aerosol absorption due to soot, organic carbon, and dust to emission sources determined from in situ chemical measurements, Atmos. Chem. Phys., 2013, 13, 9337–9350 CrossRef CAS.
- T. F. Eck, B. N. Holben, A. Sinyuk, R. T. Pinker, P. Goloub, H. Chen, B. Chatenet, Z. Li, R. P. Singh, S. N. Tripathi, J. S. Reid, D. M. Giles, O. Dubovik, N. T. O'Neill, A. Smirnov, P. Wang and X. Xia, Climatological aspects of the optical properties of fine/coarse mode aerosol mixtures, J. Geophys. Res., [Atmos.], 2010, 115, D19205 CrossRef.
- D. M. Giles, B. N. Holben, T. F. Eck, A. Sinyuk, A. Smirnov, I. Slutsker, R. R. Dickerson, A. M. Thompson and J. S. Schafer, An analysis of AERONET aerosol absorption properties and classifications representative of aerosol source regions, J. Geophys. Res., [Atmos.], 2012, 117, D17203 CrossRef.
- R. A. Kahn, D. L. Nelson, M. J. Garay, R. C. Levy, M. A. Bull, D. J. Diner, J. V. Martonchik, S. R. Paradise, E. G. Hansen and L. A. Remer, MISR aerosol product attributes and statistical comparisons with MODIS, Geosci. Remote Sens. IEEE Transact., 2009, 47, 4095–4114 CrossRef.
- A. H. Omar, D. M. Winker, M. A. Vaughan, Y. Hu, C. R. Trepte, R. A. Ferrare, K.-P. Lee, C. A. Hostetler, C. Kittaka, R. R. Rogers, R. E. Kuehn and Z. Liu, The CALIPSO Automated Aerosol Classification and Lidar Ratio Selection Algorithm, J. Atmos. Ocean. Technol., 2009, 26, 1994–2014 CrossRef.
- L. A. Remer, S. Mattoo, R. C. Levy and L. A. Munchak, MODIS 3 km aerosol product: algorithm and global perspective, Atmos. Meas. Tech., 2013, 6, 1829–1844 CrossRef.
- J. Zhang and J. S. Reid, A decadal regional and global trend analysis of the aerosol optical depth using a data-assimilation grade over-water MODIS and Level 2 MISR aerosol products, Atmos. Chem. Phys., 2010, 10, 10949–10963 CrossRef CAS.
- S. Kinne, D. O'Donnel, P. Stier, S. Kloster, K. Zhang, H. Schmidt, S. Rast, M. Giorgetta, T. F. Eck and B. Stevens, MAC-v1: A new global aerosol climatology for climate studies, J. Adv. Model. Earth Syst., 2013, 5, 704–740 CrossRef.
- V. E. Cachorro, A. Berjón, C. Toledano, S. Mogo, N. Prats, A. M. de Frutos, J. M. Vilaplana, M. Sorribas, B. A. De La Morena, J. Gröbner and N. Laulainen, Detailed Aerosol Optical Depth Intercomparison between Brewer and Li-Cor 1800 Spectroradiometers and a Cimel Sun Photometer, J. Atmos. Ocean. Technol., 2009, 26, 1558–1571 CrossRef.
- E. Gerasopoulos, V. Amiridis, S. Kazadzis, P. Kokkalis, K. Eleftheratos, M. O. Andreae, T. W. Andreae, H. El-Askary and C. S. Zerefos, Three-year ground based measurements of aerosol optical depth over the Eastern Mediterranean: the urban environment of Athens, Atmos. Chem. Phys., 2011, 11, 2145–2159 CrossRef CAS.
- W. Kumharn, J. S. Rimmer, A. R. D. Smedley, T. Y. Ying and A. R. Webb, Aerosol optical depth and the global Brewer network: A study using U.K.- and Malaysia-based Brewer spectrophotometers, J. Atmos. Ocean. Technol., 2012, 29, 857–866 CrossRef.
- J. B. Liley and B. W. Forgan, Aerosol optical depth over Lauder, New Zealand, Geophys. Res. Lett., 2009, 36, L07811 CrossRef.
- R. L. McKenzie, C. Weinreis, P. V. Johnston, B. Liley, H. Shiona, M. Kotkamp, D. Smale, N. Takegawa and Y. Kondo, Effects of urban pollution on UV spectral irradiances, Atmos. Chem. Phys., 2008, 8, 5683–5697 CrossRef CAS.
- G. G. Palancar, B. L. Lefer, S. R. Hall, W. J. Shaw, C. A. Corr, S. C. Herndon, J. R. Slusser and S. Madronich, Effect of aerosols and NO2 concentration on ultraviolet actinic flux near Mexico City during MILAGRO: measurements and model calculations, Atmos. Chem. Phys., 2013, 13, 1011–1022 CrossRef.
- N. Chubarova, Y. Nezval, I. Sviridenkov, A. Smirnov and I. Slutsker, Smoke aerosol and its radiative effects during extreme fire event over Central Russia in summer 2010, Atmos. Meas. Tech., 2012, 5, 557–568 CrossRef PubMed.
- R. R. Dickerson, S. K. Ondragunta, G. Stenchikov, K. L. Civerolo, B. G. Doddridge and B. N. Holben, The impact of aerosols on solar ultraviolet radiation and photochemical smog, Science, 1997, 278, 827–830 CrossRef CAS.
- Y. Feng, V. Ramanathan and V. R. Kotamarthi, Brown carbon: a significant atmospheric absorber of solar radiation?, Atmos. Chem. Phys., 2013, 13, 8607–8621 CrossRef CAS.
- A. Hoffer, A. Gelencsér, P. Guyon, G. Kiss, O. Schmid, G. P. Frank, P. Artaxo and M. O. Andreae, Optical properties of humic-like substances (HULIS) in biomass-burning aerosols, Atmos. Chem. Phys., 2006, 6, 3563–3570 CrossRef CAS.
- T. W. Kirchstetter and T. L. Thatcher, Contribution of organic carbon to wood smoke particulate matter absorption of solar radiation, Atmos. Chem. Phys., 2012, 12, 6067–6072 CrossRef CAS.
- S. Kazadzis, N. Kouremeti, A. Bais, A. Kazantzidis and C. Meleti, Aerosol forcing efficiency in the UVA region from spectral solar irradiance measurements at an urban environment, Ann. Geophys., 2009, 27, 2515–2522 CrossRef PubMed.
- C. A. Corr, S. R. Hall, K. Ullmann, B. E. Anderson, A. J. Beyersdorf, K. L. Thornhill, M. J. Cubison, J. L. Jimenez, A. Wisthaler and J. E. Dibb, Spectral absorption of biomass burning aerosol determined from retrieved single scattering albedo during ARCTAS, Atmos. Chem. Phys., 2012, 12, 10505–10518 CrossRef CAS.
- B. N. Wenny, J. S. Schafer, J. J. DeLuisi, V. K. Saxena, W. F. Barnard, I. V. Petropavlovskikh and A. J. Vergamini, A study of regional aerosol radiative properties and effects on ultraviolet-B radiation, J. Geophys. Res., [Atmos.], 1998, 103, 17083–17097 CrossRef CAS.
- J. L. Petters, V. K. Saxena, J. R. Slusser, B. N. Wenny and S. Madronich, Aerosol single scattering albedo retrieved from measurements of surface UV irradiance and a radiative transfer model, J. Geophys. Res., [Atmos.], 2003, 108, 4288 CrossRef.
- M. A. Wetzel, G. E. Shaw, J. R. Slusser, R. D. Borys and C. F. Cahill, Physical, chemical, and ultraviolet radiative characteristics of aerosol in central Alaska, J. Geophys. Res., [Atmos.], 2003, 108, 4418 CrossRef.
- C. D. Goering, T. S. L'Ecuyer, G. L. Stephens, J. R. Slusser, G. Scott, J. Davis, J. C. Barnard and S. Madronich, Simultaneous retrievals of column ozone and aerosol optical properties from direct and diffuse solar irradiance measurements, J. Geophys. Res., [Atmos.], 2005, 110, D05204 CrossRef.
- A. F. Bais, A. Kazantzidis, S. Kazadzis, D. S. Balis, C. S. Zerefos and C. Meleti, Deriving an effective aerosol single scattering albedo from spectral surface UV irradiance measurements, Atmos. Environ., 2005, 39, 1093–1102 CrossRef CAS PubMed.
