DOI:
10.1039/C4MT00206G
(Paper)
Metallomics, 2015,
7, 102-111
The biochemical effects of extracellular Zn2+ and other metal ions are severely affected by their speciation in cell culture media
Received
3rd August 2014
, Accepted 15th October 2014
First published on 15th October 2014
Abstract
Investigations of physiological and toxicological effects of metal ions are frequently based on in vitro cell culture systems, in which cells are incubated with these ions in specialized culture media, instead of their physiological environment. This allows for targeted examination on the cellular or even molecular level. However, it disregards one important aspect, the different metal ion speciation under these conditions. This study explores the role of culture conditions in investigations with zinc ions (Zn2+). Their concentration is buffered by several orders of magnitude by fetal calf serum. Due to the complexity of serum and its many zinc-binding components, zinc speciation in culture media cannot be completely predicted. Still, the primary effect is due to the main Zn2+-binding protein albumin. Buffering reduces the free Zn2+ concentration, thereby diminishing its biological effects, such as cytotoxicity and the impact on protein phosphorylation. This is not limited to Zn2+, but is also observed with Ag+, Cu2+, Pb2+, Cd2+, Hg2+, and Ni2+. Usually, the serum content of culture media, and thereby their metal buffering capacity, is only a fraction of that in the physiological cellular environment. This leads to systematic over-estimation of the effects of extracellular metal ions when standard cell culture conditions are used as model systems for assessing potential in vivo effects.
Introduction
On average, the total Zn2+ concentration in mammalian cells is several hundred micromolar, most of it tightly bound to proteins.1 Frequently, “free” Zn2+ is used as an operational term to distinguish an exchangeable pool bound by smaller ligands, such as amino acids, low molecular weight anions, and water, from protein bound Zn2+. Within cells, free Zn2+ is thought to serve functions in signal transduction, for example, negatively regulating the activity of protein tyrosine phosphatases (PTP).2 Hence, considerable efforts have been made to measure the free intracellular concentrations of Zn2+, which usually lie in the picomolar concentration range.3 The cellular proteome has a significant buffering capacity for ions such as Zn2+.4 This is also well documented for the extracellular environment. Metal ions can bind to various extracellular proteins, leading to free ion concentrations that are orders of magnitude below the total concentration, in particular, in the presence of serum or plasma. These contain high concentrations of albumin and several other proteins, which bind transition metal ions.5,6 For example, next to albumin, which is the major zinc-binding protein in plasma, 12 additional proteins also bind Zn2+.6
Cell culture-based investigations have been successfully applied for investigating many cellular and molecular mechanisms in heavy metal ion toxicity, including the role of Toll-like receptor 4 in nickel allergy, the immunotoxicity of mercury, and the involvement of Zn2+-toxicity in various pathologies of the central nervous system.7–9 Still, extracellular metal ion buffering is generally being ignored. In most cases, defined ion concentrations are added to cell cultures, without particular concern regarding the metal ion speciation in the extracellular environment. Nevertheless, it has been shown that protein composition of the culture medium affects the dose–response of Zn2+-induced cytokine secretion in monocytes.10 In one of the few studies taking into account the speciation of extracellular Zn2+, it was shown that cells die in a narrow concentration range of free Zn2+, which is normally nanomolar.11 It has been suggested that the free Zn2+ concentration is potentially a far more meaningful parameter for describing its biological activity than the total concentration, and that, analogous to the pH, pZn (the negative decadic logarithm) could be an appropriate measure to describe it.3,11
This study investigates the impact of metal ion buffering by culture medium on the toxic effects of metal ions, mainly using Zn2+ as a model ion. In cell culture media serum levels in general, and albumin in particular, have substantial impact on the toxicity of Zn2+. Under normal culture conditions, the free concentration of this ion is buffered by several orders of magnitude, significantly reducing its toxicity and ability to induce p38 phosphorylation. Accordingly, similar effects on toxicity were observed for several other toxicologically relevant metal ions, namely, Cu2+, Pb2+, Cd2+, Hg2+, Ni2+, and Ag+.
