DOI:
10.1039/C4OB02681K
(Review Article)
Org. Biomol. Chem., 2015,
13, 5570-5585
The tale of RNA G-quadruplex
Received
29th December 2014
, Accepted 1st April 2015
First published on 1st April 2015
Abstract
G-quadruplexes are non-canonical secondary structures found in guanine rich regions of DNA and RNA. Reports have indicated the wide occurrence of RNA G-quadruplexes across the transcriptome in various regions of mRNAs and non-coding RNAs. RNA G-quadruplexes have been implicated in playing an important role in translational regulation, mRNA processing events and maintenance of chromosomal end integrity. In this review, we summarize the structural and functional aspects of RNA G-quadruplexes with emphasis on recent progress to understand the protein/trans factors binding these motifs. With the revelation of the importance of these secondary structures as regulatory modules in biology, we have also evaluated the various advancements towards targeting these structures and the challenges associated with them. Apart from this, numerous potential applications of this secondary motif have also been discussed.
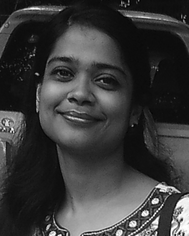 Prachi Agarwala | Prachi Agarwala did her B.Sc. in Zoology (Honours) from Presidency College, Kolkata and M.Sc. in Zoology from the University of Calcutta, Kolkata, India. She is currently pursuing her Ph.D. degree under the supervision of Dr Souvik Maiti at the CSIR-Institute of Genomics and Integrative Biology, New Delhi, India. Her current research focuses on understanding the function of G-quadruplex in the untranslated region of mRNA. |
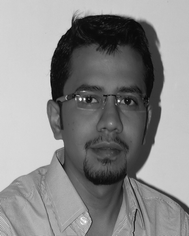 Satyaprakash Pandey | Satyaprakash Pandey obtained his B.Sc. degree in Biotechnology and M.Sc. degree in Life Sciences from the University of Mumbai, India. He is currently pursuing a Ph.D. degree under the supervision of Dr Souvik Maiti at the CSIR-Institute of Genomics and Integrative Biology, New Delhi, India. His present research focuses on studying the role of G-quadruplex in non-coding RNA. |
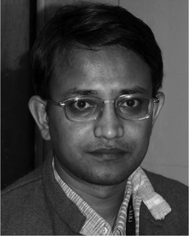 Souvik Maiti | Souvik Maiti completed his M. Sc. in Chemistry from Jadavpur University, Kolkata, India, and Ph.D. in polymer chemistry from CSIR-Institute of Chemical Technology, Hyderabad, India. Subsequently, he performed postdoctoral research on biophysical aspects of DNA secondary structures and development of DNA-based nanomaterials in Professor Luis A. Marky's laboratory at the University of Nebraska Medical Center, Omaha, Nebraska and Professor Francis Rondelez's laboratory, Curie University, Paris, France, respectively. He is currently a Principal Investigator in the Proteomics and Structural Biology Unit of CSIR-Institute of Genomics and Integrative Biology, New Delhi, India. His present research interests involve understanding the structural and functional aspects of G-quadruplexes in non-coding RNA and targeting these structures as well as miRNAs by chemically engineered oligonucleotides or small molecules. |
Introduction
G-quadruplexes are non-canonical four stranded secondary structures found in nucleic acid sequences rich in guanine residues. These structures are formed of stacks of G-quartets – cyclic planar arrangement of four Hoogsteen hydrogen bonded guanine residues (Fig. 1).1 In 1910, high concentration of guanylic acid (GMP) was demonstrated to form gels in aqueous solution.2 Fifty years later, Gellert et al. revealed a G-quadruplex structure based on X-ray fiber diffraction studies,3 where each guanine residue in G-quartet acts both as an acceptor and a donor of two hydrogen bonds.
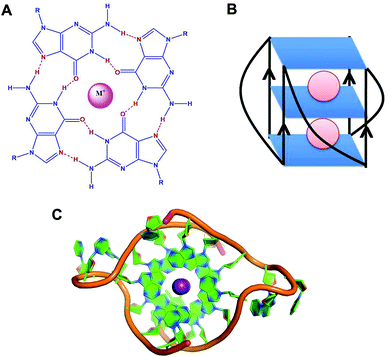 |
| Fig. 1 (A) Schematic representation of a G-quartet. (B) Schematic representation of RNA G-quadruplex with parallel topology. (C) Crystal structure of the intermolecular RNA G-quadruplex (PDB ID – 3IBK).50 Ribose sugar and nitrogenous bases are represented in green colour and the phosphate backbone is represented in orange colour. Potassium ions (K+) are shown in violet colour. | |
G-quadruplexes exhibit diverse topologies depending on the monovalent cation (K+ or Na+), glycosidic conformation (syn or anti), number of molecules of the nucleic acid involved in their formation (intramolecular, bimolecular or tetramolecular), relative orientation of the strands (parallel or antiparallel), number of stacking G-quartets and nucleotide sequences.4,5 Monovalent cations stabilise these structures by interacting with the negatively charged carbonyl oxygen atoms located at the centre of the G-quartet stacks.6 These monovalent cations stabilise the structure in the order of K+ > Na+ > Li+.7
There are about 376
000 DNA G-quadruplex forming sequences in the human genome. Whilst initial studies dealt with understanding the role of G-quadruplexes at the telomere ends, DNA G-quadruplexes are found enriched in the promoters of proto-oncogenes, immunoglobulin heavy chain switch regions and mutational hotspots, and have been implicated in the maintenance of chromosomal integrity and regulation of replication, transcription and recombination processes.8 Owing to the functional association between telomeres, oncogenes and cancer, extensive research on targeting DNA G-quadruplexes for therapeutic intervention is an ongoing active area of research.9,10
Although a considerable amount of work has been conducted on DNA G-quadruplex, recent years have encountered a gradual shift towards understanding G-quadruplexes at the RNA level. In 1991, Kim et al. showed that a 19 nt sequence situated at the 3′ terminus of 5S rRNA of Escherichia coli forms a G-quadruplex.11 Later, RNA G-quadruplex was reported to be present in the 3′ untranslated region (UTR) of insulin-like growth factor II (IGF II) mRNA located just downstream of the endonucleolytic cleavage site.12 Subsequently, various studies have reported the presence of RNA G-quadruplex in the mRNA, long non-coding RNAs and telomeric ends.5,13,14 It is sited in the untranslated region (UTR) and intronic and coding regions of mRNA, further cementing its regulatory potential.15
The existence of RNA G-quadruplex is more likely than its DNA counterpart. DNA exists under double stranded conditions, being base paired with the complementary strand. However, RNA being single stranded, can adopt a complex higher order architecture and is majorly localised in the cytoplasm.16 Various RNA secondary structures such as hairpin, loop, bulge with functional implications have been reported in the literature and discussed in detail elsewhere.17–20 RNA G-quadruplex representing one of these structures has been associated with translational regulation, 3′ end processing, transcription termination, alternative splicing, mRNA localization, protein binding and telomeric RNA biology.15,16,21 Recently, Balasubramanian and coworkers for the first time demonstrated visual localisation of RNA G-quadruplex within the cytoplasm of human cells using a G-quadruplex specific antibody.22 With information on the biological importance of RNA G-quadruplex pouring in over the recent years, here we have reviewed the structural and functional aspects of RNA G-quadruplexes and highlighted various applications of this structure.
Structure
RNA G-quadruplex forms a more thermodynamically stable, compact and less hydrated structure than DNA G-quadruplex (Fig. 2).23,24 Conceptually, the fundamental difference between RNA and DNA quadruplexes is the presence of a ribose sugar instead of a deoxyribose sugar and the presence of uracil instead of a thymine residue. The presence of a 2′ hydroxyl group in its ribose sugars causes more intramolecular interactions within the structure leading to the enhanced stability of RNA G-quadruplex. As a matter of fact, these 2′ hydroxyl groups exert conformational constraints on G-quadruplex topology, preventing it from attaining syn-conformation, a prerequisite for antiparallel topology. Instead it restricts orientation of the base about the glycosidic bond to anti-conformation, imparting C3′ endo puckering and limiting the topology of RNA G-quadruplex to parallel conformation (Fig. 1B).25 This implies less dependence of RNA G-quadruplex topology on the environmental conditions as compared to DNA G-quadruplex.26,27 DNA G-quadruplex can form both parallel and antiparallel conformations. In parallel conformation, the strands in G-quadruplex are oriented in the same direction and propeller loops connect the top of one strand with the bottom of another strand. On the other hand, strands are oriented in opposite directions in antiparallel conformation and lateral loops connect adjacent strands or/and diagonal loops connect diagonally opposite, aiding the antiparallel arrangement of strands. Overall, this monomorphic parallel topology reduces the diversity of RNA G-quadruplex structures as compared to DNA G-quadruplex.28
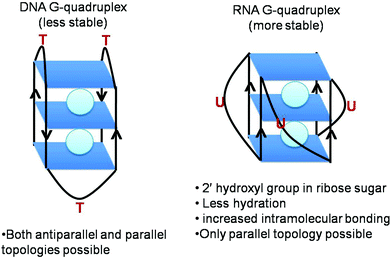 |
| Fig. 2 Differences between DNA G-quadruplex and RNA G-quadruplex. | |
Using the naturally occurring sequences of the bcl-2 gene, Sugimoto and coworkers compared the stability of RNA and DNA G-quadruplexes and revealed their significantly different hydration patterns in spite of their exposition of parallel conformation.28 On a similar line, studies on naturally occurring RNA sequences and their DNA counterparts affirm the more stable nature of RNA quadruplex.23,24,28 The inherent chemistry of the 2′ hydroxyl group of the ribose sugar confers increased stability to RNA G-quadruplex, whose significance is well highlighted in reports where it is replaced with different OH analogues. Substitution of the 2′-OH group by other chemically modified analogues culminated in destabilisation of G-quadruplex.29 The RNA quadruplex was found to be more robust than its DNA counterpart even in the presence of polyethylene glycol.26,27 Thus, the higher stability of RNA G-quadruplex is attributed to the 2′-OH group of the ribose sugar which acts as a scaffold for an ordered network of water molecules and bonding patterns.25
Marky and his group performed an elegant experiment by substituting thymine with uracil in the loop to G-quadruplex and revealed that it stabilises G-quadruplexes and makes them less hydrated. The removal of the methyl group stabilizes the stacking interactions and releases the structured water molecules. By a thymine to uracil substitution, the authors also suggested the importance of loop residues in G-quadruplex stability.30
In addition, various independent studies have demonstrated the effect of varying loop lengths and compositions on the stability of G-quadruplexes at both DNA and RNA levels.31–39 All of them demonstrated that the shorter the loop, the greater is the stability of RNA/DNA G-quadruplex.39 In contrast to the greater importance of the middle quartet in maintaining DNA G-quadruplex stability,40,41 all three G-quartets were found to contribute equally to the stability of RNA G-quadruplex.42 The type of monovalent cation also affects G-quadruplex stability. Potassium ions are preferred over sodium ions because dehydration of the cations prior to their entry in the central channel of stacks formed by G-tetrads causes more energy loss for Na+ than for K+.5,43
Recently, studies on folding dynamics by Mullen et al. have suggested that RNA G-quadruplex formation with two G-quartets shows positive cooperativity, high dependence on K+ concentration and very few populating intermediate states.44 On the other hand, G-quadruplex formation with three G-quartets demonstrates less dependence on K+ ion concentration and no or even negative cooperativity. Intentional increase in intermediate state population was also reported to broaden the range of K+ ion concentration detected by RNA G-quadruplex.45
Although several NMR and crystallography studies have been performed on DNA G-quadruplexes,46,47 only a few studies have been conducted on RNA G-quadruplexes. The NMR spectrum of an RNA quadruplex was first reported by the Uesugi group for RNA G-quadruplex sequence R14 (GGAGGUUUUGGAGG) present in mRNAs of immunoglobulin genes.48 The reported NMR structure elucidates the dimeric parallel quadruplex structure of R14 comprising two stacks of G-quartets. The same group further conducted comparative studies between R14 and D14 sequences and revealed that D14 forms an antiparallel DNA quadruplex with diagonal loops in contrast to R14′s parallel RNA quadruplex conformation with propeller loops.49 Solution based studies of NMR were further extended to the crystallography studies by Collie et al. where the 2′ hydroxyl group and rigid C3′-endo sugar puckering in RNA G-quadruplex were shown to redefine the hydration structure in grooves and hydrogen bonding patterns (Fig. 1C).50 Thereafter, the crystal structure of TERRA G-quadruplex–acridine small molecule complex was also accounted by the same group where the 2′ hydroxyl group of the ribose sugar was found to be involved in binding with acridine.51 All of the above mentioned NMR and crystallography studies have been performed on intermolecular RNA G-quadruplexes. However, no such studies have been conducted on intramolecular RNA G-quadruplexes yet.