- J. C. Barnard, R. Volkamer and E. I. Kassianov, Estimation of the mass absorption cross section of the organic carbon component of aerosols in the Mexico City Metropolitan Area, Atmos. Chem. Phys., 2008, 8, 6665–6679 CrossRef CAS.
- C. A. Corr, N. Krotkov, S. Madronich, J. R. Slusser, B. Holben, W. Gao, J. Flynn, B. Lefer and S. M. Kreidenweis, Retrieval of aerosol single scattering albedo at ultraviolet wavelengths at the T1 site during MILAGRO, Atmos. Chem. Phys., 2009, 9, 5813–5827 CrossRef CAS.
- I. Ialongo, V. Buchard, C. Brogniez, G. R. Casale and A. M. Siani, Aerosol single scattering albedo retrieval in the UV range: an application to OMI satellite validation, Atmos. Chem. Phys., 2010, 10, 331–340 CrossRef CAS.
- M. Gyawali, W. P. Arnott, R. A. Zaveri, C. Song, H. Moosmüller, L. Liu, M. I. Mishchenko, L. W. A. Chen, M. C. Green, J. G. Watson and J. C. Chow, Photoacoustic optical properties at UV, VIS, and near IR wavelengths for laboratory generated and winter time ambient urban aerosols, Atmos. Chem. Phys., 2012, 12, 2587–2601 CrossRef CAS.
- V. Buchard, C. Brogniez, F. Auriol and B. Bonnel, Aerosol single scattering albedo retrieved from ground-based measurements in the UV and visible region, Atmos. Meas. Tech., 2011, 4, 1–7 CrossRef PubMed.
- R. Medina, R. M. Fitzgerald and Q. Min, Retrieval of the single scattering albedo in the El Paso-Juarez Airshed using the TUV model and a UV-MFRSR radiometer, Atmos. Environ., 2012, 46, 430–440 CrossRef CAS PubMed.
- Q. Zhang, J. L. Jimenez, M. R. Canagaratna, J. D. Allan, H. Coe, I. Ulbrich, M. R. Alfarra, A. Takami, A. M. Middlebrook, Y. L. Sun, K. Dzepina, E. Dunlea, K. Docherty, P. F. DeCarlo, D. Salcedo, T. Onasch, J. T. Jayne, T. Miyoshi, A. Shimono, S. Hatakeyama, N. Takegawa, Y. Kondo, J. Schneider, F. Drewnick, S. Borrmann, S. Weimer, K. Demerjian, P. Williams, K. Bower, R. Bahreini, L. Cottrell, R. J. Griffin, J. Rautiainen, J. Y. Sun and Y. M. Zhang, Ubiquity and dominance of oxygenated species in organic aerosols in anthropogenically-influenced Northern Hemisphere midlatitudes, Geophys. Res. Lett., 2007, 34, L13801 Search PubMed.
- C. L. Heald, H. Coe, J. L. Jimenez, R. J. Weber, R. Bahreini, A. M. Middlebrook, L. M. Russell, M. Jolleys, T. M. Fu, J. D. Allan, K. N. Bower, G. Capes, J. Crosier, W. T. Morgan, N. H. Robinson, P. I. Williams, M. J. Cubison, P. F. DeCarlo and E. J. Dunlea, Exploring the vertical profileof atmospheric organic aerosol: comparing 17 aircraft field campaigns with a global model, Atmos. Chem. Phys., 2011, 11, 12673–12696 CAS.
- D. L. Bones, D. K. Henricksen, S. A. Mang, M. Gonsior, A. P. Bateman, T. B. Nguyen, W. J. Cooper and S. A. Nizkorodov, Appearance of strong absorbers and fluorophores in limonene-O3 secondary
organic aerosol due to NH4+-mediated chemical aging over long time scales, J. Geophys. Res., [Atmos.], 2010, 115, D05203 CrossRef.
- X. Zhang, Y.-H. Lin, J. D. Surratt, P. Zotter, A. S. H. Prévôt and R. J. Weber, Light-absorbing soluble organic aerosol in Los Angeles and Atlanta: A contrast in secondary organic aerosol, Geophys. Res. Lett., 2011, 38, L21810 Search PubMed.
- Y. Desyaterik, Y. Sun, X. Shen, T. Lee, X. Wang, T. Wang and J. L. Collett, Speciation of “brown” carbon in cloud water impacted by agricultural biomass burning in eastern China, J. Geophys. Res., [Atmos.], 2013, 118, 7389–7399 CAS.
- S. A. Epstein, E. Tapavicza, F. Furche and S. A. Nizkorodov, Direct photolysis of carbonyl compounds dissolved in cloud and fog-droplets, Atmos. Chem. Phys., 2013, 13, 9461–9477 Search PubMed.
- A. T. Lambe, C. D. Cappa, P. Massoli, T. B. Onasch, S. D. Forestieri, A. T. Martin, M. J. Cummings, D. R. Croasdale, W. H. Brune, D. R. Worsnop and P. Davidovits, Relationship between Oxidation Level and Optical Properties of Secondary Organic Aerosol, Environ. Sci. Technol., 2013, 47, 6349–6357 CAS.
- K. M. Updyke, T. B. Nguyen and S. A. Nizkorodov, Formation of brown carbon via reactions of ammonia with secondary organic aerosols from biogenic and anthropogenic precursors, Atmos. Environ., 2012, 63, 22–31 CrossRef CAS PubMed.
- A. G. Rincón, M. I. Guzmán, M. R. Hoffmann and A. J. Colussi, Thermochromism of Model Organic Aerosol Matter, J. Phys. Chem. Lett., 2009, 1, 368–373 CrossRef.
- D. G. Streets, F. Yan, M. Chin, T. Diehl, N. Mahowald, M. Schultz, M. Wild, Y. Wu and C. Yu, Anthropogenic and natural contributions to regional trends in aerosol optical depth, 1980–2006, J. Geophys. Res., [Atmos.], 2009, 114, D00D18 CrossRef.
- M. Chin, T. Diehl, Q. Tan, J. M. Prospero, R. A. Kahn, L. A. Remer, H. Yu, A. M. Sayer, H. Bian, I. V. Geogdzhayev, B. N. Holben, S. G. Howell, B. J. Huebert, N. C. Hsu, D. Kim, T. L. Kucsera, R. C. Levy, M. I. Mishchenko, X. Pan, P. K. Quinn, G. L. Schuster, D. G. Streets, S. A. Strode, O. Torres and X. P. Zhao, Multi-decadal aerosol variations from 1980 to 2009: a perspective from observations and a global model, Atmos. Chem. Phys., 2014, 14, 3657–3690 Search PubMed.
- P. N. den Outer, H. Slaper, J. Kaurola, A. Lindfors, A. Kazantzidis, A. F. Bais, U. Feister, J. Junk, M. Janouch and W. Josefsson, Reconstructing of erythemal ultraviolet radiation levels in Europe for the past 4 decades, J. Geophys. Res., [Atmos.], 2010, 115, 10102 CrossRef.
- C. S. Zerefos, K. Eleftheratos, C. Meleti, S. Kazadzis, A. Romanou, C. Ichoku, G. Tselioudis and A. Bais, Solar dimming and brightening over Thessaloniki, Greece, and Beijing, China, Tellus Ser. B, 2009, 61, 657–665 CrossRef PubMed.
- C. Meleti, A. F. Bais, S. Kazadzis, N. Kouremeti, K. Garane and C. Zerefos, Factors affecting solar ultraviolet irradiance measuredsince 1990 at Thessaloniki, Greece, Int. J. Remote Sens., 2009, 30, 4167–4179 CrossRef.
- J. Yoon, W. von Hoyningen-Huene, M. Vountas and J. P. Burrows, Analysis of linear long-term trend of aerosol optical thickness derived from SeaWiFS using BAER over Europe and South China, Atmos. Chem. Phys., 2011, 11, 12149–12167 CrossRef CAS.
- Z. B. Wang, M. Hu, Z. J. Wu, D. L. Yue, L. Y. He, X. F. Huang, X. G. Liu and A. Wiedensohler, Long-term measurements of particle number size distributions and the relationships with air mass history and source apportionment in the summer of Beijing, Atmos. Chem. Phys., 2013, 13, 10159–10170 CrossRef CAS.