Results
Relationship between cytotoxicity and free Zn2+
Culture of Jurkat cells in the presence of different Zn2+ concentrations leads to a sharp decline in cellular vitality at concentrations exceeding 100 μM of added Zn2+, with nearly complete toxicity at 250 μM (Fig. 1A). Despite the addition of up to 250 μM Zn2+, free Zn2+ in the culture medium remains below 30 nM, being buffered by nearly four orders of magnitude (Fig. 1B). The loss of cellular vitality coincides with a steep increase of free medium Zn2+, indicating the end of a limited buffering capacity of the culture medium, with subsequent uptake into the cells. Accordingly, free intracellular Zn2+ is significantly elevated under these conditions (Fig. 1C), even though the free intracellular concentration remains below 4 nM. Notably, Zn2+ buffering by culture medium (in the absence of cells) seems to have a component with slow kinetics. There still is a considerable decline of free Zn2+ between 4 h and 8 h, with no further major reduction between 8 h and 24 h (Fig. 1D).
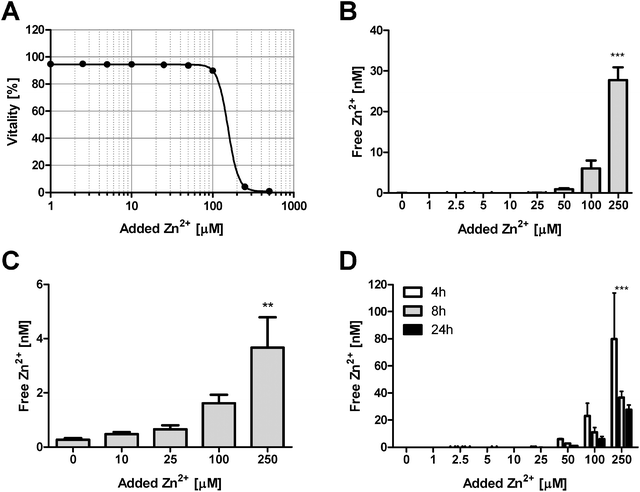 |
| Fig. 1 Toxicity and free Zn2+ under normal culture conditions. RPMI 1640 cell culture medium containing 10% FCS was supplemented with the indicated concentrations of ZnSO4. (A) After incubation for 24 h, the vitality of Jurkat cells was measured by staining with propidium iodide and flow cytometry. A sigmoidal dose–response curve was fitted by non-linear regression. (B) Free Zn2+ concentration in cell-free culture medium after 24 h, measured with FluoZin-3. (C) Free intracellular Zn2+ concentration, measured in Jurkat cells incubated with the indicated concentrations of Zn2+ for 2 h in normal culture medium and loaded with FluoZin-3. (D) Free Zn2+ concentration in media incubated for 4 h, 8 h, and 24 h. All data are shown as means of n = 3 independent experiments ± SEM. Means significantly different from the untreated controls are indicated (** = p < 0.01; *** = p < 0.001; ANOVA with Bonferroni post hoc test). | |
Fetal calf serum (FCS) as a Zn2+-buffer
Zn2+-buffering in the culture medium is likely mediated by Zn2+-binding serum proteins. Consequently, the lethal concentration (LC50) is reduced from 154.5 μM at 10% FCS (Fig. 1A) to 82.8 μM in the presence of 1% FCS (Fig. 2A). The effect of buffering is not limited to cytotoxicity. Zn2+ inhibits PTPs, thereby activating phosphorylation of kinases such as the mitogen-activated protein kinase (MAPK) p38. Western blot analysis shows increased p38 phosphorylation after addition of 100 and 300 μM Zn2+ in medium containing 1% FCS, whereas in the presence of 10% FCS, there is only weak phosphorylation at 300 μM (Fig. 2B and C). According to a two-way ANOVA of the densitometric quantification, the concentrations of Zn2+ and FCS are both extremely significant factors affecting the phosphorylation of p38 (p < 0.0001).