In addition, a telomeric repeat containing RNA (TERRA) has been studied by electrospray ionisation mass spectroscopy (ESI-MS). These studies demonstrate the formation of higher order assemblies of RNA quadruplexes of variable lengths (12 mer, 22 mer, 45 mer) of human telomeric RNA sequences.52 This stacking nature of RNA quadruplexes is comparable with the “beads on a string” model for G-quadruplex assembly.53,54 RNase T1 digestion of TERRA generating a ladder of bands with size in integral multiple of 24 nucleotides further supported this model.55 Lately, NMR structure of TERRA also revealed 5′-5′ end stacking of two RNA G-quadruplexes.56
Function
While the main focus of research has been on DNA G-quadruplexes and their potential role in biology, in recent years the role of RNA G-quadruplexes as regulatory elements of gene expression is beginning to emerge (Table 1 and Fig. 3). In this regard, the initial emphasis was on G-quadruplexes located in the 5′ UTR of mRNA, which is known to be involved in translational regulation.
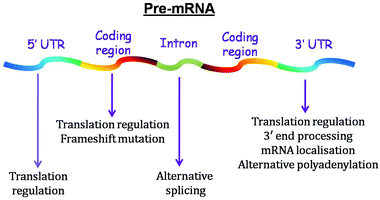 |
| Fig. 3 Functions of G-quadruplex located in different regions of pre-mRNA. | |
Table 1 Biological functions of RNA G-quadruplexes
Role |
mRNA |
Probable functions |
References |
1. Translational regulation |
NRAS |
G-quadruplex in the 5′ UTR of these cellular mRNAs represses translation. |
13
|
(a) Repression |
Human and bovine ESR |
|
60,61
|
|
Zic-1 |
|
57
|
|
BcL2 |
|
63
|
|
TRF-2 |
|
64
|
|
MT3-MMP |
|
62
|
|
ADAM-1 |
|
66
|
|
Cyclin D3 |
|
67
|
|
AKTIP |
|
72
|
|
CTSB |
|
72
|
|
Estrogen receptor |
G-quadruplex present in the ORF of this mRNA halts translational elongation. |
78,79
|
|
PIM1 |
G-quadruplex in the 3′ UTR of this cellular mRNA is associated with its role in translational repression. |
82
|
(b) Augmentation |
FGF-2 |
G-quadruplex is essential for IRES-A mediated cap-independent translation initiation. |
59
|
|
VEGF |
|
65
|
|
TGFβ2 |
G-quadruplex in the 5′ UTR of these cellular mRNAs increases translation. |
68
|
|
FOXE3 |
|
72
|
2. 3′ end processing |
IGF-II |
This transcript undergoes a specific cleavage reaction in vivo at a site just 5′ to G-quadruplex motif. |
12,85
|
|
p53 |
G-quadruplex downstream from the p53 pre-mRNA cleavage site is crucial for maintaining p53 3′ end processing efficiency following UV irradiation. |
86
|
Alternative polyadenylation |
LRP5 and FXR1 |
G-quadruplexes located in the 3′ UTR of these genes increases alternative polyadenylation efficiency generating several short transcripts. |
87
|
3. Transcription termination |
Control region-conserved sequence block II (CSB II) of mitochondria |
G-quadruplex helps in transcription termination and the mechanism is similar to Rho-independent transcription termination in prokaryotes. |
88
|
4. mRNA localisation |
PSD-95 and CaMKIIa |
G-quadruplexes in the 3′-UTR of these mRNA are neurite-targeting elements that aid transport of these mRNAs from soma to dendrites. |
89
|
5. Alternative splicing |
src N1 |
G-quadruplexes affect the splicing and gene expression by providing binding sites for proteins required for splicing such as hnRNP protein family. |
91
|
|
Tropomyosin |
|
92
|
|
hTERT |
|
93
|
|
FMR1 |
|
94
|
|
BACE1 |
|
96
|
|
PAX9 |
|
97
|
|
p53 |
G-quadruplex in intron 3 modulates the splicing of intron 2, leading to differential expression of transcripts encoding distinct p53 isoforms. |
95
|
Translational regulation
The presence of G-quadruplex structures in the 5′ UTR of cellular mRNAs is generally associated with its role in translational repression. The first example of translational repression by an RNA G-quadruplex was reported by the Balasubramanian group in the 5′ UTR of the gene transcript of the human NRAS (Neuroblastoma RAS) proto-oncogene.13 Using a cell-free translation reporter assay, they demonstrated that a thermodynamically stable G-quadruplex in NRAS mRNA inhibits gene expression. However, their studies were performed under in vitro conditions. Subsequently our group for the first time examined the role of RNA G-quadruplex present in the 5′ UTR of the human Zic-1 (Zinc finger of the cerebellum 1) zinc finger protein in eukaryotic cells using the dual luciferase reporter assay and reported its inhibitory role at the translational level.57
Genome-wide computational analysis revealed the presence of 4141 5′ UTR G-quadruplex motifs in 5′ UTR of mRNAs.58 Following NRAS and Zic-1, the functional relevance of this secondary structure has been validated experimentally in genes – FGF-2 (Fibroblast growth factor-2),59 NRAS,13 Zic-1,57 Exon C of human and bovine ESR (estrogen receptor α),60,61 MT3-MMP (Membrane-type 3 matrix metalloproteinase),62 Bcl2 (B-cell lymphoma 2),63 TRF-2 (Telomeric repeat-binding factor 2),64 VEGF (Vascular endothelial growth factor),65 ADAM-10 (Disintegrin and metalloproteinase domain-containing protein 10),66 cyclin D3
67 and TGFβ2 (Transforming growth factor β2).68 RNA G-quadruplex in the 5′ UTR of most of the aforesaid genes was shown to repress gene expression at the translational level.69 In most of the above studies and in a study by Halder et al. it was shown that G-quadruplex-forming sequences alone when present in 5′ UTRs exhibit translational repressive activity.70 Recently, transcriptome scale ribosomal profiling of helicase eIF4A-dependent transcripts revealed the enrichment of 5′ UTR sequences with propensity to form RNA G-quadruplexes, indicating the probable role of this factor in unwinding of this secondary structure during the translation process.71
Although most studies indicate the translational inhibitory role of the 5′ UTR G-quadruplex, few examples indicate its contrasting role. The first translational up-regulating role of RNA G-quadruplex was revealed in the 5′-UTR of mRNA associated with cap-independent translation. Bonnal et al. were pioneers in showing the gene -up-regulating role of G-quadruplex motif in the internal ribosome entry site (IRES) sequence.59 They reported that a G-quadruplex structure in conjunction with two stem-loop structures located within the FGF-2 IRES was required for translation initiation. Subsequently, Basu and coworkers showcased a unique example wherein a quadruplex is exclusively required for cap-independent translation initiation in human VEGF IRES.65 The authors here reported that the presence of multiple G tracts in IRES-A might direct initiation the process to depend on a conformationally flexible G-quadruplex switch toggling in response to a biological stimulus. They gave it a grade of a tunable translation initiator equivalent to a riboswitch, which generally changes conformation in response to metabolite binding. However, these studies highlighted RNA G-quadruplex role in IRES and not in cap dependent translation. In this direction, our group, for first time, established the activating role of RNA G-quadruplex in the 5′ UTR of TGFβ2 where it was demonstrated to augment gene expression.68 Subsequently, we also indicated the translational up-regulating role of this structure in the 5′ UTR of FOXE3 (Forkhead box E3).72
Furthermore, in order to show the gene regulatory role of G-quadruplexes, Wieland and Hartig designed and inserted a series of quadruplex forming sequences surrounding the Shine–Dalgarno sequence of a GFP reporter gene.73,74 They showed that these motifs readily fold in vivo and modulate gene expression if positioned within the ribosome binding site (RBS), thereby providing a novel way to interfere with translational initiation in bacteria. Moreover, even less stable G-quadruplexes composed of only two stacks of G tetrads was reported to exhibit pronounced gene regulating activity when placed at critical positions in the ribosome binding site.
Given the hypothesis that the position of G-quadruplex in 5′ UTR might affect its function, two independent groups shifted the position of G-quadruplex in 5′ UTR to understand the position specific effect of G-quadruplexes. Kumari et al. reported that shifting this motif in NRAS 5′ UTR to a position far from the cap region causes loss of its function75 whereas Huppert et al. demonstrated that this shift in mRNA does not change the function of RNA G-quadruplex.70 The difference in the outcome of the shift may be attributed to the different mRNAs or different experimental setups they employed for their studies. To further delineate the position specific role of G-quadruplex, our group assessed its role when located at the 5′ end of naturally occurring mRNAs and found that G-quadruplex exhibited varied functions in different genes.72 It inhibited gene expression in AKTIP (AKT interacting protein) and CTSB (Cathepsin B), but augmented protein production in FOXE3, suggesting that neighbouring nucleotide sequence, trans factors and intracellular conditions might affect the function of G-quadruplex. From the above studies, we hypothesise that the inhibitory G-quadruplex may itself act as a roadblock impeding the movement of a per-initiation complex or may recruit some inhibitory factors repressing mRNA translation (Fig. 4A and 4B). On the other hand, activating G-quadruplex may engage activating factors to augment gene expression (Fig. 4C). More studies are required to decipher the mechanistic understanding of G-quadruplexes in this direction.
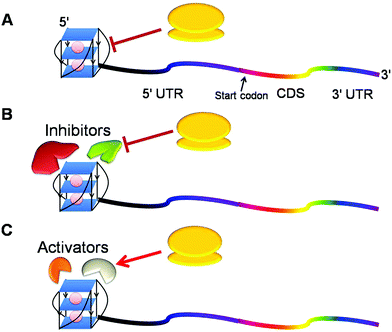 |
| Fig. 4 Probable mechanisms for regulation of gene expression by RNA G-quadruplex in the 5′ UTR of mRNA. (A) G-quadruplex itself may interfere with the recruitment of a preinitiation complex and subsequently delays the translation. (B) This structure may decoy inhibitors which in turn hinder the translation process. (C) G-quadruplex may employ factors that actively engage the translation machinery to translate mRNA into the protein. | |
RNA G-quadruplexes influence the gene expression not only via their location in UTRs but also in the coding sequence of mRNA. Upon evaluating the role of G-quadruplex in open reading frame (ORF), it was revealed that it suppresses translation and the extent of gene suppression depends on its stability.76,77 G-quadruplex present in the ORF of human estrogen receptor α was also shown to halt translational elongation and influence protein folding and proteolysis.78,79 Subsequently, ORF G-quadruplex was demonstrated to cause frameshift mutation of one nucleotide leading to the recoding process.80,81 In essence, these studies show that G-quadruplex can impede the process of translation at the elongation level despite the helicase activity of ribosome.