- J. F. Lamarque, T. C. Bond, V. Eyring, C. Granier, A. Heil, Z. Klimont, D. Lee, C. Liousse, A. Mieville, B. Owen, M. G. Schultz, D. Shindell, S. J. Smith, E. Stehfest, J. Van Aardenne, O. R. Cooper, M. Kainuma, N. Mahowald, J. R. McConnell, V. Naik, K. Riahi and D. P. van Vuuren, Historical (1850–2000) gridded anthropogenic and biomass burning emissions of reactive gases and aerosols: methodology and application, Atmos. Chem. Phys., 2010, 10, 7017–7039 CrossRef CAS.
- S. J. Smith and T. C. Bond, Two hundred fifty years of aerosols and climate: the end of the age of aerosols, Atmos. Chem. Phys., 2014, 14, 537–549 CAS.
- N. Bellouin, J. Rae, A. Jones, C. Johnson, J. Haywood and O. Boucher, Aerosol forcing in the Climate Model Intercomparison Project (CMIP5) simulations by HadGEM2-ES and the role of ammonium nitrate, J. Geophys. Res., [Atmos.], 2011, 116, D20206 CrossRef.
- A. de Miguel, R. Román, J. Bilbao and D. Mateos, Evolution of erythemal and total shortwave solar radiation in Valladolid, Spain: Effects of atmospheric factors, J. Atmos. Sol.–Terr. Phys., 2011, 73, 578–586 CrossRef PubMed.
- A. A. Silva, Erythemal dose rate under noon overcast skies, Photochem. Photobiol. Sci., 2013, 12, 777–786 CAS.
- A. Kazantzidis, K. Eleftheratos and C. S. Zerefos, Effects of cirrus cloudiness on solar irradiance in four spectral bands, Atmos. Res., 2011, 102, 452–459 CrossRef PubMed.
- Y. Liu, J. R. Key, Z. Liu, X. Wang and S. J. Vavrus, A cloudier Arctic expected with diminishing sea ice, Geophys. Res. Lett., 2012, 39, L05705 Search PubMed.
- M. Antón, L. Alados-Arboledas, J. L. Guerrero-Rascado, M. J. Costa, J. C. Chiu and F. J. Olmo, Experimental and modeled UV erythemal irradiance under overcast conditions: the role of cloud optical depth, Atmos. Chem. Phys., 2012, 12, 11723–11732 CrossRef.
-
UNEP, Environmental Effects of Ozone Depletion and its Interaction with Climate Change: 2010 Assessment, United Nations Environment Programme (UNEP), Nairobi, 2010 Search PubMed.
-
A. Kreuter, M. Blumthaler, A. R. Webb, A. F. Bais, R. Kift and N. Kouremeti, Effects of Albedo on Solar Irradiance, in Advances in Meteorology, Climatology and Atmospheric Physics, Springer, Berlin, Heidelberg, Germany, 2013, pp. 1089–1095 Search PubMed.
- O. Meinander, S. Kazadzis, A. Arola, A. Riihelä, P. Räisänen, R. Kivi, A. Kontu, R. Kouznetsov, M. Sofiev, J. Svensson, H. Suokanerva, V. Aaltonen, T. Manninen, J. L. Roujean and O. Hautecoeur, Spectral albedo of seasonal snow during intensive melt period at Sodankylä, beyond the Arctic Circle, Atmos. Chem. Phys., 2013, 13, 3793–3810 CrossRef CAS.
- S. Simic, M. Fitzka, A. Schmalwieser, P. Weihs and J. Hadzimustafic, Factors affecting UV irradiance at selected wavelengths at Hoher Sonnblick, Atmos. Res., 2011, 101, 869–878 CrossRef PubMed.
- P. J. Hezel, X. Zhang, C. M. Bitz, B. P. Kelly and F. Massonnet, Projected decline in spring snow depth on Arctic sea ice caused by progressively later autumn open ocean freeze-up this century, Geophys. Res. Lett., 2012, 39, L17505 CrossRef.
- K. E. Frey, D. K. Perovich and B. Light, The spatial distribution
of solar radiation under a melting Arctic sea ice cover, Geophys. Res. Lett., 2011, 38, L22501 CrossRef.
- E. J. Steig, Q. Ding, D. S. Battisti and A. Jenkins, Tropical forcing of Circumpolar Deep Water Inflow and outlet glacier thinning in the Amundsen Sea Embayment, West Antarctica, Ann. Glaciol., 2012, 53, 19–28 CrossRef.
- E. Rignot, J. Mouginot, M. Morlighem, H. Seroussi and B. Scheuchl, Widespread, rapid grounding line retreat of Pine Island, Thwaites, Smith, and Kohler glaciers, West Antarctica, from 1992 to 2011, Geophys. Res. Lett., 2014, 41, 3502–3509 CrossRef.
- I. Joughin, B. E. Smith and B. Medley, Marine ice sheet collapse potentially under way for the Thwaites Glacier Basin, West Antarctica, Science, 2014, 344, 735–738 CrossRef CAS PubMed.
- L. L. Hood and B. E. Soukharev, The Lower-Stratospheric Response to 11-Yr Solar Forcing: Coupling to the Troposphere–Ocean Response, J. Atmos. Sci., 2012, 69, 1841–1864 CrossRef.
- M. Lockwood, C. Bell, T. Woollings, R. G. Harrison, L. J. Gray and J. D. Haigh, Top-down solar modulation of climate: evidence for centennial-scale change, Environ. Res. Lett., 2010, 5, 034008 CrossRef.
- J. W. Harder, J. M. Fontenla, P. Pilewskie, E. C. Richard and T. N. Woods, Trends in solar spectral irradiance variability in the visible and infrared, Geophys. Res. Lett., 2009, 36, L07801 CrossRef.
- J. D. Haigh, A. R. Winning, R. Toumi and J. W. Harder, An influence of solar spectral variations on radiative forcing of climate, Nature, 2010, 467, 696–699 CrossRef CAS PubMed.
- I. Ermolli, K. Matthes, T. Dudok de Wit, N. A. Krivova, K. Tourpali, M. Weber, Y. C. Unruh, L. Gray, U. Langematz, P. Pilewskie, E. Rozanov, W. Schmutz, A. Shapiro, S. K. Solanki and T. N. Woods, Recent variability of the solar spectral irradiance and its impact on climate modelling, Atmos. Chem. Phys., 2013, 13, 3945–3977 CrossRef CAS.
- W. H. Swartz, R. S. Stolarski, L. D. Oman, E. L. Fleming and C. H. Jackman, Middle atmosphere response to different descriptions of the 11-yr solar cycle in spectral irradiance in a chemistry-climate model, Atmos. Chem. Phys., 2012, 12, 5937–5948 CrossRef CAS.
- M. Lockwood, M. J. Owens, L. Barnard, C. J. Davis and F. Steinhilber, The persistence of solar activity indicators and the descent of the Sun into Maunder Minimum conditions, Geophys. Res. Lett., 2011, 38, L22105 CrossRef.
- M. Lockwood, A. P. Rouillard and I. D. Finch, The rise and fall of open solar flux during the current grand solar maximum, Astrophys. J., 2009, 700, 937 CrossRef.
- F. Steinhilber and J. Beer, Prediction of solar activity for the next 500 years, J. Geophys. Res.: Space Phys., 2013, 118, 1861–1867 Search PubMed.
- M. J. Owens, M. Lockwood, L. Barnard and C. J. Davis, Solar cycle 24: Implications for energetic particles and long-term space climate change, Geophys. Res. Lett., 2011, 38, L19106 CrossRef.
- J. A. Abreu, J. Beer, F. Steinhilber, S. M. Tobias and N. O. Weiss, For how long will the current grand maximum of solar activity persist?, Geophys. Res. Lett., 2008, 35, L20109 CrossRef.
- J. G. Anet, E. V. Rozanov, S. Muthers, T. Peter, S. Brönnimann, F. Arfeuille, J. Beer, A. I. Shapiro, C. C. Raible, F. Steinhilber and W. K. Schmutz, Impact of a potential 21st century “grand solar minimum” on surface temperatures and stratospheric ozone, Geophys. Res. Lett., 2013, 40, 4420–4425 CrossRef CAS.
- J. E. Frederick and A. L. Hodge, Solar irradiance at the earth's surface: long-term behavior observed at the South Pole, Atmos. Chem. Phys., 2011, 11, 1177–1189 CrossRef CAS.