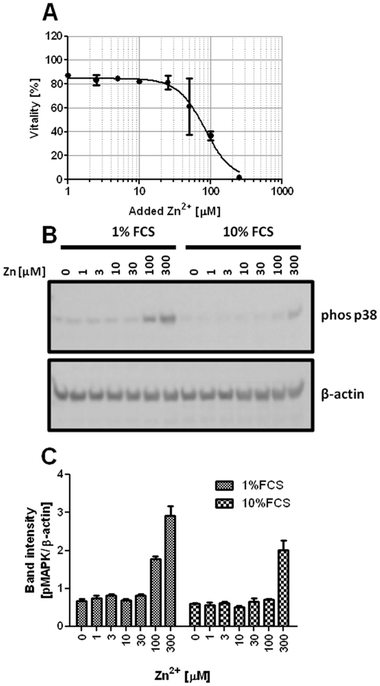 |
| Fig. 2 Impact of FCS on Zn2+-mediated p38 activation. Jurkat cells were incubated with the indicated concentrations of ZnSO4. (A) Vitality of cells after 24 h incubation in RPMI 1640 cell culture medium containing 1% FCS. A sigmoidal dose–response curve was fitted by non-linear regression. (B and C) Western blot of cells incubated for 30 min either in RPMI 1640 cell culture medium containing 1% or 10% FCS. Data are shown as one representative blot (B) or densitometric quantification (C) of n = 3 independent experiments. | |
Reduced toxicity of Zn2+ in the presence of higher FCS concentrations is not limited to Jurkat cells. Three other cell lines, Raw 264.7 (Fig. 3A), BV-2 (Fig. 3B), and L929 (Fig. 3C), showed an equivalent effect when Zn2+ toxicity was compared in the presence of 1% and 10% FCS.
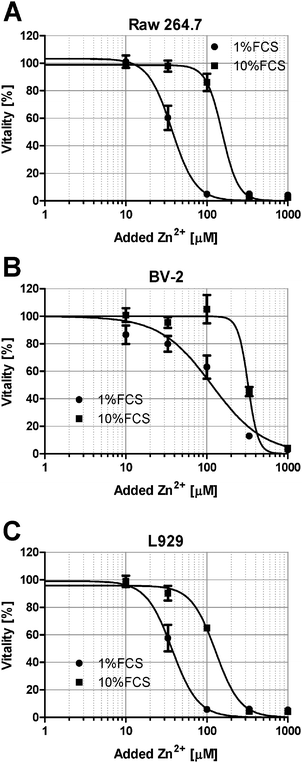 |
| Fig. 3 Impact of serum on Zn2+ toxicity in different cell lines. Raw 264.7 (A), BV-2 (B), and L929 (C) cells were cultivated for 24 h in their respective culture media, supplemented with either 1% or 10% FCS, in the presence of the indicated concentrations of Zn2+. Cellular vitality was determined using the MTT assay and sigmoidal dose–response curves were fitted by non-linear regression. All data are shown as means ± SEM of n = 3 independent experiments. | |
To investigate the potential impact of FCS on Zn2+ uptake, free intracellular Zn2+ was measured in Raw 264.7 cells loaded with FluoZin-3. In the absence of serum, the addition of 1 μM Zn2+ causes a transient spike to approximately 75 nM free intracellular Zn2+ (Fig. 4A), whereas 1% FCS reduces the effect to 1 nM (Fig. 4B), and it is almost completely abrogated by 10% FCS (Fig. 4C).