In contrast to 5′ UTR G-quadruplexes, very few studies have been performed to unravel the translational regulatory role of G-quadruplexes in the 3′ UTR of mRNAs. G-quadruplex in the proto-oncogene PIM1 3′ UTR was reported to cause a translational suppression, though the mechanism of suppression is unclear.82
While the above studies discussed upon the implication of intramolecular RNA G-quadruplexes on gene translation, the Ito et al. studies indicated an intermolecular RNA G-quadruplex influence upon the translational process. Using reporter systems, they demonstrated that G-rich RNA can bind G-rich sequences in UTRs or the coding region of mRNAs through RNA–RNA interaction forming an intermolecular G-quadruplex that can cause gene inhibition in cellulo conditions.83 In a similar line, a recent report by Basu and coworkers indicated that DNA oligonucleotide induced hybrid DNA: RNA G-quadruplex formation in the 5′ UTR and protein coding region of eIF4E mRNA causes reduced eIF4E protein production in human cancer cells.84 Essentially, these reports extended the concept of small nucleic acid mediated gene regulation that can occur through intermolecular structure formation in living cells.
3′ end processing and alternative polyadenylation
G-quadruplex function in physiology was initiated with the discovery of a G quadruplex structure downstream of an endonucleolytic cleavage site in the 3′ UTR of IGF II (insulin-like growth factor II) mRNA.12,85 Since this transcript undergoes a specific cleavage reaction at a site just 5′ to this motif, it suggested the role of G-quadruplex in targeting mRNA-processing events.85 Subsequently, G-quadruplex formation was found to assist mRNA 3′ end processing in p53 in the event of DNA damage (Fig. 5). Decorsiere et al. demonstrated that the presence of a stable G-quadruplex structure downstream from the p53 pre-mRNA cleavage site is critical for maintaining p53 3′ end processing efficiency following UV irradiation mediated DNA damage. This cis element via interactions with the hnRNP H/F protein maintains p53 expression and leads to apoptosis following DNA damage.86
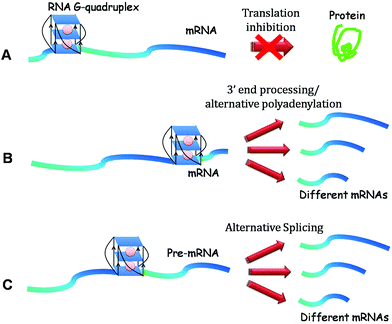 |
| Fig. 5 Functions of RNA G-quadruplexes. (A) Translation repression. (B) 3′ end processing and alternative polyadenylation when located in the 3′ UTR of mRNA. (C) Alternative splicing when present in introns. | |
Lately, G-quadruplexes located in the 3′ UTR of LRP5 (low-density lipoprotein receptor-related protein 5) and FXR1 (fragile X mental retardation 1) mRNAs were shown to increase the efficiency of alternative polyadenylation leading to the generation of several short transcripts. These short transcript productions were further implicated in impairing miRNA regulation of FXR1 mRNA.87
Mitochondrial transcription termination
The role of 3′ UTR G-quadruplexes has been further explored in the diverse areas of gene regulation. It has been involved in aiding transcriptional termination of mitochondrial light strands in a manner analogues to Rho-independent transcription termination in prokaryotes, where a G-quadruplex motif substitutes the hairpin loop formed in bacterial mRNA.88 It was also exhibited to generate primers for heavy strand DNA replication.
mRNA localisation
3′ UTR RNA G-quadruplexes have been shown to act as structural “zipcodes” assisting the process of mRNA localization in neurons.89 G quadruplexes in the 3′ UTR of two mRNAs – PSD-95 (postsynaptic density protein 95) and CaMKIIa (Calcium/calmodulin-dependent protein kinase IIa) – are required for their localisation from soma to dendrites of cortical neurites. Since these mRNAs were previously described as targets of FMRP, the authors demonstrated that FMRP depletion did not abolish the transport, thus negating the possibility of FMRP's role in ferrying the RNA to the neurites. Bioinformatic analyses further showed the presence of a putative G-quadruplex sequence in the 3′ UTR of 30% highly conserved dendritic mRNAs, suggesting that these neurite-targeting elements are important for the establishment and maintenance of cell polarity.89 More studies on these dendritic mRNAs as well as other mRNAs would reveal the mechanistic understanding of the zipcoding function of RNA G-quadruplex.
Alternative splicing
G-quadruplexes located in the intronic regions of mRNA also affect alternative splicing – a mechanism of producing multiple protein products from a limited number of genes. Trans elements namely proteins of the hnRNP family play crucial roles in splicing (Fig. 5). Genome wide studies indicated that G-rich tracts in the introns of many genes exhibit the potentiality to form G-quadruplexes and thus affect their splicing and expression patterns.90 The first report on the cis-regulatory element G-quadruplex role in alternative splicing demonstrated that hnRNP F binds to a G-rich tract in the pre-mRNA which could form a G-quadruplex structure and is essential in the splicing of the scr N1 exon.91 Subsequently, G-quadruplex mediated regulation of alternate splicing was demonstrated to occur in a number of physiologically important genes such as B-tropomyosin,92 hTERT (human telomerase reverse transcriptase),93 FMR1 (Fragile X mental retardation 1),94 p53,95 BACE-1 (beta-site APP-cleaving enzyme-1)96 and PAX9 (Paired box gene 9).97 Interestingly, alternative splicing was found to be different in p53 compared to the rest of the examples cited above, as the authors showed that G-quadruplex in p53 is located in another intron (intron 3) than the one affected by alternative splicing (intron 2). Additionally, they showed that G-quadruplex presence in p53 did not induce a complete shift from one splicing pattern to the other, but rather modified the equilibrium between the two spliced products in a subtle manner, leading to differential expression of transcripts encoding distinct p53 isoforms;95 further indicating the ability of G-quadruplex motifs to fine tune the levels of different isoforms. There is a need to explore the prevalence of this kind of splicing regulation in other genes as well.
Proteins interacting with RNA G-quadruplex
The number of RNA G-quadruplex binding proteins reported is relatively low in contrast to proteins known to bind to DNA G-quadruplex.98,99 Among them, FMRP is the most extensively studied RNA G-quadruplex binding protein.
FMRP
In recent years, the role of RNA G-quadruplex upon interacting with two RNA-binding proteins: FMRP (Fragile X Mental Retardation Protein) and FMR2P (Fragile X Mental Retardation 2 Protein) has been extensively studied.100–102 Impaired production of these proteins is associated with two forms of mental diseases: the Fragile X Mental Retardation syndrome (FXS) and the FRAXE-associated mental retardation (FRAXE).103
FMRP protein has been shown to bind RNA targets harboring a G quadruplex motif with high affinity and specificity via its arginine–glycine–glycine (RGG) box.101,104 RNA binding studies have revealed that an array of FMRP's RNA targets have G-quadruplexes in their 5′ UTR,105,106 coding regions107,108 and 3′ UTR.109,110 RNA targets of FMRP, particularly Microtubules Associated Protein 1b (MAP1B) and a catalytic subunit of Protein Phosphatase 2A (PP2Ac) evinced the presence of one or more G-quadruplex structures in their 5′ UTR.101,106 FMRP binds to these motifs in MAP1B mRNA and inhibits its translation during active synaptogenesis in neonatal brain development. In the absence of FMRP, increased MAP1B protein expression is shown to cause abnormally enhanced microtubule stability, which in turn interferes with the normal development of dendritic spines.105 FMRP also negatively regulates PP2Ac mRNA translation by binding with its 5′ UTR G-quadruplex. In the absence of FMRP, the expression of PP2Ac increases and thereby alters actin remodelling in fibroblast cell lines.106 Additionally, a report by Khateb et al. showed that the longer (CGG)n sequences in the 5′ UTR of FMR1 gene in FXS form G-quadruplex secondary structures, blocking the migration of the 40S subunit and thereby leading to impeded translation of FMR1 mRNA. Indeed, expression of the quadruplex disrupting proteins hnRNP A2 or CBFA was shown to alleviate this translational block.111
RNA targets of FMRP harbouring G-quadruplexes in their 3′ UTR – PSD95
110 and Sem3F (Semaphorin 3F),109,112 mRNAs have been reported.103 Melko et al. demonstrated that the association of Sem3F mRNAs with polyribosomes is decreased in the absence of FMRP, thereby leading to their reduced expression. Hence, they indirectly suggested that FMRP in this instance may act as a translational activator by sequestration or may influence mRNA stability.103
Interestingly, FMRP was found to regulate its own activity by binding to G-quadruplex present in the coding region of its mRNA and inhibiting its translation by a negative feedback loop.107 Likewise, FMRP binds to G-quadruplex in the coding region of APP (Amyloid Precursor Protein) and regulates its translation.108 Besides this modulation in gene expression, a G-quadruplex structure present in exon 15 of the FMR1 gene is depicted as essential for alternative splicing of exon 14,94 where it has been shown to be a potent exonic splicing enhancer.102
Helicase proteins
RHAU is a DEAH box containing RNA helicase that unwinds both RNA and DNA G-quadruplexes.113–117 Although the N-terminal region of the RHAU protein known as the RHAU specific motif (RSM) harbors G-quadruplex recognition ability, its helicase activity is found to require the full length protein to unfold G-quadruplex structure.118,119 This helicase remodels G-quadruplex located in the 5′ end of telomerase RNA and resolves G-quadruplex increasing the expression of the Yin Yang 1 gene.117,120 In contrast, the RHAU (DHX36) RNA helicase was recently reported to bind to G-quadruplex present in the 3′ UTR of PITX1 mRNA and suppress its expression.121 The authors further emphasised that these changes are brought about in association with argonaute-2 protein, a part of the RISC silencing complex involved in microRNA mediated gene regulation, indicating a novel role of RHAU in microRNA mediated gene regulation of G-quadruplex containing 3′ UTR in some mRNAs. Another helicase DHX9 has also been reported to unwind RNA and DNA G-quadruplexes.122 This increasing repertoire of G-quadruplex resolving helicases would likely increase our overall understanding of the mechanism of unfolding and the maintenance of equilibrium between folded and unfolded states of this structure.