- D. S. Berger and F. Urbach, A climatology of sunburning ultraviolet radiation, Photochem. Photobiol., 1982, 35, 187–192 CrossRef CAS PubMed.
- N. Y. Chubarova and Y. I. Nezval, Thirty year variability of UV irradiance in Moscow, J. Geophys. Res., [Atmos.], 2000, 105, 12529–12539 CrossRef CAS.
- J. C. Farman, B. G. Gardiner and J. D. Shanklin, Large losses of total ozone in Antarctica reveal seasonal ClOx/NOx interaction, Nature, 1985, 315, 207–210 CrossRef CAS.
- Z. Gao, N.-B. Chang and W. Gao, Comparative analyses of the ultraviolet-B flux over the continental United State based on the NASA total ozone mapping spectrometer data and USDA ground-based measurements, J. Appl. Remote Sens., 2010, 4, 043547 CrossRef PubMed.
- M. G. Kimlin, K. A. Schallhorn, R. S. Meltzer, J. R. Slusser and K. Lantz, Comparison of ultraviolet data from colocated instruments from the U.S. EPA Brewer Spectrophotometer Network and the U.S. Department of Agriculture UV-B Monitoring and Research Program, Opt. Eng., 2005, 44, 1–8 Search PubMed.
- V. E. Fioletov, L. J. McArthur, T. W. Mathews and L. Marrett, Estimated ultraviolet exposure levels for a sufficient vitamin D status in North America, J. Photochem. Photobiol., B, 2010, 100, 57–66 CrossRef CAS PubMed.
- H. He, V. E. Fioletov, D. W. Tarasick, T. W. Mathews and C. Long, Validation of Environment Canada and NOAA UV Index Forecasts with Brewer Measurements from Canada, J. Appl. Meteorol. Climatol., 2013, 52, 1477–1489 CrossRef.
- S. Cabrera, A. Ipiña, A. Damiani, R. R. Cordero and R. D. Piacentini, UV index values and trends in Santiago, Chile (33.5°S) based on ground and satellite data, J. Photochem. Photobiol., B, 2012, 115, 73–84 CrossRef CAS PubMed.
- S. Diaz, M. Vernet, A. Paladini, H. Fuenzalida, G. Deferrari, C. R. Booth, S. Cabrera, C. Casiccia, M. Dieguez, C. Lovengreen, J. Pedroni, A. Rosales and J. Vrsalovic, Availability of vitamin D photoconversion weighted UV radiation in southern South America, Photochem. Photobiol. Sci., 2011, 10, 1854–1867 CAS.
- J. Krzyścin, P. Sobolewski, J. Jarosławski, J. Podgórski and B. Rajewska-Więch, Erythemal UV observations at Belsk, Poland, in the period 1976–2008: Data homogenization, climatology, and trends, Acta Geophys., 2011, 59, 155–182 CrossRef PubMed.
- G. Seckmeyer, D. Pissulla, M. Glandorf, D. Henriques, B. Johnsen, A. Webb, A. M. Siani, A. Bais, B. Kjeldstad, C. Brogniez, J. Lenoble, B. Gardiner, P. Kirsch, T. Koskela, J. Kaurola, B. Uhlmann, H. Slaper, P. den Outer, M. Janouch, P. Werle, J. Grobner, B. Mayer, A. de la Casiniere, S. Simic and F. Carvalho, Variability of UV irradiance in Europe, Photochem. Photobiol., 2008, 84, 172–179 CAS.
- M. P. Utrillas, M. J. Marín, A. R. Esteve, V. Estellés, S. Gandía, J. A. Núnez and J. A. Martínez-Lozano, Ten years of measured UV Index from the Spanish UVB Radiometric Network, J. Photochem. Photobiol., B, 2013, 125, 1–7 CrossRef CAS PubMed.
- A. M. Siani, S. Modesti, G. R. Casale, H. Diemoz and A. Colosimo, Biologically effective surface UV climatology at Rome and Aosta, Italy, AIP Conf. Proc., 2013, 1531, 903–906 CrossRef PubMed.
- R. L. McKenzie, J. B. Liley and L. O. Björn, UV Radiation: Balancing Risks and Benefits, Photochem. Photobiol., 2009, 85, 88–98 CrossRef CAS PubMed.
- P. Gies, A. Klekociuk, M. Tully, S. Henderson, J. Javorniczky, K. King, L. Lemus-Deschamps and J. Makin, Low Ozone Over Southern Australia in August 2011 and its Impact on Solar Ultraviolet Radiation Levels, Photochem. Photobiol., 2013, 89, 984–994 CrossRef CAS PubMed.
- G. Bernhard, A. Dahlback, V. Fioletov, A. Heikkilä, B. Johnsen, T. Koskela, K. Lakkala and T. Svendby, High levels of ultraviolet radiation observed by ground-based instruments below the 2011 Arctic ozone hole, Atmos. Chem. Phys., 2013, 13, 10573–10590 Search PubMed.
-
G. Bernhard, C. Booth and J. Ehramjian, Climatology of Ultraviolet Radiation at High Latitudes Derived from Measurements of the National Science Foundation's Ultraviolet Spectral Irradiance Monitoring Network, in UV Radiation in Global Climate Change, ed. W. Gao, J. Slusser and D. Schmoldt, Springer, Berlin, Heidelberg, 2010, pp. 48–72 Search PubMed.
- N. P. Sharma, B. K. Bhattarai, B. Sapkota and B. Kjeldstad, Satellite estimation and ground measurements of solar UV index and influence of aerosol in ground based UV data in Kathmandu, Nepal, Int. J. Environ. Sci., 2013, 3, 1362–1372 CAS.
- S. Buntoung and A. R. Webb, Comparison of erythemal UV irradiances from Ozone Monitoring Instrument (OMI) and ground-based data at four Thai stations, J. Geophys. Res., [Atmos.], 2010, 115, D18215 CrossRef.
- S. Janjai, S. Wisitsirikun, S. Buntoung, S. Pattarapanitchai, R. Wattan, I. Masiri and B. K. Bhattarai, Comparison of UV index from Ozone Monitoring Instrument (OMI) with multi-channel filter radiometers at four sites in the tropics: effects of aerosols and clouds, Int. J. Climatol., 2013, 34, 453–461 CrossRef.
- B. Hu and Y. S. Wang, Ultraviolet radiation in the background region over Northeast China, J. Atmos. Chem., 2013, 70, 283–296 CrossRef CAS.
-
A. Webb, B. G. Gardiner, K. Leszczynski, V. A. Mohnen, P. Johnston, N. Harrison and D. Bigelow, Quality Assurance in Monitoring Solar Ultraviolet Radiation: The State of the Art, World Meteorological Organisation, Global Atmospheric Watch, Report No. 146, Geneva, 2003.
-
G. Seckmeyer, A. Bais, G. Bernhard, M. Blumthaler, S. Drüke, P. Kiedron, K. Lantz, R. L. McKenzie and S. Riechelmann, Instruments to Measure Solar Ultraviolet Radiation Part 4: Array Spectroradiometers, World Meteorological Organization, Global Atmospheric Watch, Report No. 191, Geneva, 2010, p. 43.
- A. Kreuter, R. Buras, B. Mayer, A. Webb, R. Kift, A. Bais, N. Kouremeti and M. Blumthaler, Solar irradiance in the heterogeneous albedo environment of the Arctic coast: measurements and a 3-D model study, Atmos. Chem. Phys., 2014, 14, 5989–6002 CrossRef CAS.
- R. R. Cordero, G. Seckmeyer, S. Riechelmann, A. Damiani and F. Labbe, Monte Carlo-based uncertainty analysis of UV array spectroradiometers, Metrologia, 2012, 49, 745 CrossRef.
- R. R. Cordero, G. Seckmeyer, A. Damiani, F. Labbe and D. Laroze, Monte Carlo-based uncertainties of surface UV estimates from models and from spectroradiometers, Metrologia, 2013, 50 DOI:10.1088/0026-1394/50/5/L1.
- D. Vernez, A. Milon, L. Vuilleumier and J. L. Bulliard, Anatomical exposure patterns of skin to sunlight: relative contributions of direct, diffuse and reflected ultraviolet radiation, Br. J. Dermatol., 2012, 167, 383–390 CrossRef CAS PubMed.