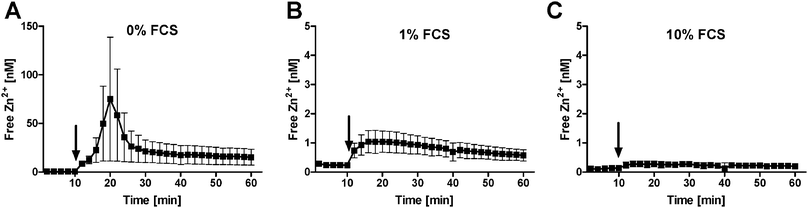 |
| Fig. 4 Impact of serum on free Zn2+ uptake. Raw 264.7 cells were loaded with FluoZin-3 and free intracellular Zn2+ was measured in the presence of 0% (A), 1% (B) or 10% (C) FCS. After 10 minutes recording of the baseline, 1 μM ZnSO4 was added (arrow). Data are shown as means of n = 3 independent experiments ± SEM. | |
Zn2+ buffering by albumin
Serum albumin is the major Zn2+-binding protein in serum.5 Addition of a physiological concentration of BSA (50 mg ml−1, corresponding to approximately 750 μM) to serum-free culture medium abrogates Zn2+ toxicity in Raw 264.7 cells (Fig. 5A). In contrast, addition of Na2HPO4 (10 mM, triplicating the medium's concentration of phosphate), which can form Zn2+-complexes with low solubility,12 has no effect (Fig. 5A). Cell-free measurements confirm a concentration-dependent buffering of free Zn2+ by purified BSA (Fig. 5B). FCS contains growth factors, serving as signals for cellular survival and division.13 The absence of these factors could render the cells more susceptible to Zn2+ toxicity, independent of buffering of the metal ion. However, in the presence of 10% FCS, addition of BSA still reduces Zn2+ toxicity (Fig. 5C), indicating that this effect is also observed when the concentration of growth factors remains unchanged.
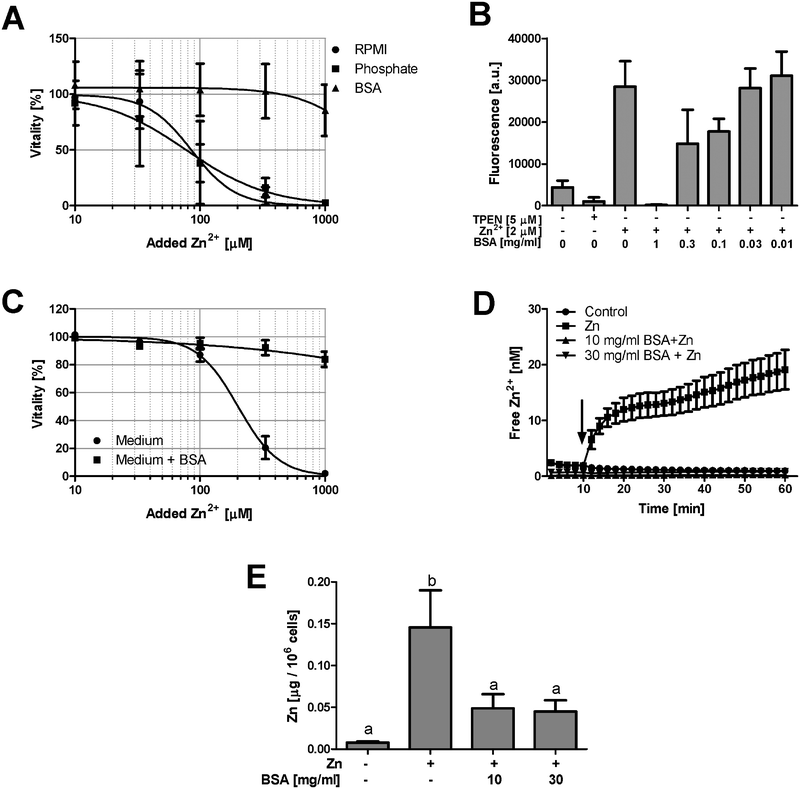 |
| Fig. 5 Zn2+ buffering by BSA and phosphate. (A) Raw 264.7 cells were cultured for 24 h in serum-free RPMI 1640 supplemented with Na2HPO4 (10 mM) or BSA (50 mg ml−1), followed by measurement of vitality with the MTT assay. Sigmoidal dose–response curves were fitted by non-linear regression. (B) Cell-free measurement of Zn2+ with FluoZin-3 in the presence of the indicated concentrations of BSA. (C) Raw 264.7 cells were cultured for 24 h in normal culture medium (containing 10% FCS) and in medium additionally supplemented with BSA (50 mg ml−1). Cellular vitality was determined using the MTT assay and sigmoidal dose–response curves were fitted by non-linear regression. All data are shown as means with SEM from at least n = 3 independent experiments. (D) PBMC were isolated from healthy human donors, loaded with FluoZin-3 and, after 10 minutes recording of the baseline, exposed to 15 μM Zn2+ (arrow) in the presence of the indicated concentrations of BSA. Data show one representative of n = 5 donors (measured in triplicate, ±SEM). (E) Total zinc was measured by AAS after 30 min incubation of PBMC with 15 μM Zn2+ in the presence of the indicated concentrations of BSA. Data are shown as means ± SEM from n = 8 donors. Significantly different means do not share the same letter (one-way ANOVA with Bonferroni post hoc test). | |
PBMC are directly exposed to plasma proteins and Zn2+ in the blood. In a buffer system with minimal Zn2+-binding, the nominally physiological concentration of 15 μM Zn2+ leads to massive uptake of this ion, which is abrogated by 10 mg ml−1 (corresponding to approximately 150 μM) BSA (Fig. 5D). This is not due to interference of BSA with the detection of Zn2+ by the fluorescent probe, because exposure of PBMC to Zn2+ elevated the total cellular Zn2+ content, measured by atomic absorption spectrometry (AAS), an effect which is also significantly reduced by the presence of BSA (Fig. 5E).