Other proteins
Hexanucleotide repeat expansion (HRE), (GGGGCC)n in the non-coding region of C9orf72 is associated with neurodegenerative diseases – amyotrophic lateral sclerosis (ALS) and frontotemporal dementia (FTD). This HRE can form DNA G-quadruplexes and promote the transcriptionally induced RNA–DNA hybrid called R-loop formation.123 An increase in the number of repeats in HRE under pathological conditions displays increased structural polymorphism causing increased transcriptional pauses, thereby leading to accumulation of abortive transcripts containing HRE. These transcripts forming RNA G-quadruplexes were found to entrap several ribonucleoproteins such as nucleolin leading to nucleolar stress and molecular cascades culminating in ALS and FTD pathologies.123 Recently, these transcripts were shown to undergo repeat associated non-ATG translation generating C9RAN proteins which are specifically detected in the cerebrospinal fluid of ALS patients, providing it a status of a biomarker of this disease.124
In addition, TLS/FUS – a telomere binding protein – has been shown to bind telomeric DNA G-quadruplex and TERRA RNA G-quadruplex via its RGG domain to form a ternary complex. As a matter of fact, these G-quadruplexes have been illustrated to serve as a scaffold for recruitment of these proteins to telomere, thereby regulating the telomere length by histone modifications of the telomere.125
Furthermore, the Frantz and Gilbert group purified and characterized two activities from yeast, G4p1 and G4p2 and found that both these activities bind G-quadruplex structures.126,127 Subsequently, Xenopus Pat 1 proteins were also demonstrated to bind RNA G-quadruplexes.128
Recently, pull down studies followed by matrix assisted laser desorption ionisation time of flight mass spectroscopy (MALDI-TOF MS) were utilised to identify the interacting partners of G-quadruplex located in the 5′ UTR of MMP6 and ARPC2 (actin-related protein 2/3 complex subunit 2) mRNAs.129 Binding partners were found to predominantly comprise ribosomal proteins, heterogeneous nuclear ribonucleoproteins, nucleolin and splicing factors. Few novel proteins previously unknown to bind nucleic acids were also identified.129 However, the mode of action of these proteins was not elucidated in these studies.
Telomeric RNA G-quadruplexes
In biological systems, functions of G-quadruplexes were first elucidated in the context of telomeric regions of the chromosomes, where the highest genomic prevalence of this secondary structure occurs.130 The telomeric regions (2–10 kb) of mammalian chromosomes consist of tandem repeats of the sequence d(TTAGGG) and a G-rich strand that forms 100–200 nucleotide long 3′ overhang called a G-tail. Telomeres and their associated enzyme telomerase are vital elements for the maintenance of chromosomal end integrity. A protein complex shelterin further upholds the integrity of telomeric ends.131 Dynamic resolution and formation of G-quadruplex structures in tandem repeats of the sequence d(TTAGGG) were proposed to aid chromosomal end maintenance.132 Since these telomeric G-quadruplexes are implicated in cancer biology, considerable research effort has been focused on therapeutic implications and targeting of such structures.9
Initially, the telomeric region was thought to be transcriptionally silent, but a landmark study in 2007 provided unequivocal evidence that the C-rich strand of the telomeric DNA is in fact actively transcribed by DNA-dependent RNA polymerase II (Pol II), giving rise to transcripts of variable lengths (0.1–9.0 kb) known as telomeric repeats containing RNA (TERRA or telRNA).133 TERRA was observed to exclusively localize to the nucleus near the telomeric regions.134,135 It has been identified in humans, mice, zebrafish and yeast, indicating its evolutionary conservation and hinting towards its probable important role within cells.133,136,137 These long non-coding RNAs are involved in a number of key cellular processes, including regulation of the telomere length and inhibition of telomerase.138,139
Since TERRA consists of consecutive repeats of UUAGGG, extensive studies were carried out to assess G-quadruplex formation in these telomeric RNAs.52 These studies evinced that TERRA RNA could form stable G-quadruplexes and further evidence of their in vivo existence provided clues about their unknown roles in telomere biology.135 Employing pull down studies and MALDI-TOF MS, a wide array of proteins has been identified to bind TERRA RNA.140,141 These proteins were deduced to regulate the localization and local concentration of the TERRA RNA. The shelterin proteins TRF1 and TRF2 were shown to be capable of binding directly to TERRA, as well as to elements of the origin recognition complex and H3TK9. In particular, TRF2 binds simultaneously to both TERRA RNA G-quadruplex and telomeric DNA G-quadruplex.142 Moreover, proteins belonging to the hnRNP class were also found enriched in the pool of binding partners. Pull down studies with a poly UUAGGG tract indicated binding of hnRNP A1 to G-quadruplex structure.141 Thus, these reports make protein binding and thus function mediated via the formation of a G-quadruplex structure in TERRA plausible.
TERRA provides an excellent example of a non-coding RNA that is involved in maintenance of chromosomal end integrity by most probably acting as a scaffold for recruiting proteins. With growing literature on long non-coding RNA with unknown functions and bioinformatic prediction of G-quadruplex in non-coding RNAs,14 we speculate that non-coding RNA harbouring G-quadruplexes might be a more universal phenomenon with probable important functions.
Tools for detecting RNA G-quadruplexes
G-quadruplexes are detected by biophysical techniques such as UV-vis spectroscopy, Circular Dichroism (CD), fluorescence spectroscopy, single molecule FRET spectroscopy and electrospray ionisation mass spectroscopy.52 Biophysical characterisation of G-rich sequences is performed in buffer containing potassium/sodium salts. A hypochromic shift with increasing temperature at 295 nm is a characteristic signature of G-quadruplex during UV melting.143 CD scans of RNA G-quadruplex show a positive peak at 262 nm and a negative peak at 240 nm of wavelength, indicating its parallel topology. The thermal difference spectrum of G-quadruplex where the differential absorbance of the structure under unfolded and folded conditions is plotted against wavelength indicates two positive peaks and a negative peak (at 295 nm).144 A fluorescence resonance energy transfer study of G-quadruplex forming sequence with a pair of acceptor/donor fluorophores covalently attached to the 5′ and 3′ ends is also used.41 Besides biophysical characterisation, polyacrylamide gel electrophoresis, reverse transcription assay and footprinting analysis of the sequences are employed.44,88 As the RNase T1 enzyme cleaves after guanine residue under the single stranded conditions and G-quadruplex secondary structure confers protection to the guanine residues involved against RNase T1 digestion, the RNase T1 footprinting technique is widely used.62 DMS footprinting and in line probing are also performed to indicate the guanine residues involved in G-quadruplex formation.59,69 Furthermore, to detect the folding topologies of G-quadruplex at the atomic level, techniques such as NMR spectroscopy and crystallography are employed.48,50 These techniques have been discussed in detail elsewhere.145
In order to show the existence and function of G-quadruplexes in cells, various controls are included in the experiments. For example, G-quadruplex sequences with point mutations that disrupt G-quadruplex formation are used as control to elucidate its function.13,57,89 Also proteins and small molecule known to bind these structures are used to unravel their role; they are discussed in detail in the next section. Recently, Balasubramanian and co-workers developed a single chain fragment antibody for visualisation of RNA G-quadruplex in human cells.22 However, more probe molecules to identify and study this structure in cells are highly required.
Targeting RNA G-quadruplex
Manipulation of cellular events by targeting the nucleic acid secondary structure is a promising strategy to control gene expression.99,145 Oligonucleotide based targeting agents interfere with gene function but exhibit poor pharmacological properties.146 Small molecule based intervention methodology overcomes the disadvantages associated with nucleic acid based approaches, thereby translating chemical biology towards developing therapeutics.147,148
As G-quadruplex at the telomeric ends and promoter regions are well established targets for cancer therapeutics, extensive research on the ligands targeting DNA G-quadruplex has been performed in the last few decades.149 Small molecules such as porphyrin (TmPyP4), acridine, pentacridium, quinacridine, telomestatin, naphthalene diiamide, bisquinolium and their derivatives are found to selectively bind and stabilise DNA G-quadruplex.150,151 Various crystal structures of DNA G-quadruplex–small molecule are reported.150 Even a few of the above ligands have been tested to bind and modulate DNA G-quadruplex function under cellular conditions.151
Small molecules synthesised to selectively bind to G-quadruplex typically exhibit the following features: (1) a large planar aromatic core in order to maximise π-stacking interactions with G-quartets of G-quadruplex. (2) A positive charge to neutralise the negatively charged phosphate groups of the nucleic acid backbone. (3) Positive charged side chains with functional groups to enhance contacts with grooves/loops/inner G-quartet core of G-quadruplex.145 However till date, only a few studies on ligand targeting RNA G-quadruplex have been conducted.152 Since various clinically important genes are known to harbour RNA G-quadruplexes, designing small molecules that stabilise or destabilise this structure acts as an attractive strategy towards drug discovery against various diseases including cancer.145 In particular, G-quadruplexes in telomeric RNA containing the repeat (TERRA) transcribed from the subtelomeric loci present at chromosomal ends and the untranslated region of the mRNA of oncogenes are plausibly the most suitable targets for drug discovery. Moreover, the parallel topology of RNA G-quadruplexes limits its conformational diversity, making selective targeting less challenging.152
Initially, Collie et al. studied the selectivity of few small molecules against DNA and RNA G-quadruplexes and found that BRACO-19 was more selective for the telomeric DNA G-quadruplex and a naphthalene diamide derivative was more selective towards RNA G-quadruplex.153 Thereafter, the same group reported the crystal structure of TERRA G-quadruplex–acridine small molecule complex where increased involvement of the 2′ hydroxyl group of the ribose sugar in binding with acridine was revealed (Fig. 6).51 In contrast to DNA G-quadruplex–acridine complex, the loops of RNA G-quadruplex were shown to interact with acridine. To date, this has been the only crystal structure of the ligand–RNA G-quadruplex complex available. Nevertheless, ESI mass spectroscopy has also been employed to understand the binding behaviour of three natural alkaloids (nitidine, palmatine, and jatrorrizine) to RNA G-quadruplex structure present in the 5′ UTR of Bcl2. They are found to bind the Bcl2 RNA quadruplex with high affinity and binding stoichiometry ranging from 1
:
1 to 3
:
1.154 ESI-TOF-MS experiments also demonstrated that chelerythrine can bind both DNA and RNA G-quadruplexes.155 However, no NMR or crystallography studies have been performed on the mRNA G-quadruplex.
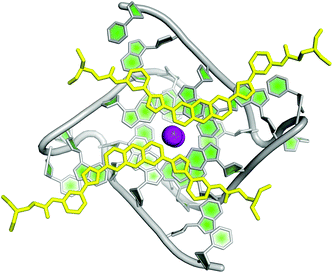 |
| Fig. 6 Crystal structure of RNA G-quadruplex-acridine complex (PDB ID – 3MIJ).51 The grey, dark green and light green represent the phosphate backbone, ribose sugar and nitrogenous bases of RNA oligonucleotides, respectively. The acridine molecules N,N′-((1,10-(acridine-3,6-diyl)bis(1H-1,2,3-triazole-4,1-diyl))bis(3,1-phenylene))bis-(2-(diethylamino)acetamide) are shown in yellow colour. Potassium ions (K+) are depicted in violet colour. | |
Pyridostatin is a known molecule to stack over the terminal G-quartets of DNA G-quadruplex stabilising the structure and to cause growth arrest in cancer cells by inducing extensive DNA damage.156 Through a template-directed in situ ‘click chemistry’ approach, a derivative of this molecule – carboxypyridostatin – was discovered to exhibit more binding preference and selectivity towards RNA G-quadruplex over DNA G-quadruplex.157 In fact, this selectivity of carboxypyridostatin was later exploited in conjunction with G-quadruplex structure specific antibody probe for the direct visualisation of RNA G-quadruplexes in the cytoplasm of the cell.22 Recently, Basu and co-workers screened a library of RNA G-quadruplex forming sequences with varying loop lengths for their binding to aminoglycoside kanamycin A.158 They found that only a few RNA G-quadruplexes with two nucleotide loop lengths with specific combinations were able to bind to kanamycin A.