- G. Seckmeyer, M. Schrempf, A. Wieczorek, S. Riechelmann, K. Graw, S. Seckmeyer and M. Zankl, A novel method to calculate solar UV exposure relevant to vitamin D production in humans, Photochem. Photobiol., 2013, 89, 974–983 CrossRef CAS PubMed.
- S. Riechelmann, M. Schrempf and G. Seckmeyer, Simultaneous measurement of spectral sky radiance by a non-scanning multidirectional spectroradiometer (MUDIS), Meas. Sci. Technol., 2013, 24, 125501 CrossRef.
- A. Lindfors, A. Tanskanen, A. Arola, R. van der A, A. Bais, U. Feister, M. Janouch, W. Josefsson, T. Koskela, K. Lakkala, P. N. den Outer, A. R. D. Smedley, H. Slaper and A. R. Webb, The PROMOTE UV record: Toward a global satellite-based climatology of surface ultraviolet irradiance, IEEE J. Sel. Top. Appl. Earth Obs. Remote Sens., 2009, 2, 207–212 CrossRef.
- A. Arola, S. Kazadzis, A. Lindfors, N. Krotkov, J. Kujanpää, J. Tamminen, A. Bais, A. d. Sarra, J. M. Villaplana, C. Brogniez, A. M. Siani, M. Janouch, P. Weihs, A. Webb, T. Koskela, N. Kouremeti, D. Meloni, V. Buchard, F. Auriol, I. Ialongo, M. Staneck, S. Simic, A. Smedley and S. Kinne, A new approach to correct for absorbing aerosols in OMI UV, Geophys. Res. Lett., 2009, 36, L22805 CrossRef.
- A. Damiani, S. Cabrera, R. C. Muñoz, R. R. Cordero and F. Labbe, Satellite-derived UV irradiance for a region with complex morphology and meteorology: comparison against ground measurements in Santiago de Chile, Int. J. Remote Sens., 2013, 34, 5812–5833 CrossRef.
- R. R. Cordero, G. Seckmeyer, A. Damiani, J. Jorquera, J. Carrasco, R. Muñoz, L. Da Silva, F. Labbe and D. Laroze, Aerosol effects on the UV irradiance in Santiago de Chile, Atmos. Res., 2014, 149, 282–291 CrossRef CAS PubMed.
- F. Jégou, S. Godin-Beekman, M. P. Corrêa, C. Brogniez, F. Auriol, V. H. Peuch, M. Haeffelin, A. Pazmino, P. Saiag, F. Goutail and E. Mahé, Validity of satellite measurements used for the monitoring of UV radiation risk on health, Atmos. Chem. Phys., 2011, 11, 13377–13394 CrossRef.
- M. Antón, V. E. Cachorro, J. M. Vilaplana, C. Toledano, N. A. Krotkov, A. Arola, A. Serrano and B. de la Morena, Comparison of UV irradiances from Aura/Ozone Monitoring Instrument (OMI) with Brewer measurements at El Arenosillo (Spain) – Part 1: Analysis of parameter influence, Atmos. Chem. Phys., 2010, 10, 5979–5989 CrossRef.
- M. Xu, X. Z. Liang, W. Gao and N. Krotkov, Comparison of TOMS retrievals and UVMRP measurements of surface spectral UV radiation in the United States, Atmos. Chem. Phys., 2010, 10, 8669–8683 CrossRef CAS.
- J. E. Wagner, F. Angelini, A. Arola, M. Blumthaler, M. Fitzka, G. P. Gobbi, R. Kift, A. Kreuter, H. E. Rieder, S. Simic, A. N. N. Webb and P. Weihs, Comparison of surface UV irradiance in mountainous regions derived from satellite observations and model calculations with ground-based measurements, Metero. Zeits., 2010, 19, 481–490 CrossRef.
- P. Pandey, K. De Ridder, D. Gillotay and N. P. M. van Lipzig, Estimating cloud optical thickness and associated surface UV irradiance from SEVIRI by implementing a semi-analytical cloud retrieval algorithm, Atmos. Chem. Phys., 2012, 12, 7961–7975 CrossRef CAS.
-
R. L. McKenzie, P. V. Johnston and G. Seckmeyer, UV spectro-radiometry in the network for the detection of stratospheric change (NDSC), in Solar Ultraviolet Radiation. Modelling, Measurements and Effects, ed. C. S. Zerefos and A. F. Bais, Springer-Verlag, Berlin, 1997, vol. 1.52, pp. 279–287 Search PubMed.
- G. Seckmeyer and R. L. McKenzie, Increased ultraviolet-radiation in New-Zealand (45-degrees-S) relative to Germany (48-degrees-N), Nature, 1992, 359, 135–137 CrossRef.
- F. Zaratti, R. D. Piacentini, H. A. Guillen, S. Cabrera, B. Liley and R. McKenzie, Proposal for a modification of the UVI Risk Scale, Photochem. Photobiol. Sci., 2014, 13, 980–985 CAS.
- R. R. Cordero, G. Seckmeyer, A. Damiani, S. Riechelmann, J. Rayas, F. Labbe and D. Laroze, The world's highest levels of surface UV, Photochem. Photobiol. Sci., 2014, 13, 70–81 CAS.
- G. Norsang, Y.-C. Chen, N. Pingcuo, A. Dahlback, Ø. Frette, B. Kjeldstad, B. Hamre, K. Stamnes and J. J. Stamnes, Comparison of ground-based measurements of solar UV radiation at four sites on the Tibetan Plateau, Appl. Opt., 2014, 53, 736–747 CrossRef PubMed.
- U. Feister, G. Meyer and U. Kirst, Solar UV exposure of seafarers along subtropical and tropical shipping routes, Photochem. Photobiol., 2013, 89, 1497–1506 CrossRef CAS PubMed.
- U. Feister, G. Laschewski and R.-D. Grewe, UV index forecasts and measurements of health-effective radiation, J. Photochem. Photobiol., B, 2011, 102, 55–68 CrossRef CAS PubMed.
- N. A. Cabrol, U. Feister, D.-P. Häder, H. Piazena, E. A. Grin and A. Klein, Record solar UV irradiance in the tropical Andes, Front. Environ. Sci., 2014, 2 DOI:10.3389/fenvs.2014.00019.
- A. Y. Karpechko, L. Backman, L. Thölix, I. Ialongo, M. Andersson, V. Fioletov, A. Heikkilä, B. Johnsen, T. Koskela, E. Kyrölä, K. Lakkala, C. L. Myhre, M. Rex, V. F. Sofieva, J. Tamminen and I. Wohltmann, The link between springtime total ozone and summer UV radiation in Northern Hemisphere extratropics, J. Geophys. Res., [Atmos.], 2013, 118, 8649–8661 CAS.
-
J. Lee-Taylor, S. Madronich, C. Fischer and B. Mayer, A Climatology of UV Radiation, 1979–2000, 65S–65N, in UV Radiation in Global Climate Change, ed. W. Gao, J. Slusser and D. Schmoldt, Springer, Berlin, Heidelberg, 2010, pp. 1–20 Search PubMed.
- J. R. Herman, Use of an improved radiation amplification factor to estimate the effect of total ozone changes on action spectrum weighted irradiances and an instrument response function, J. Geophys. Res., [Atmos.], 2010, 115, D23119 CrossRef.
- R. L. McKenzie, P. J. Aucamp, A. F. Bais, L. O. Björn, M. Ilyas and S. Madronich, Ozone depletion and climate change: Impacts on UV radiation, Photochem. Photobiol. Sci., 2011, 10, 182–198 CAS.
- I. Ialongo, A. Arola, J. Kujanpää and J. Tamminen, Use of satellite erythemal UV products in analysing the global UV changes, Atmos. Chem. Phys., 2011, 11, 9649–9658 CrossRef CAS.
- A. Tanskanen, A. Lindfors, A. Maatta, N. Krotkov, J. Herman, J. Kaurola, T. Koskela, K. Lakkala, V. Fioletov, G. Bernhard, R. McKenzie, Y. Kondo, M. O'Neill, H. Slaper, P. den Outer, A. F. Bais and J. Tamminen, Validation of daily erythemal doses from ozone monitoring instrument with ground-based UV measurement data, J. Geophys. Res., [Atmos.], 2007, 112, D24S44 CrossRef.
- G. J. Labow, J. R. Herman, L.-K. Huang, S. A. Lloyd, M. T. DeLand, W. Qin, J. Mao and D. E. Larko, Diurnal variation of 340 nm Lambertian equivalent reflectivity due to clouds and aerosols over land and oceans, J. Geophys. Res., [Atmos.], 2011, 116, D11202 CrossRef.