Zn2+ buffering in the presence of pyrithione
The next experiments are performed to elucidate if the effect of FCS is also observed when pyrithione is present, which is frequently used as an ionophore, facilitating Zn2+ uptake into cells. Pyrithione shifts toxicity to lower Zn2+ concentrations, but FCS still preserves vitality (Fig. 6A). Additionally, the presence of pyrithione reduces the Zn2+ concentration required to induce p38 phosphorylation in Jurkat cells by one order of magnitude (Fig. 2B, C and 6B, C). Nevertheless, the impact of Zn2+ is antagonized by FCS; a two-way ANOVA of the densitometric quantification confirms that the concentrations of Zn2+ (p = 0.0002) and FCS (p = 0.0026) both significantly affect p38 phosphorylation in the presence of pyrithione.
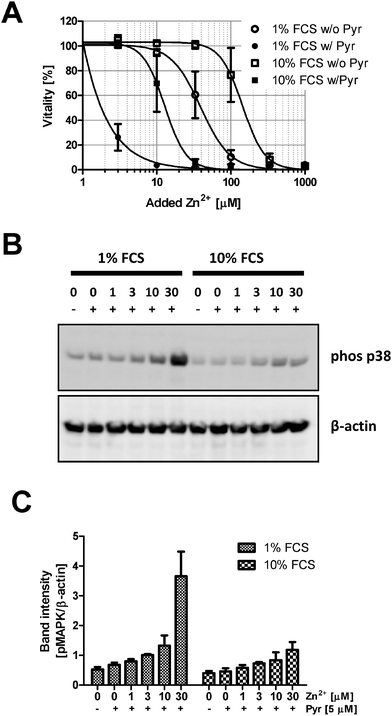 |
| Fig. 6 Effect of serum on Zn2+ uptake mediated by pyrithione. (A) Raw 264.7 cells were kept in RPMI 1640 cell culture medium containing either 1% (circles) or 10% (squares) FCS. Cellular vitality was determined by the MTT assay after culture for 24 h in the absence (empty symbols) or presence (filled symbols) of sodium pyrithione (Pyr, 5 μM) and varying concentrations of ZnSO4 as indicated. Data are shown as means from n = 3 independent experiments ± SEM and sigmoidal dose–response curves were fitted by non-linear regression. (B and C) Phosphorylation of p38 MAPK was analyzed by Western blots with Jurkat cells incubated as indicated for 30 min in RPMI 1640 cell culture medium containing 1% or 10% FCS. Data are shown as one representative blot (B) or densitometric quantification (C) of n = 3 independent experiments. | |
Impact of FCS on the cytotoxicity of other metal ions
Investigation of metal ions in cell culture is clearly not limited to Zn2+, but also deals with many other heavy metal ions, and BSA is known to bind several additional metal ions that have physiological or toxicological relevance.5 In addition to ZnSO4, the toxicity of CuSO4, Pb(NO3)2, CdSO4, HgCl2, NiSO4, and AgNO3 is examined in Raw 264.7 cells. For all metal ions investigated, a similar buffering by FCS is observed, and while there is a considerable variation between the different ions due to distinct degrees of their cytotoxicity, the respective LC50 values for all ions consistently increase from 1% over 5% to 10% FCS (Fig. 7).