Ligands binding to G-quadruplex can behave in varying manners: they can (a) stabilise G-quadruplex, thereby intensifying its function, (b) destabilise the structure, thus minimising its role and (c) interfere with the binding of trans factor to G-quadruplex, consequently hindering its activity (Fig. 7).152 In this regard, Balasubramanian and coworkers for the first time demonstrated the proof of concept for small molecule mediated gene regulation. They showed that small molecule – bisquinolium derivatives RR82 and RR110 – are potent binders and stabilisers of RNA G-quadruplex present in the 5′ UTR of NRAS mRNA, enhancing inhibition of NRAS gene expression.148 In addition, three bisquinolium derivatives – PhenDC3, PhenDC6 and 360A with binding affinity similar to RNA G-quadruplex and DNA G-quadruplex were found to perform small molecule–RNA G-quadruplex mediated gene regulation.63,64,81,95,159 The Balasubramanian group also showed that ligand TmPyP4 does not exhibit RNA G-quadruplex selectivity and thereby, does not inhibit NRAS translation.148 In contrast, Basu and coworkers demonstrated that TmPyP4 destabilises RNA G-quadruplex present in the 5′ UTR of MT3-MMP gene, relieving the suppressive role of RNA G-quadruplex. Recently, this molecule was shown to distort RNA G-quadruplex structures formed by r(GGGGCC)n repeats of the C9orf72 gene interrupting their interaction with various proteins.160 Moreover, a photoactivable alkyl substituted TmPyP4 was developed to bind the KRAS G-quadruplex and cause mRNA degradation and translation suppression upon photoactivation.161 TmPyP4 tetrachloride was further demonstrated to bind the TERRA RNA G-quadruplex with binding affinity of a magnitude one order higher than that of the telomeric DNA G-quadruplex using ESI-TOF-MS.162 These studies, thus, indicated that a molecule against RNA G-quadruplex such as TmPyP4 can behave differently in different gene contexts.
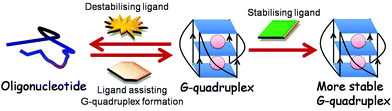 |
| Fig. 7 A ligand can stabilise, destabilise RNA G-quadruplex or can aid RNA G-quadruplex formation from oligonucleotide. | |
Employing robust approaches to identify ligands for RNA G-quadruplexes is of great interest from a drug discovery point of view.163 Various high throughput screening platforms such as virtual docking164–166 and fluorescence resonance energy transfer (FRET) based methods167–169 are used for identifying the binders. However, these screening were majorly utilised to identify molecules against DNA G-quadruplexes. Lacroix et al. used a fluorescence based approach and quadruplex–duplex competition to screen various compounds and identified selective RNA G-quadruplex binders.170 Recently, Garavis et al. used 19F-NMR fragment based screening of various compounds to identify ligands that can bind with TERRA RNA G-quadruplex with high selectivity.164 Some of these RNA G-quadruplex ligands identified were also observed to bind DNA G-quadruplexes with parallel topology.
Template assisted synthetic G-quartets (TASQs) are prototype G-quadruplex ligands that do not fall into the category of “small molecules having planar aromatic rings”. These TASQs undergo conformational rearrangement upon binding to G-quadruplex, ensuring a high level of selectivity.171 A deviation of this prototype, pyrene template-assisted synthetic G-quartets (PyroTASQ) ligand was recently reported to emit fluorescence upon binding to DNA/RNA G-quadruplex.172
Current research on ligands is mainly restricted to small molecules having planar aromatic rings that primarily undergo extensive π-stacking interactions with the end G-quartets of G-quadruplex.5 There is a requirement to explore ligands that can bind to grooves, loops and other regions of G-quadruplex. As proteins are known to bind G-quadruplex, another line of research has led to advancements towards developing peptides and antibodies towards this secondary structure. Peptides provide a good alternative to the generally used heterocyclic molecules that stack with terminal G-quartets of DNA G-quadruplex. Peptides with amino groups exhibit potential to interact with loops, grooves and the phosphate backbone of G-quadruplex structure. The prime peptide developed against this structure was a single chain antibody fragment that recognises DNA G-quadruplex structures formed by telomeric repeats and was employed to show in vivo evidence of G-quadruplex structures within the macronucleus of Stylonychia lemnae for the first time.173 Recently, Balasubramanian and coworkers have identified a single chain fragment selective for G-quadruplex using the peptide display library and have used them as a valuable visual tool to demonstrate the presence of both DNA and RNA G-quadruplex in the human cells (Fig. 8).22,174 In another study, chemically modified peptides with zinc finger motifs were identified as suitable ligands for G-quadruplex on screening the phage display library.175 These peptides were shown to inhibit telomerase activity when administered in the nanomolar range.176
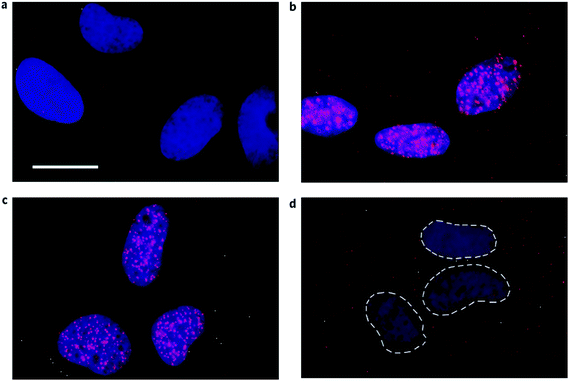 |
| Fig. 8 Visual identification of RNA G-quadruplex in the human cells. G-quadruplex specific antibody BG4 (shown in red) was used to detect RNA G-quadruplex in the cytoplasm of SV40-transformed MRC-5 fibroblast using indirect immunofluorescence microscopy. Nucleus is stained with DAPI and the cytoplasm is seen as a dark region around the nuclei. (a) No primary antibody (b) BG4 staining (red) shows the presence of G-quadruplex in both nucleus and cytoplasm (c) Loss of BG4 staining in the cytoplasm after RNase A treatment (d) Loss of BG4 staining in the nucleus after DNase treatment. Reprinted with the permission from Macmillan Publishers Ltd: [Nature Chemistry],22 copyright (2014). | |
Besides this, a few other peptides were identified to bind G-quadruplex structures. Human antimicrobial peptide – cathelicidin peptide LL37 – was reported as a potent binder of DNA G-quadruplex structure.177 In addition, the RGG (arginine–glycine–glycine) domain of TLS protein has been demonstrated to bind to both telomeric RNA and DNA G-quadruplexes.125 Interestingly, on substituting tyrosine for phenylalanine in the RGG domain of the TLS protein, the binding specificity of the engineered RGG domain was found to greatly increase for RNA G-quadruplex and drastically decrease for DNA G-quadruplex.178 Indeed, this report is a paradigm where modifications incorporated in G-quadruplex specific peptide are shown to alter its affinity to either RNA or DNA G-quadruplex. Thus lately, although there has been emerging interest in using RNA G-quadruplex as a potential molecular target for small molecule therapeutic agents and a few molecules have been developed in this direction, most of the compounds identified against RNA G-quadruplex have resulted from rational modification of the molecules already tested against DNA G-quadruplexes. Therefore, the major challenge remains to identify/design novel ligands against RNA G-quadruplexes. Novel ligands may be developed with a careful understanding of the inherent differences between DNA and RNA G-quadruplex structures. Exploiting the effects of the ribose hydroxyl group on RNA G-quadruplex structure can form the basis for the ligand design for discerning the differences between DNA and RNA G-quadruplexes.179
Applications
Various G-quadruplexes forming oligonucleotides functioning as aptamers are being investigated for targeting molecules of therapeutic importance. Compared to monoclonal antibodies and other targeting tools, G-quadruplex aptamers offer several advantages of non-immunogenicity, heat stability, biostability, cost effectiveness, ease of chemical synthesis, enhanced cellular uptake efficiency and flexibility for introducing chemical modifications. In this regard, the most extensive work has been performed on RNA G-quadruplex based aptamers against prion protein, the agent causing transmissible neurodegenerative encephalopathy known as Creutzfeldt-Jakob disease.180 This aptamer targeting the N-terminal region of prion protein (PrPc) comprises G-quadruplex structure, and substitution of guanine bases with uracil residues was revealed to result in complete loss of PrPc binding, strongly suggesting that G-quadruplex is indispensible for PrPc recognition.181,182
In addition, numerous G-quadruplex based RNA aptamers have been identified against other pathological diseases. In the process of developing an aptamer against rheumatoid arthritis using SELEX, a G-quadruplex based RNA aptamer highly selective against human receptor activator of NF-κB (RANK) was identified.183 This aptamer was also found to target other TNF receptor family proteins, such as TRAIL-R2, CD30, NGFR and osteoprotegerin, a decoy receptor for RANK. Furthermore, an aptamer had been successfully developed as a trypanocidal agent to treat sleeping sickness.184 This 2′-NH2 modified aptamer possessing a stable G-quadruplex directly binds live trypanosomes at the parasite surface, thereby targeting it for immunoglobulin attack. In addition, several G-rich sequences were identified during in vitro screening of RNA sequences targeting the HIV integrase enzyme.185 Most of these sequences harboured the motif capable of forming a stable G-quadruplex structure.
Furthermore, G-quadruplex based aptamers are being developed to bind with important biomolecules and cofactors of metabolic pathways such as riboflavin186 and thyroxine.187 Recently, RNA aptamers have also been used as diagnostic tools for the detection of SARS coronavirus.188 G-quadruplex in the spinach RNA aptamer also has been shown to bind the GFP like ligand and activates its fluorescence, suggesting its use in imaging techniques.189,190 RNA G-quadruplex has already been used as a thermoregulator to detect changes in temperature in E.coli. Like DNA G-quadruplex, it exhibits traits required for its use as a biosensor, molecular beacon, nanoswitch, nanowire and nanodevice191–194 and has become an important module in the field of synthetic biology, which has been discussed in detail elsewhere.195
Conclusion
With a large number of RNA G-quadruplexes present across the transcriptome, it becomes imperative to understand the biological importance of these structural motifs. Although the progress of research executed in this arena is highly commendable, much remains to be explored. The mechanism of G-quadruplex mediated gene regulation requires special attention. Recent progress in unravelling the function of RNA G-quadruplex has projected it as a suitable candidate for therapeutic intervention with considerable effort being invested in manipulating cellular events by strategically targeting this secondary structure.145,152 In this regard, optimization of suitable drug candidates that can interact selectively with RNA quadruplexes is of utmost importance.99 An array of ligands targeting DNA quadruplexes may be screened for suitable interactions with RNA counterparts and novel ligands may be designed to selectively interact with RNA G-quadruplexes. Though a few ligands have been identified, a better understanding of the thermodynamic basis of these studied interactions would further open a new avenue for developing improved therapeutic molecules targeting these secondary structures. Moreover, understanding the structural contribution of the versatile G-quadruplex to physiological functioning remains an unrevealed in vivo scenario. More animal model studies are required in this direction. Along with fundamentals and their therapeutic application in the form of aptamers, RNA G-quadruplex research possesses the potential to contribute immensely to the field of synthetic biology. We envision that increased availability of high-resolution structures of RNA G-quadruplexes in concert with an increased understanding of in vivo G-quadruplex topologies will expand the scope of identification of G-quadruplex ligands and their utility in the future.