- J. R. Herman, M. T. DeLand, L. K. Huang, G. Labow, D. Larko, S. A. Lloyd, J. Mao, W. Qin and C. Weaver, A net decrease in the Earth's cloud, aerosol, and surface 340 nm reflectivity during the past 33 year (1979–2011), Atmos. Chem. Phys., 2013, 13, 8505–8524 CrossRef CAS.
- C. S. Zerefos, K. Tourpali, K. Eleftheratos, S. Kazadzis, C. Meleti, U. Feister, T. Koskela and A. Heikkilä, Evidence of a possible turning point in solar UV-B over Canada, Europe and Japan, Atmos. Chem. Phys., 2012, 12, 2469–2477 CAS.
- A. M. Vogelmann, T. P. Ackerman and R. P. Turco, Enhancements in biologically effective ultraviolet radiation following volcanic eruptions, Nature, 1992, 359, 47 CrossRef CAS PubMed.
- G. Bernhard, C. R. Booth, J. C. Ehramjian, R. Stone and E. G. Dutton, Ultraviolet and visible radiation at Barrow, Alaska: Climatology and influencing factors on the basis of version 2 National Science Foundation network data, J. Geophys. Res., [Atmos.], 2007, 112, D09101 CrossRef.
- A. F. Bais, T. Drosoglou, C. Meleti, K. Tourpali and N. Kouremeti, Changes in surface shortwave solar irradiance from 1993 to 2011 at Thessaloniki (Greece), Int. J. Climatol., 2013, 33, 2871–2876 CrossRef.
- R. Philipona, K. Behrens and C. Ruckstuhl, How declining aerosols and rising greenhouse gases forced rapid warming in Europe since the 1980s, Geophys. Res. Lett., 2009, 36, L02806 CrossRef.
- A. Sanchez-Lorenzo, J. Calbó and M. Wild, Global and diffuse solar radiation in Spain: Building a homogeneous dataset and assessing their trends, Global Planet. Change, 2013, 100, 343–352 CrossRef PubMed.
- S. Nyeki, C. H. Halios, W. Baum, K. Eleftheriadis, H. Flentje, J. Gröbner, L. Vuilleumier and C. Wehrli, Ground-based aerosol optical depth trends at three high-altitude sites in Switzerland and southern Germany from 1995 to 2010, J. Geophys. Res., [Atmos.], 2012, 117, D18202 CrossRef.
- A. R. D. Smedley, J. S. Rimmer, D. Moore, R. Toumi and A. R. Webb, Total ozone and surface UV trends in the United Kingdom: 1979–2008, Int. J. Climatol., 2012, 32, 338–346 CrossRef.
- M. Fitzka, S. Simic and J. Hadzimustafic, Trends in spectral UV radiation from long-term measurements at Hoher Sonnblick, Austria, Theotet. Appl. Climatol., 2012, 110, 585–593 CrossRef.
- G. Bernhard, Trends of solar ultraviolet irradiance at Barrow, Alaska, and the effect of measurement uncertainties on trend detection, Atmos. Chem. Phys., 2011, 11, 13029–13045 CrossRef CAS.
- K. Eleftheratos, S. Kazadzis, C. S. Zerefos, K. Tourpali, C. Meleti, D. Balis, I. Zyrichidou, K. Lakkala, U. Feister, T. Koskela, A. Heikkilä and J. M. Karhu, Ozone and spectroradiometric UV changes in the past 20 years over high latitudes, Atmos.–Ocean, 2014 DOI:10.1080/07055900.2014.919897.
- S. Watanabe, T. Takemura, K. Sudo, T. Yokohata and H. Kawase, Anthropogenic changes in the surface all-sky UV-B radiation through 1850–2005 simulated by an Earth system model, Atmos. Chem. Phys., 2012, 12, 5249–5257 CrossRef CAS.
- J. Junk, U. Feister, A. Helbig, K. Görgen, E. Rozanov, J. W. Krzyścin and L. Hoffmann, The benefit of modeled ozone data for the reconstruction of a 99-yearUV radiation time series, J. Geophys. Res., [Atmos.], 2012, 117, D16102 CrossRef.
- L. Lemus-Deschamps and J. K. Makin, Fifty years of changes in UV Index and implications for skin cancer in Australia, Int. J. Biometeorol., 2012, 56, 727–735 CrossRef PubMed.
- J. Para, B. Charrière, A. Matsuoka, W. L. Miller, J. F. Rontani and R. Sempéré, UV/PAR radiation and DOM properties in surface coastal waters of the Canadian shelf of the Beaufort Sea during summer 2009, Biogeosciences., 2013, 10, 2761–2774 CrossRef CAS.
- R. G. Zepp, G. C. Shank, E. Stabenau, K. W. Patterson, M. Cyterski, W. Fisher, E. Bartels and S. L. Anderson, Spatial and temporal variability of solar ultraviolet exposure of coral assemblages in the Florida Keys: Importance of colored dissolved organic matter, Limnol. Oceanogr., 2008, 53, 1909–1922 CrossRef CAS.
- B. B. Barnes, C. Hu, J. P. Cannizzaro, S. E. Craig, P. Hallock, D. L. Jones, J. C. Lehrter, N. Melo, B. A. Schaeffer and R. Zepp, Estimation of diffuse attenuation of ultraviolet light in optically shallow Florida Keys waters from MODIS measurements, Rem. Sens. Environ., 2014, 140, 519–532 CrossRef PubMed.
- M. Tedetti, R. Sempéré, A. Vasilkov, B. Charrière, D. Nérini, W. L. Miller, K. Kawamura and P. Raimbault, High penetration of ultraviolet radiation in the south east Pacific waters, Geophys. Res. Lett., 2007, 34, L12610 CrossRef.
- M. P. Gall, R. J. Davies-Colley and R. A. Merrilees, Exceptional visual clarity and optical purity in a sub-alpine lake, Limnol. Oceanogr., 2013, 58, 443–451 Search PubMed.
- J. A. L. Gareis, L. F. W. Lesack and M. L. Bothwell, Attenuation of in situ UV radiation in Mackenzie Delta lakes with varying dissolved organic matter compositions, Water Resou. Res., 2010, 46, W09516 Search PubMed.
- D. K. Perovich, The interaction of ultraviolet light with Arctic sea ice during SHEBA, Ann. Glaciol., 2006, 44, 47–52 CrossRef CAS.
- I. Fountoulakis, A. F. Bais, K. Tourpali, K. Fragkos and S. Misios, Projected changes in solar UV radiation in the Arctic and sub-Arctic Ocean: Effects from changes in reflectivity, ice transmittance, clouds, and ozone, J. Geophys. Res., [Atmos.], 2014, 119, 8073–8090 Search PubMed.
- A. F. Bais, K. Tourpali, A. Kazantzidis, H. Akiyoshi, S. Bekki, P. Braesicke, M. P. Chipperfield, M. Dameris, V. Eyring, H. Garny, D. Iachetti, P. Jöckel, A. Kubin, U. Langematz, E. Mancini, M. Michou, O. Morgenstern, T. Nakamura, P. A. Newman, G. Pitari, D. A. Plummer, E. Rozanov, T. G. Shepherd, K. Shibata, W. Tian and Y. Yamashita, Projections of UV radiation changes in the 21st century: impact of ozone recovery and cloud effects, Atmos. Chem. Phys., 2011, 11, 7533–7545 CrossRef CAS.
- T. Egorova, E. Rozanov, J. Gröbner, M. Hauser and W. Schmutz, Montreal Protocol Benefits simulated with CCM SOCOL, Atmos. Chem. Phys., 2013, 13, 3811–3823 CrossRef CAS.
- M. d. P. Correa, S. Godin-Beekmann, M. Haeffelin, S. Bekki, P. Saiag, J. Badosa, F. Jegou, A. Pazmino and E. Mahe, Projected changes in clear-sky erythemal and vitamin D effective UV doses for Europe over the period 2006 to 2100, Photochem. Photobiol. Sci., 2013, 12, 1053–1064 CAS.
- K. E. Taylor, R. J. Stouffer and G. A. Meehl, An overview of CMIP5 and the experiment design, Bull. Am. Meteorol. Soc., 2011, 93, 485–498 CrossRef.
- V. Eyring, J. M. Arblaster, I. Cionni, J. Sedláček, J. Perlwitz, P. J. Young, S. Bekki, D. Bergmann, P. Cameron-Smith, W. J. Collins, G. Faluvegi, K. D. Gottschaldt, L. W. Horowitz, D. E. Kinnison, J. F. Lamarque, D. R. Marsh, D. Saint-Martin, D. T. Shindell, K. Sudo, S. Szopa and S. Watanabe, Long-term ozone changes and associated climate impacts in CMIP5 simulations, J. Geophys. Res., [Atmos.], 2013, 118, 5029–5060 CAS.
- P. R. Gent, G. Danabasoglu, L. J. Donner, M. M. Holland, E. C. Hunke, S. R. Jayne, D. M. Lawrence, R. B. Neale, P. J. Rasch, M. Vertenstein, P. H. Worley, Z.-L. Yang and M. Zhang, The Community Climate System Model Version 4, J. Clim., 2011, 24, 4973–4991 CrossRef.
- A. Hilboll, A. Richter and J. P. Burrows, Long-term changes of tropospheric NO2 over megacities derived from multiple satellite instruments, Atmos. Chem. Phys., 2013, 13, 4145–4169 CAS.
- J.-F. Lamarque, G. P. Kyle, M. Meinshausen, K. Riahi, S. J. Smith, D. P. van Vuuren, A. J. Conley and F. Vitt, Global and regional evolution of short-lived radiatively-active gases and aerosols in the Representative Concentration Pathways, Clim. Change, 2011, 109, 191–212 CrossRef CAS PubMed.
- Y. W. Wang and Y. H. Yang, China's dimming and brightening: evidence, causes and hydrological implications, Ann. Geophys., 2014, 32, 41–55 CrossRef PubMed.
- D. A. Randall, Beyond deadlock, Geophys. Res. Lett., 2013, 40, 5970–5976 CrossRef.
- V. Aquila, L. D. Oman, R. Stolarski, A. R. Douglass and P. A. Newman, The response of ozone and nitrogen dioxide to the eruption of Mt. Pinatubo at southern and northern midlatitudes, J. Atmos. Sci., 2012, 70, 894–900 CrossRef PubMed.
- R. R. Garcia, D. E. Kinnison and D. R. Marsh, “World avoided” simulations with the Whole Atmosphere Community Climate Model, J. Geophys. Res., [Atmos.], 2012, 117, D23303 CrossRef.
- P. A. Newman and R. L. McKenzie, UV impacts avoided by the Montreal Protocol, Photochem. Photobiol. Sci., 2011, 10, 1152–1160 CAS.
- P. A. Newman, L. D. Oman, A. R. Douglass, E. L. Fleming, S. M. Frith, M. M. Hurwitz, S. R. Kawa, C. H. Jackman, N. A. Krotkov, E. R. Nash, J. E. Nielsen, S. Pawson, R. S. Stolarski and G. J. M. Velders, What would have happened to the ozone layer if chlorofluorocarbons (CFCs) had not been regulated?, Atmos. Chem. Phys., 2009, 9, 2113–2128 CrossRef CAS.
- A. van Dijk, H. Slaper, P. N. d. Outer, O. Morgenstern, P. Braesicke, J. A. Pyle, H. Garny, A. Stenke, M. Dameris, A. Kazantzidis, K. Tourpali and A. F. Bais, Skin cancer risks avoided by the Montreal Protocol – worldwide modelling integrating coupled climate-chemistry models with a risk model for UV, Photochem. Photobiol. Sci., 2013, 89, 234–246 CAS.
- R. M. Lucas, M. Norval, R. E. Neale, A. R. Young, F. R. d. Gruijl, Y. Takizawa and J. C. v. d. Leun, The consequences for human health of stratospheric ozone depletion in association with other environmental factors, Photochem. Photobiol. Sci., 2015, 14 10.1039/c4pp90033b , this issue.
- A. W. Schmalwieser, S. Wallisch and B. Diffey, A library of action spectra for erythema and pigmentation, Photochem. Photobiol. Sci., 2012, 11, 251–268 CAS.
- M. I. Micheletti, R. D. Piacentini and S. Madronich, Sensitivity of biologically active UV radiation to stratospheric ozone changes: Effects of action spectrum shape and wavelength range, Photochem. Photobiol., 2003, 78, 456–461 CrossRef CAS.
- M. Norval, L. O. Björn and F. R. d. Gruijl, Is the action spectrum for the UV-induced production of previtamin D3 in human skin correct?, Photochem. Photobiol. Sci., 2009, 9, 11–17 Search PubMed.
-
R. Bouillon, J. Eisman, M. Garabedian, M. Holick, J. Kleinschmidt, T. Suda, I. Terenetskaya and A. Webb, Action Spectrum for the Production of Previtamin D3 in Human Skin, CIE Report No 174, Vol. 174:2006, Commission Internationale de L'Eclairage, Vienna, 2006.
-
CIE, Erythema Reference Action Spectrum and Standard Erythema Dose, CIE DS 007.1/E-1998, Commission Internationale de l'Eclairage, Vienna, Austria, 1998 Search PubMed.
-
A. F. McKinlay and B. L. Diffey, A Reference Action Spectrum for Ultraviolet Induced Erythema in Human Skin, in Human Exposure to Ultraviolet Radiation: Risks and Regulations, ed. W. F. Passchier and B. F. M. Bosnajakovic, Elsevier, Amsterdam, 1987, pp. 83–87 Search PubMed.
- A. R. Webb, H. Slaper, P. Koepke and A. W. Schmalwieser, Know your standard: Clarifying the CIE erythema action spectrum, Photochem. Photobiol., 2011, 87, 483–486 CrossRef CAS PubMed.
-
W. Olds, Elucidating the Links Between UV Radiation and Vitamin D Synthesis: Using an In Vitro Model, PhD Thesis, Queensland University of Technology, Brisbane, 2010 Search PubMed.
- D. Bolsee, A. R. Webb, D. Gillotay, B. Dorschel, P. Knuschke, A. Krins and I. Terenetskaya, Laboratory facilities and recommendations for the characterization of biological ultraviolet dosimeters, Appl. Opt., 2000, 39, 2813–2822 CrossRef CAS.
- V. Vitale, B. Petkov, F. Goutail, C. Lanconelli, A. Lupi, M. Mazzola, M. Busetto, A. Pazmino, R. Schioppo, L. Genoni and C. Tomasi, Variations of UV irradiance at Antarctic station Concordia during the springs of 2008 and 2009, Antarct. Sci., 2011, 23, 389–398 CrossRef.
- B. Petkov, V. Vitale, J. Gröbner, G. Hülsen, S. De Simone, V. Gallo, C. Tomasi, M. Busetto, V. L. Barth, C. Lanconelli and M. Mazzola, Short-term variations in surface UV-B irradiance and total ozone column at Ny-Ålesund during the QAARC campaign, Atmos. Res., 2012, 108, 9–18 CrossRef CAS PubMed.
-
CIE, A Reference Action Spectrum for Ultraviolet Induced Erythemal in Human Skin, Commission Internationale de l'Eclairage, Vienna, Austria, 1987, vol. 61 Search PubMed.
-
G. Seckmeyer, A. Bais, G. Bernhard, M. Blumthaler, C. R. Booth, K. Lantz and R. L. McKenzie, Instruments to measure solar ultraviolet irradiance. Part 2: Broadband instruments measuring erythemally weighted solar irradiance, World Meteorological Organisation, Global Atmospheric Watch, Report No. 164, Geneva, 2005.
- E. T. Butson, T. Cheung, P. K. N. Yu and M. J. Butson, Measuring solar UV radiation with EBT radiochromic film, Phys. Med. Biol., 2010, 55, N487–N493 CrossRef PubMed.
- A. M. Siani, G. R. Casale, R. Sisto, A. Colosimo, C. A. Lang and M. G. Kimlin, Occupational Exposures to Solar Ultraviolet Radiation of Vineyard Workers in Tuscany (Italy), Photochem. Photobiol., 2011, 87, 925–934 CrossRef CAS PubMed.
- J. Turner and A. V. Parisi, Influence of reflected UV irradiance on occupational exposure from combinations of reflective wall surfaces, Photochem. Photobiol. Sci., 2013, 12, 1589–1595 CAS.