Acknowledgements
This work was financially supported by the BSC0123 project (project: Genome Dynamics in Cellular Organization, Differentiation and Enantiostasis) from the Council of Scientific and Industrial Research (CSIR), Government of India. The authors would also like to thank Dr Mary Krishna Ekka for modelling crystal structures of RNA G-quadruplex.
References
- F. W. Smith and J. Feigon, Nature, 1992, 356, 164 CrossRef CAS PubMed.
- J. T. Davis, Angew. Chem., Int. Ed., 2004, 43, 668 CrossRef CAS PubMed.
- M. Gellert, M. N. Lipsett and D. R. Davies, Proc. Natl. Acad. Sci. U. S. A., 1962, 48, 2013 CrossRef CAS.
- M. A. Keniry, Biopolymers, 2000, 56, 123 CrossRef CAS.
- D. J. Patel, A. T. Phan and V. Kuryavyi, Nucleic Acids Res., 2007, 35, 7429 CrossRef CAS PubMed.
- C. C. Hardin, T. Watson, M. Corregan and C. Bailey, Biochemistry, 1992, 31, 833 CrossRef CAS.
- S. Burge, G. N. Parkinson, P. Hazel, A. K. Todd and S. Neidle, Nucleic Acids Res., 2006, 34, 5402 CrossRef CAS PubMed.
- T. Simonsson, Biol. Chem., 2001, 382, 621 CrossRef CAS PubMed.
- D. Monchaud and M. P. Teulade-Fichou, Org. Biomol. Chem., 2008, 6, 627 CAS.
- M. C. Nielsen and T. Ulven, Curr. Med. Chem., 2010, 17, 3438 CrossRef CAS.
- J. Kim, C. Cheong and P. B. Moore, Nature, 1991, 351, 331 CrossRef CAS PubMed.
- J. Christiansen, M. Kofod and F. C. Nielsen, Nucleic Acids Res., 1994, 22, 5709 CrossRef CAS PubMed.
- S. Kumari, A. Bugaut, J. L. Huppert and S. Balasubramanian, Nat. Chem. Biol., 2007, 3, 218 CrossRef CAS PubMed.
- G. G. Jayaraj, S. Pandey, V. Scaria and S. Maiti, RNA Biol., 2012, 9, 81 CrossRef CAS PubMed.
- S. Millevoi, H. Moine and S. Vagner, Wiley Interdiscip Rev. RNA, 2012, 3, 495 CrossRef CAS PubMed.
- X. Ji, H. Sun, H. Zhou, J. Xiang, Y. Tang and C. Zhao, Nucleic Acid Ther., 2011, 21, 185 CrossRef CAS PubMed.
- P. Svoboda and A. Di Cara, Cell Mol. Life Sci., 2006, 63, 901 CrossRef CAS PubMed.
- A. Jambhekar and J. L. Derisi, RNA, 2007, 13, 625 CrossRef CAS PubMed.
- P. C. Bevilacqua and J. M. Blose, Annu. Rev. Phys. Chem., 2008, 59, 79 CrossRef CAS PubMed.
- C. J. McManus and B. R. Graveley, Curr. Opin. Genet. Dev., 2011, 21, 373 CrossRef CAS PubMed.
- O. Kikin, Z. Zappala, L. D'Antonio and P. S. Bagga, Nucleic Acids Res., 2008, 36, D141 CrossRef CAS PubMed.
- G. Biffi, M. Di Antonio, D. Tannahill and S. Balasubramanian, Nat. Chem., 2014, 6, 75 CrossRef CAS PubMed.
- A. Joachimi, A. Benz and J. S. Hartig, Bioorg. Med. Chem., 2009, 17, 6811 CrossRef CAS PubMed.
- A. Arora and S. Maiti, J. Phys. Chem. B, 2009, 113, 10515 CrossRef CAS PubMed.
- C. F. Tang and R. H. Shafer, J. Am. Chem. Soc., 2006, 128, 5966 CrossRef CAS PubMed.
- D. Miyoshi, A. Nakao and N. Sugimoto, Biochemistry, 2002, 41, 15017 CrossRef CAS PubMed.
- D. Miyoshi, H. Karimata and N. Sugimoto, J. Am. Chem. Soc., 2006, 128, 7957 CrossRef CAS PubMed.
- D. H. Zhang, T. Fujimoto, S. Saxena, H. Q. Yu, D. Miyoshi and N. Sugimoto, Biochemistry, 2010, 49, 4554 CrossRef CAS PubMed.
- B. Sacca, L. Lacroix and J. L. Mergny, Nucleic Acids Res., 2005, 33, 1182 CrossRef CAS PubMed.
- C. M. Olsen and L. A. Marky, J. Phys. Chem. B, 2009, 113, 9 CrossRef CAS.
- P. Hazel, J. Huppert, S. Balasubramanian and S. Neidle, J. Am. Chem. Soc., 2004, 126, 16405 CrossRef CAS PubMed.
- A. Risitano and K. R. Fox, Nucleic Acids Res., 2004, 32, 2598 CrossRef CAS PubMed.
- M. Cevec and J. Plavec, Biochemistry, 2005, 44, 15238 CrossRef CAS PubMed.
- N. Kumar, B. Sahoo, K. A. Varun and S. Maiti, Nucleic Acids Res., 2008, 36, 4433 CrossRef CAS PubMed.
- A. Guedin, A. De Cian, J. Gros, L. Lacroix and J. L. Mergny, Biochimie, 2008, 90, 686 CrossRef CAS PubMed.
- A. Arora and S. Maiti, J. Phys. Chem. B, 2009, 113, 8784 CrossRef CAS PubMed.
- A. Guedin, J. Gros, P. Alberti and J. L. Mergny, Nucleic Acids Res., 2010, 38, 7858 CrossRef CAS PubMed.
- A. Y. Zhang, A. Bugaut and S. Balasubramanian, Biochemistry, 2011, 50, 7251 CrossRef CAS PubMed.
- S. Pandey, P. Agarwala and S. Maiti, J. Phys. Chem. B, 2013, 117, 6896 CrossRef CAS PubMed.
- M. Tomasko, M. Vorlickova and J. Sagi, Biochimie, 2009, 91, 171 CrossRef CAS PubMed.
- J. Y. Lee and D. S. Kim, Nucleic Acids Res., 2009, 37, 3625 CrossRef CAS PubMed.
- P. Agarwala, S. Kumar, S. Pandey and S. Maiti, J. Phys. Chem. B, 2015, 119, 4617 CrossRef CAS PubMed.
- N. V. Hud, F. W. Smith, F. A. Anet and J. Feigon, Biochemistry, 1996, 35, 15383 CrossRef CAS PubMed.
- M. A. Mullen, S. M. Assmann and P. C. Bevilacqua, J. Am. Chem. Soc., 2012, 134, 812 CrossRef CAS PubMed.
- C. K. Kwok, M. E. Sherlock and P. C. Bevilacqua, Angew. Chem., Int. Ed., 2013, 52, 683 CrossRef CAS PubMed.
- C. Kang, X. Zhang, R. Ratliff, R. Moyzis and A. Rich, Nature, 1992, 356, 126 CrossRef CAS PubMed.
- G. N. Parkinson, M. P. Lee and S. Neidle, Nature, 2002, 417, 876 CrossRef CAS PubMed.
- H. Liu, M. Kanagawa, A. Matsugami, Y. Tanaka, M. Katahira and S. Uesugi, Nucleic Acids Symp. Ser., 2000, 65 CrossRef.
- H. Liu, A. Kugimiya, T. Sakurai, M. Katahira and S. Uesugi, Nucleosides, Nucleotides Nucleic Acids, 2002, 21, 785 CAS.
- G. W. Collie, S. M. Haider, S. Neidle and G. N. Parkinson, Nucleic Acids Res., 2010, 38, 5569 CrossRef CAS PubMed.
- G. W. Collie, S. Sparapani, G. N. Parkinson and S. Neidle, J. Am. Chem. Soc., 2011, 133, 2721 CrossRef CAS PubMed.
- G. W. Collie, G. N. Parkinson, S. Neidle, F. Rosu, E. De Pauw and V. Gabelica, J. Am. Chem. Soc., 2010, 132, 9328 CrossRef CAS PubMed.
- H. Martadinata and A. T. Phan, J. Am. Chem. Soc., 2009, 131, 2570 CrossRef CAS PubMed.
- L. Petraccone, Top. Curr. Chem., 2013, 330, 23 CrossRef CAS.
- A. Randall and J. D. Griffith, J. Biol. Chem., 2009, 284, 13980 CrossRef CAS PubMed.
- H. Martadinata and A. T. Phan, Biochemistry, 2013, 52, 2176 CrossRef CAS PubMed.
- A. Arora, M. Dutkiewicz, V. Scaria, M. Hariharan, S. Maiti and J. Kurreck, RNA, 2008, 14, 1290 CrossRef CAS PubMed.
- J. L. Huppert, A. Bugaut, S. Kumari and S. Balasubramanian, Nucleic Acids Res., 2008, 36, 6260 CrossRef CAS PubMed.
- S. Bonnal, C. Schaeffer, L. Creancier, S. Clamens, H. Moine, A. C. Prats and S. Vagner, J. Biol. Chem., 2003, 278, 39330 CrossRef CAS PubMed.
- K. Derecka, G. D. Balkwill, T. P. Garner, C. Hodgman, A. P. Flint and M. S. Searle, Biochemistry, 2010, 49, 7625 CrossRef CAS PubMed.
- G. D. Balkwill, K. Derecka, T. P. Garner, C. Hodgman, A. P. Flint and M. S. Searle, Biochemistry, 2009, 48, 11487 CrossRef CAS PubMed.
- M. J. Morris and S. Basu, Biochemistry, 2009, 48, 5313 CrossRef CAS PubMed.
- R. Shahid, A. Bugaut and S. Balasubramanian, Biochemistry, 2010, 49, 8300 CrossRef CAS PubMed.
- D. Gomez, A. Guedin, J. L. Mergny, B. Salles, J. F. Riou, M. P. Teulade-Fichou and P. Calsou, Nucleic Acids Res., 2010, 38, 7187 CrossRef CAS PubMed.
- M. J. Morris, Y. Negishi, C. Pazsint, J. D. Schonhoft and S. Basu, J. Am. Chem. Soc., 2010, 132, 17831 CrossRef CAS PubMed.
- S. Lammich, F. Kamp, J. Wagner, B. Nuscher, S. Zilow, A. K. Ludwig, M. Willem and C. Haass, J. Biol. Chem., 2011, 286, 45063 CrossRef CAS PubMed.
- H. Y. Weng, H. L. Huang, P. P. Zhao, H. Zhou and L. H. Qu, RNA Biol., 2012, 9, 1099 CrossRef CAS PubMed.
- P. Agarwala, S. Pandey, K. Mapa and S. Maiti, Biochemistry, 2013, 52, 1528 CrossRef CAS PubMed.
- J. D. Beaudoin and J. P. Perreault, Nucleic Acids Res., 2010, 38, 7022 CrossRef CAS PubMed.
- K. Halder, M. Wieland and J. S. Hartig, Nucleic Acids Res., 2009, 37, 6811 CrossRef CAS PubMed.
- A. L. Wolfe, K. Singh, Y. Zhong, P. Drewe, V. K. Rajasekhar, V. R. Sanghvi, K. J. Mavrakis, M. Jiang, J. E. Roderick, J. Van der Meulen, J. H. Schatz, C. M. Rodrigo, C. Zhao, P. Rondou, E. de Stanchina, J. Teruya-Feldstein, M. A. Kelliher, F. Speleman, J. A. Porco, J. Pelletier, G. Ratsch and H. G. Wendel, Nature, 2014, 513, 65 CrossRef CAS PubMed.
- P. Agarwala, S. Pandey and S. Maiti, Biochim. Biophys. Acta, 2014, 1840, 3503 CrossRef CAS PubMed.
- M. Wieland and J. S. Hartig, Chem. Biol., 2007, 14, 757 CrossRef CAS PubMed.
- M. Wieland and J. S. Hartig, Nat. Protoc., 2009, 4, 1632 CrossRef CAS PubMed.
- S. Kumari, A. Bugaut and S. Balasubramanian, Biochemistry, 2008, 47, 12664 CrossRef CAS PubMed.
- T. Endoh, Y. Kawasaki and N. Sugimoto, Angew. Chem., Int. Ed., 2013, 52, 5522 CrossRef CAS PubMed.
- P. Murat, J. Zhong, L. Lekieffre, N. P. Cowieson, J. L. Clancy, T. Preiss, S. Balasubramanian, R. Khanna and J. Tellam, Nat. Chem. Biol., 2014, 10, 358 CrossRef CAS PubMed.
- T. Endoh, Y. Kawasaki and N. Sugimoto, Nucleic Acids Res., 2013, 41, 6222 CrossRef CAS PubMed.
- T. Endoh, Y. Kawasaki and N. Sugimoto, Methods, 2013, 64, 73 CrossRef CAS PubMed.
- T. Endoh and N. Sugimoto, Anal. Chem., 2013, 85, 11435 CrossRef CAS PubMed.
- C. H. Yu, M. P. Teulade-Fichou and R. C. Olsthoorn, Nucleic Acids Res., 2014, 42, 1887 CrossRef CAS PubMed.
- A. Arora and B. Suess, RNA Biol., 2011, 8, 802 CrossRef CAS PubMed.
- K. Ito, S. Go, M. Komiyama and Y. Xu, J. Am. Chem. Soc., 2011, 133, 19153 CrossRef CAS PubMed.
- D. Bhattacharyya, K. Nguyen and S. Basu, Biochemistry, 2014, 53, 5461 CrossRef CAS PubMed.
- F. C. Nielsen and J. Christiansen, Scand. J. Clin. Lab. Invest. Suppl., 1995, 220, 37 CAS.
- A. Decorsiere, A. Cayrel, S. Vagner and S. Millevoi, Genes Dev., 2011, 25, 220 CrossRef CAS PubMed.
- J. D. Beaudoin and J. P. Perreault, Nucleic Acids Res., 2013, 41, 5898 CrossRef CAS PubMed.
- P. H. Wanrooij, J. P. Uhler, T. Simonsson, M. Falkenberg and C. M. Gustafsson, Proc. Natl. Acad. Sci. U. S. A., 2010, 107, 16072 CrossRef CAS PubMed.
- M. Subramanian, F. Rage, R. Tabet, E. Flatter, J. L. Mandel and H. Moine, EMBO Rep., 2011, 12, 697 CrossRef CAS PubMed.
- R. Kostadinov, N. Malhotra, M. Viotti, R. Shine, L. D'Antonio and P. Bagga, Nucleic Acids Res., 2006, 34, D119 CrossRef CAS PubMed.
- H. Min, R. C. Chan and D. L. Black, Genes Dev., 1995, 9, 2659 CrossRef CAS.
- P. Sirand-Pugnet, P. Durosay, E. Brody and J. Marie, Nucleic Acids Res., 1995, 23, 3501 CrossRef CAS PubMed.
- D. Gomez, T. Lemarteleur, L. Lacroix, P. Mailliet, J. L. Mergny and J. F. Riou, Nucleic Acids Res., 2004, 32, 371 CrossRef CAS PubMed.
- M. C. Didiot, Z. Tian, C. Schaeffer, M. Subramanian, J. L. Mandel and H. Moine, Nucleic Acids Res., 2008, 36, 4902 CrossRef CAS PubMed.
- V. Marcel, P. L. Tran, C. Sagne, G. Martel-Planche, L. Vaslin, M. P. Teulade-Fichou, J. Hall, J. L. Mergny, P. Hainaut and E. Van Dyck, Carcinogenesis, 2011, 32, 271 CrossRef CAS PubMed.
- J. F. Fisette, D. R. Montagna, M. R. Mihailescu and M. S. Wolfe, J. Neurochem., 2012, 121, 763 CrossRef CAS PubMed.
- M. M. Ribeiro, G. S. Teixeira, L. Martins, M. R. Marques, A. P. de Souza and S. R. Line, Hum. Genet., 2014, 134, 37 CrossRef PubMed.
- M. Fry, Front. Biosci., 2007, 12, 4336 CrossRef CAS PubMed.
- C. Sissi, B. Gatto and M. Palumbo, Biochimie, 2011, 93, 1219 CrossRef CAS PubMed.
- V. Brown, P. Jin, S. Ceman, J. C. Darnell, W. T. O'Donnell, S. A. Tenenbaum, X. Jin, Y. Feng, K.
D. Wilkinson, J. D. Keene, R. B. Darnell and S. T. Warren, Cell, 2001, 107, 477 CrossRef CAS.
- J. C. Darnell, K. B. Jensen, P. Jin, V. Brown, S. T. Warren and R. B. Darnell, Cell, 2001, 107, 489 CrossRef CAS.
- M. Bensaid, M. Melko, E. G. Bechara, L. Davidovic, A. Berretta, M. V. Catania, J. Gecz, E. Lalli and B. Bardoni, Nucleic Acids Res., 2009, 37, 1269 CrossRef CAS PubMed.
- M. Melko and B. Bardoni, Biochimie, 2010, 92, 919 CrossRef CAS PubMed.
- E. Blackwell, X. Zhang and S. Ceman, Hum. Mol. Genet., 2010, 19, 1314 CrossRef CAS PubMed.
- R. Lu, H. Wang, Z. Liang, L. Ku, T. O'Donnell W, W. Li, S. T. Warren and Y. Feng, Proc. Natl. Acad. Sci. U. S. A., 2004, 101, 15201 CrossRef CAS PubMed.
- M. Castets, C. Schaeffer, E. Bechara, A. Schenck, E. W. Khandjian, S. Luche, H. Moine, T. Rabilloud, J. L. Mandel and B. Bardoni, Hum. Mol. Genet., 2005, 14, 835 CrossRef CAS PubMed.
- C. Schaeffer, B. Bardoni, J. L. Mandel, B. Ehresmann, C. Ehresmann and H. Moine, EMBO J., 2001, 20, 4803 CrossRef CAS PubMed.
- C. J. Westmark and J. S. Malter, PLoS Biol., 2007, 5, e52 Search PubMed.
- L. Menon and M. R. Mihailescu, Nucleic Acids Res., 2007, 35, 5379 CrossRef CAS PubMed.
- P. K. Todd, K. J. Mack and J. S. Malter, Proc. Natl. Acad. Sci. U. S. A., 2003, 100, 14374 CrossRef CAS PubMed.
- S. Khateb, P. Weisman-Shomer, I. Hershco-Shani, A. L. Ludwig and M. Fry, Nucleic Acids Res., 2007, 35, 5775 CrossRef CAS PubMed.
- T. L. Evans, A. C. Blice-Baum and M. R. Mihailescu, Mol. Biosyst., 2012, 8, 642 RSC.
- J. P. Vaughn, S. D. Creacy, E. D. Routh, C. Joyner-Butt, G. S. Jenkins, S. Pauli, Y. Nagamine and S. A. Akman, J. Biol. Chem., 2005, 280, 38117 CrossRef CAS PubMed.
- S. D. Creacy, E. D. Routh, F. Iwamoto, Y. Nagamine, S. A. Akman and J. P. Vaughn, J. Biol. Chem., 2008, 283, 34626 CrossRef CAS PubMed.
- S. Lattmann, M. B. Stadler, J. P. Vaughn, S. A. Akman and Y. Nagamine, Nucleic Acids Res., 2011, 39, 9390 CrossRef CAS PubMed.
- A. N. Sexton and K. Collins, Mol. Cell Biol., 2011, 31, 736 CrossRef CAS PubMed.
- E. P. Booy, M. Meier, N. Okun, S. K. Novakowski, S. Xiong, J. Stetefeld and S. A. McKenna, Nucleic Acids Res., 2012, 40, 4110 CrossRef CAS PubMed.
- S. Lattmann, B. Giri, J. P. Vaughn, S. A. Akman and Y. Nagamine, Nucleic Acids Res., 2010, 38, 6219 CrossRef CAS PubMed.
- M. Meier, T. R. Patel, E. P. Booy, O. Marushchak, N. Okun, S. Deo, R. Howard, K. McEleney, S. E. Harding, J. Stetefeld and S. A. McKenna, J. Biol. Chem., 2013, 288, 35014 CrossRef CAS PubMed.
- W. Huang, P. J. Smaldino, Q. Zhang, L. D. Miller, P. Cao, K. Stadelman, M. Wan, B. Giri, M. Lei, Y. Nagamine, J. P. Vaughn, S. A. Akman and G. Sui, Nucleic Acids Res., 2012, 40, 1033 CrossRef CAS PubMed.
- E. P. Booy, R. Howard, O. Marushchak, E. O. Ariyo, M. Meier, S. K. Novakowski, S. R. Deo, E. Dzananovic, J. Stetefeld and S. A. McKenna, Nucleic Acids Res., 2014, 42, 3346 CrossRef CAS PubMed.
- P. Chakraborty and F. Grosse, DNA Repair, 2011, 10, 654 CrossRef CAS PubMed.
- A. R. Haeusler, C. J. Donnelly, G. Periz, E. A. Simko, P. G. Shaw, M. S. Kim, N. J. Maragakis, J. C. Troncoso, A. Pandey, R. Sattler, J. D. Rothstein and J. Wang, Nature, 2014, 507, 195 CrossRef CAS PubMed.
- Z. Su, Y. Zhang, T. F. Gendron, P. O. Bauer, J. Chew, W. Y. Yang, E. Fostvedt, K. Jansen-West, V. V. Belzil, P. Desaro, A. Johnston, K. Overstreet, S. Y. Oh, P. K. Todd, J. D. Berry, M. E. Cudkowicz, B. F. Boeve, D. Dickson, M. K. Floeter, B. J. Traynor, C. Morelli, A. Ratti, V. Silani, R. Rademakers, R. H. Brown, J. D. Rothstein, K. B. Boylan, L. Petrucelli and M. D. Disney, Neuron, 2014, 83, 1043 CrossRef CAS PubMed.
- K. Takahama, A. Takada, S. Tada, M. Shimizu, K. Sayama, R. Kurokawa and T. Oyoshi, Chem. Biol., 2013, 20, 341 CrossRef CAS PubMed.
- J. D. Frantz and W. Gilbert, J. Biol. Chem., 1995, 270, 9413 CrossRef CAS PubMed.
- J. D. Frantz and W. Gilbert, J. Biol. Chem., 1995, 270, 20692 CrossRef CAS PubMed.
- A. Marnef, M. Maldonado, A. Bugaut, S. Balasubramanian, M. Kress, D. Weil and N. Standart, RNA, 2010, 16, 2094 CrossRef CAS PubMed.
- A. von Hacht, O. Seifert, M. Menger, T. Schutze, A. Arora, Z. Konthur, P. Neubauer, A. Wagner, C. Weise and J. Kurreck, Nucleic Acids Res., 2014, 42, 6630 CrossRef CAS PubMed.