- M.-A. Serrano, J. Canada and J. C. Moreno, Erythemal ultraviolet exposure of cyclists in Valencia, Spain, Photochem. Photobiol., 2010, 86, 716–721 CrossRef CAS PubMed.
- M.-A. Serrano, J. Canada, J. C. Moreno and Members of the Research Group of Solar Radiation of Valencia, Erythemal ultraviolet solar radiation doses received by young skiers, Photochem. Photobiol. Sci., 2013, 12, 1976–1983 CAS.
- A. W. Schmalwieser, A. Cabaj, G. Schauberger, H. Rohn, B. Maier and H. Maier, Facial solar UV exposure of Austrian farmers during occupation, Photochem. Photobiol., 2010, 86, 1404–1413 CrossRef CAS PubMed.
- A. W. Schmalwieser, C. Enzi, S. Wallisch, F. Holawe, B. Maier and P. Weihs, UV exposition during typical lifestyle behavior in an urban environment, Photochem. Photobiol., 2010, 86, 711–715 CrossRef CAS PubMed.
- P. Weihs, A. Schmalwieser, C. Reinisch, E. Meraner, S. Walisch and M. Harald, Measurements of personal UV exposure on different parts of the body during various activities, Photochem. Photobiol., 2013, 89, 1004–1007 CrossRef CAS PubMed.
- A. M. Siani, G. R. Casale, S. Modesti, A. V. Parisi and A. Colosimo, Investigation on the capability of polysulphone for measuring biologically effective solar UV exposures, Photochem. Photobiol. Sci., 2014, 13, 521–530 CAS.
- G. R. Casale, A. M. Siani, H. Diémoz, M. G. Kimlin and A. Colosimo, Applicability of the Polysulphone Horizontal Calibration to Differently Inclined Dosimeters, Photochem. Photobiol., 2012, 88, 207–214 CrossRef CAS PubMed.
- R. L. McKenzie, B. Liley, P. Johnston, R. Scragg, A. Stewart, A. I. Reeder and M. W. Allen, Small doses from artificial UV sources elucidate the photo-production of vitamin D, Photochem. Photobiol. Sci., 2013, 12, 1726–1737 CAS.
- G. Seckmeyer, M. Klingebiel, S. Riechelmann, I. Lohse, R. L. McKenzie, J. Ben Liley, M. W. Allen, A.-M. Siani and G. R. Casale, A critical assessment of two types of personal UV dosimeters, Photochem. Photobiol., 2012, 88, 215–222 CrossRef CAS PubMed.
- P. Datta, M. K. Bogh, P. Olsen, P. Eriksen, A. V. Schmedes, M. M.-L. Grage, P. A. Philipsen and H. C. Wulf, Increase in serum 25-hydroxyvitamin-D3 in humans after solar exposure under natural conditions compared to artificial UVB exposure of hands and face, Photochem. Photobiol. Sci., 2012, 11, 1817–1824 CAS.
- M. d. P. Correa, S. Godin-Beekmann, M. Haeffelin, C. Brogniez, F. Verschaeve, P. Saiag, A. Pazmino and E. Mahe, Comparison between UV index measurements performed by research-grade and consumer-products instruments, Photochem. Photobiol. Sci., 2010, 9, 459–463 CAS.
- D.-P. Häder, C. E. Williamson, S.-Å. Wängberg, M. Rautio, K. C. Rose, K. Gao, E. W. Helbling, R. P. Sinha and R. Worrest, Effects of UV radiation on aquatic ecosystems and interactions with other environmental factors, Photochem. Photobiol. Sci., 2015, 14 10.1039/c4pp90035a , this issue.
- A. L. Andrady, H. H. Redhwi, AyakoTorikai, K. K. Pandey and P. Gies, Consequences of stratospheric ozone depletion and climate change on the use of materials, Photochem. Photobiol. Sci., 2015, 14 10.1039/c4pp90038c , this issue.
- R. L. McKenzie, R. Scragg, B. Liley, P. Johnston, J. Wishart, A. Stewart and R. Prematunga, Serum 25-hydroxyvitamin-D responses to multiple UV exposures from solaria: inferences for exposure to sunlight, Photochem. Photobiol. Sci., 2012, 11, 1174–1185 CAS.
- A. R. Webb, R. Kift, J. L. Berry and L. E. Rhodes, The vitamin D debate: Translating controlled experiments into reality for human sun exposure times, Photochem. Photobiol., 2011, 87, 741–745 CrossRef CAS PubMed.
- N. Chubarova and Y. Zhdanova, Ultraviolet resources over Northern Eurasia, J. Photochem. Photobiol., B, 2013, 127, 38–51 CrossRef CAS PubMed.
- S. J. Pope and D. E. Godar, Solar UV Geometric Conversion Factors: Horizontal Plane to Cylinder Model dagger, Photochem. Photobiol., 2010, 86, 457–466 CrossRef CAS PubMed.
- D. J. Turnbull and A. V. Parisi, Latitudinal variations over Australia of the solar UV-radiation exposures for vitamin D(3) in shade compared to full sun, Radiat. Res., 2010, 173, 373–379 CrossRef CAS PubMed.
-
CIE, Rationalizing Nomenclature for UV Doses and Effects on Humans, CIE 209:2014/WMO/GAW report No. 211, Commission Internationale de l'Eclairage, Vienna, Austria, 2014.
- P. J. Crutzen, Albedo enhancement by stratospheric sulfur injections: A contribution to resolve a policy dilemma?, Clim. Change, 2006, 77, 211–220 CrossRef CAS.
- P. Heckendorn, D. Weisenstein, S. Fueglistaler, B. P. Luo, E. Rozanov, M. Schraner, L. W. Thomason and T. Peter, The impact of geoengineering aerosols on stratospheric temperature and ozone, Environ. Res. Lett., 2009, 4, 045108 CrossRef.
- S. Tilmes, D. E. Kinnison, R. R. Garcia, R. Salawitch, T. Canty, J. Lee-Taylor, S. Madronich and K. Chance, Impact of very short-lived halogens on stratospheric ozone abundance and UV radiation in a geo-engineered atmosphere, Atmos. Chem. Phys., 2012, 12, 10945–10955 CAS.
- F. D. Pope, P. Braesicke, R. G. Grainger, M. Kalberer, I. M. Watson, P. J. Davidson and R. A. Cox, Stratospheric aerosol particles and solar-radiation management, Nat. Clim. Change, 2012, 2, 713–719 CrossRef CAS.
- G. Pitari, V. Aquila, B. Kravitz, A. Robock, S. Watanabe, I. Cionni, N. D. Luca, G. D. Genova, E. Mancini and S. Tilmes, Stratospheric ozone response to sulfate geoengineering: Results from the Geoengineering Model Intercomparison Project (GeoMIP), J. Geophys. Res., [Atmos.], 2014, 119, 2629–2653 CAS.
- M. Kuebbeler, U. Lohmann and J. Feichter, Effects of stratospheric sulfate aerosol geo-engineering on cirrus clouds, Geophys. Res. Lett., 2012, 39, L23803 CrossRef.
- A. J. Ferraro, E. J. Highwood and A. J. Charlton-Perez, Weakened tropical circulation and reduced precipitation in response to geoengineering, Environ. Res. Lett., 2014, 9 DOI:10.1088/1748-9326/9/1/014001.
- N. A. Krotkov, P. K. Bhartia, J. R. Herman, J. R. Slusser, G. R. Scott, G. J. Labow, A. P. Vasilkov, T. Eck, O. Doubovik and B. N. Holben, Aerosol ultraviolet absorption experiment (2002 to 2004), part 2: absorption optical thickness, refractive index, and single scattering albedo, Opt. Eng., 2005, 44, 1–17 Search PubMed.
-
S. Kazadzis, V. Amiridis and N. Kouremeti, The effect of aerosol absorption in solar UV radiation, in Advances in Meteorology, Climatology and Atmospheric Physics, ed. C. G. Helmis and P. T. Nastos, Springer, Berlin, Heidelberg, 2013, pp. 1041–1047 Search PubMed.
- E. Nikitidou, A. Kazantzidis, V. De Bock and H. De Backer, The aerosol forcing efficiency in the UV region and the estimation of single scattering albedo at a typical West European site, Atmos. Environ., 2013, 69, 313–320 CrossRef CAS PubMed.
|
This journal is © The Royal Society of Chemistry and Owner Societies 2015 |
Click here to see how this site uses Cookies. View our privacy policy here.