- R. H. Shafer and I. Smirnov, Biopolymers, 2000, 56, 209 CrossRef CAS.
- A. R. Pinto, H. Li, C. Nicholls and J. P. Liu, Front. Biosci., 2011, 16, 187 CrossRef CAS PubMed.
- M. Salazar, B. D. Thompson, S. M. Kerwin and L. H. Hurley, Biochemistry, 1996, 35, 16110 CrossRef CAS PubMed.
- C. M. Azzalin, P. Reichenbach, L. Khoriauli, E. Giulotto and J. Lingner, Science, 2007, 318, 798 CrossRef CAS PubMed.
- B. Luke and J. Lingner, EMBO J., 2009, 28, 2503 CrossRef CAS PubMed.
- Y. Xu, Y. Suzuki, K. Ito and M. Komiyama, Proc. Natl. Acad. Sci. U. S. A., 2010, 107, 14579 CrossRef CAS PubMed.
- S. Schoeftner and M. A. Blasco, Nat. Cell Biol., 2008, 10, 228 CrossRef CAS PubMed.
- B. Luke, A. Panza, S. Redon, N. Iglesias, Z. Li and J. Lingner, Mol. Cell., 2008, 32, 465 CrossRef CAS PubMed.
- B. Pan, Y. Xiong, K. Shi, J. Deng and M. Sundaralingam, Structure, 2003, 11, 815 CrossRef CAS.
- B. Pan, Y. Xiong, K. Shi and M. Sundaralingam, Structure, 2003, 11, 825 CrossRef CAS.
- I. Lopez de Silanes, M. Stagno d'Alcontres and M. A. Blasco, Nat. Commun., 2010, 1, 33 Search PubMed.
- Z. Deng, J. Norseen, A. Wiedmer, H. Riethman and P. M. Lieberman, Mol. Cell, 2009, 35, 403 CrossRef CAS PubMed.
- G. Biffi, D. Tannahill and S. Balasubramanian, J. Am. Chem. Soc., 2012, 134, 11974 CrossRef CAS PubMed.
- J. L. Mergny, A. T. Phan and L. Lacroix, FEBS Lett., 1998, 435, 74 CrossRef CAS.
- J. L. Mergny, J. Li, L. Lacroix, S. Amrane and J. B. Chaires, Nucleic Acids Res., 2005, 33, e138 CrossRef PubMed.
- G. W. Collie and G. N. Parkinson, Chem. Soc. Rev., 2011, 40, 5867 RSC.
- S. T. Crooke, Annu. Rev. Med., 2004, 55, 61 CrossRef CAS PubMed.
- J. R. Thomas and P. J. Hergenrother, Chem. Rev., 2008, 108, 1171 CrossRef CAS PubMed.
- A. Bugaut, R. Rodriguez, S. Kumari, S. T. Hsu and S. Balasubramanian, Org. Biomol. Chem., 2010, 8, 2771 CAS.
- S. A. Ohnmacht and S. Neidle, Bioorg. Med. Chem. Lett., 2014, 24, 2602 CrossRef CAS PubMed.
- A. T. Phan, V. Kuryavyi and D. J. Patel, Curr. Opin. Struct. Biol., 2006, 16, 288 CrossRef CAS PubMed.
- Y. Xu, Chem. Soc. Rev., 2011, 40, 2719 RSC.
- A. Bugaut and S. Balasubramanian, Nucleic Acids Res., 2012, 40, 4727 CrossRef CAS PubMed.
- G. Collie, A. P. Reszka, S. M. Haider, V. Gabelica, G. N. Parkinson and S. Neidle, Chem. Commun., 2009, 7482 RSC.
- W. Tan and G. Yuan, Rapid Commun. Mass Spectrom., 2013, 27, 560 CrossRef CAS PubMed.
- L. P. Bai, M. Hagihara, K. Nakatani and Z. H. Jiang, Sci. Rep., 2014, 4, 6767 CrossRef PubMed.
- R. Rodriguez, K. M. Miller, J. V. Forment, C. R. Bradshaw, M. Nikan, S. Britton, T. Oelschlaegel, B. Xhemalce, S. Balasubramanian and S. P. Jackson, Nat. Chem. Biol., 2012, 8, 301 CrossRef CAS PubMed.
- M. Di Antonio, G. Biffi, A. Mariani, E. A. Raiber, R. Rodriguez and S. Balasubramanian, Angew. Chem., Int. Ed., 2012, 51, 11073 CrossRef CAS PubMed.
- G. Mirihana Arachchilage, M. J. Morris and S. Basu, Chem. Commun., 2014, 50, 1250 RSC.
- K. Halder, E. Largy, M. Benzler, M. P. Teulade-Fichou and J. S. Hartig, ChemBioChem, 2011, 12, 1663 CrossRef CAS PubMed.
- B. Zamiri, K. Reddy, R. B. Macgregor Jr. and C. E. Pearson, J. Biol. Chem., 2014, 289, 4653 CrossRef CAS PubMed.
- M. Faudale, S. Cogoi and L. E. Xodo, Chem. Commun., 2012, 48, 874 RSC.
- L. P. Bai, J. Liu, L. Han, H. M. Ho, R. Wang and Z. H. Jiang, Anal. Bioanal. Chem., 2014, 406, 5455 CrossRef CAS PubMed.
- S. Zhang, Y. Wu and W. Zhang, ChemMedChem, 2014, 9, 899 CrossRef CAS PubMed.
- M. Garavis, B. Lopez-Mendez, A. Somoza, J. Oyarzabal, C. Dalvit, A. Villasante, R. Campos-Olivas and C. Gonzalez, ACS Chem. Biol., 2014, 9, 1559 CrossRef CAS PubMed.
- E. Largy, N. Saettel, F. Hamon, S. Dubruille and M. P. Teulade-Fichou, Curr. Pharm. Des., 2012, 18, 1992 CrossRef CAS.
- B. Pagano, S. Cosconati, V. Gabelica, L. Petraccone, S. De Tito, L. Marinelli, V. La Pietra, F. S. di Leva, I. Lauri, R. Trotta, E. Novellino, C. Giancola and A. Randazzo, Curr. Pharm. Des., 2012, 18, 1880 CrossRef CAS.
- D. Renciuk, J. Zhou, L. Beaurepaire, A. Guedin, A. Bourdoncle and J. L. Mergny, Methods, 2012, 57, 122 CrossRef CAS PubMed.
- A. Benz, V. Singh, T. U. Mayer and J. S. Hartig, ChemBioChem, 2011, 12, 1422 CrossRef CAS PubMed.
- K. M. Rahman, K. Tizkova, A. P. Reszka, S. Neidle and D. E. Thurston, Bioorg. Med. Chem. Lett., 2012, 22, 3006 CrossRef CAS PubMed.
- L. Lacroix, A. Seosse and J. L. Mergny, Nucleic Acids Res., 2011, 39, e21 CrossRef PubMed.
- R. Haudecoeur, L. Stefan, F. Denat and D. Monchaud, J. Am. Chem. Soc, 2013, 135, 550 CrossRef CAS PubMed.
- A. Laguerre, L. Stefan, M. Larrouy, D. Genest, J. Novotna, M. Pirrotta and D. Monchaud, J. Am. Chem. Soc., 2014, 136, 12406 CrossRef CAS PubMed.
- C. Schaffitzel, I. Berger, J. Postberg, J. Hanes, H. J. Lipps and A. Pluckthun, Proc. Natl. Acad. Sci. U. S. A., 2001, 98, 8572 CrossRef CAS PubMed.
- G. Biffi, D. Tannahill, J. McCafferty and S. Balasubramanian, Nat. Chem., 2013, 5, 182 CrossRef CAS PubMed.
- M. Isalan, S. D. Patel, S. Balasubramanian and Y. Choo, Biochemistry, 2001, 40, 830 CrossRef CAS PubMed.
- S. D. Patel, M. Isalan, G. Gavory, S. Ladame, Y. Choo and S. Balasubramanian, Biochemistry, 2004, 43, 13452 CrossRef CAS PubMed.
- J. Jana, R. K. Kar, A. Ghosh, A. Biswas, S. Ghosh, A. Bhunia and S. Chatterjee, Mol. Biosyst., 2013, 9, 1833 RSC.
- K. Takahama and T. Oyoshi, J. Am. Chem. Soc., 2013, 135, 18016 CrossRef CAS PubMed.
- Y. Xu and M. Komiyama, Methods, 2012, 57, 100 CrossRef CAS PubMed.
- R. C. Olsthoorn, Nucleic Acids Res., 2014, 42, 9327 CrossRef PubMed.
- S. Weiss, D. Proske, M. Neumann, M. H. Groschup, H. A. Kretzschmar, M. Famulok and E. L. Winnacker, J. Virol., 1997, 71, 8790 CAS.
- S. Sekiya, F. Nishikawa, K. Noda, P. K. Kumar, T. Yokoyama and S. Nishikawa, Nucleic Acids Symp. Ser., 2005, 361 CrossRef CAS PubMed.
- T. Mori, A. Oguro, T. Ohtsu and Y. Nakamura, Nucleic Acids Res., 2004, 32, 6120 CrossRef CAS PubMed.
- M. Homann, M. Lorger, M. Engstler, M. Zacharias and H. U. Goringer, Comb. Chem. High Throughput Screening, 2006, 9, 491 CrossRef CAS.
- M. Metifiot, O. Leon, L. Tarrago-Litvak, S. Litvak and M. L. Andreola, Biochimie, 2005, 87, 911 CrossRef CAS PubMed.
- C. T. Lauhon and J. W. Szostak, J. Am. Chem. Soc., 1995, 117, 1246 CrossRef CAS.
- D. Levesque, J. D. Beaudoin, S. Roy and J. P. Perreault, Biochem. J., 2007, 403, 129 CrossRef CAS PubMed.
- J. Tan, C. Vonrhein, O. S. Smart, G. Bricogne, M. Bollati, Y. Kusov, G. Hansen, J. R. Mesters, C. L. Schmidt and R. Hilgenfeld, PLoS Pathog., 2009, 5, e1000428 Search PubMed.
- H. Huang, N. B. Suslov, N. S. Li, S. A. Shelke, M. E. Evans, Y. Koldobskaya, P. A. Rice and J. A. Piccirilli, Nat. Chem. Biol., 2014, 10, 686 CrossRef CAS PubMed.
- K. D. Warner, M. C. Chen, W. Song, R. L. Strack, A. Thorn, S. R. Jaffrey and A. R. Ferre-D'Amare, Nat. Struct. Mol. Biol., 2014, 21, 658 CAS.
- T. Li, E. Wang and S. Dong, J. Am. Chem. Soc., 2009, 131, 15082 CrossRef CAS PubMed.
- C. Zhao, Y. Song, J. Ren and X. Qu, Biomaterials, 2009, 30, 1739 CrossRef CAS PubMed.
- D. Miyoshi, Z. M. Wang, H. Karimata and N. Sugimoto, Nucleic Acids Symp. Ser., 2005, 43 CrossRef CAS PubMed.
- Y. Krishnan and F. C. Simmel, Angew .Chem., Int. Ed., 2011, 50, 3124 CrossRef CAS PubMed.
- P. Agarwala, S. Pandey and S. Maiti, ChemBioChem, 2013, 14, 2077 CrossRef CAS PubMed.
|
This journal is © The Royal Society of Chemistry 2015 |