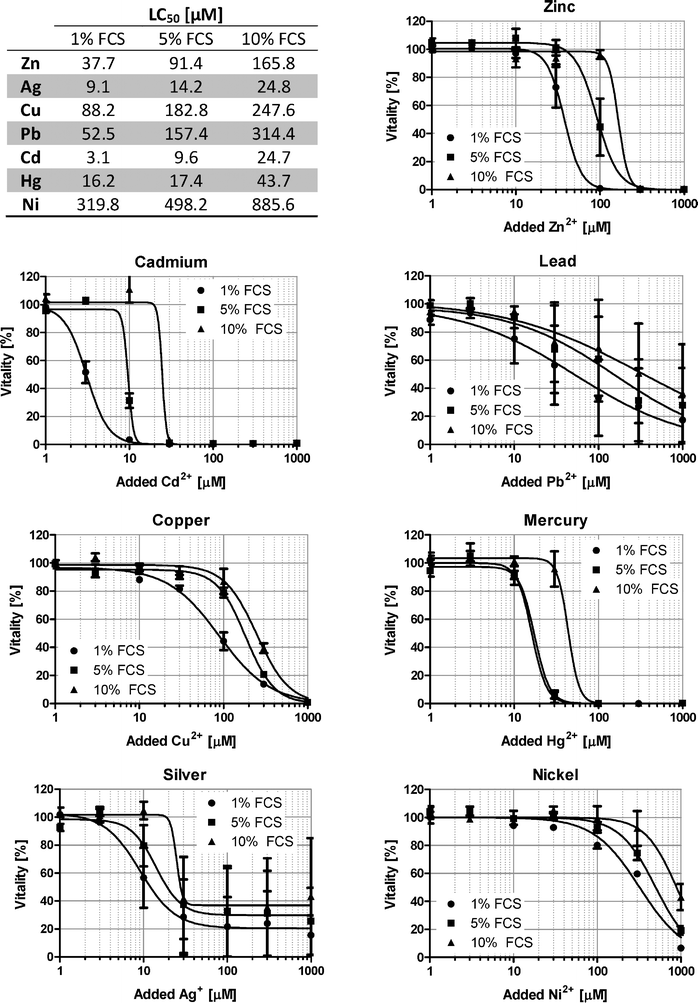 |
| Fig. 7 Effect of serum on the toxicity of seven different metal ions. Raw 264.7 cells were grown in RPMI 1640 cell culture medium containing 1% (circles), 5% (squares) or 10% (triangles) FCS. After 24 h in the presence of ZnSO4, CuSO4, Pb(NO3)2, CdSO4, HgCl2, NiSO4 or AgNO3, the remaining vitality was determined by the MTT assay. All data are shown as means ± SEM from n = 3 independent experiments. Sigmoidal dose–response curves were fitted by non-linear regression and the resulting LC50 values are indicated in the table. | |
Zn2+ toxicity at physiological serum concentrations
To investigate Zn2+ toxicity in an experimental setting closer to the in vivo situation, the toxicity in culture medium is compared to cells growing in 100% FCS (Fig. 8A). The presence of a physiological serum concentration significantly attenuates the toxic effects of Zn2+. This is confirmed by experiments with PBMC. Culture in serum obtained from the same donor leads to reduced Zn2+ toxicity, compared to cell culture medium (Fig. 8B).
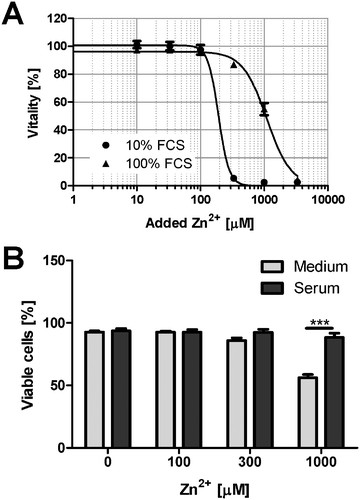 |
| Fig. 8 Zn2+ toxicity in the presence of 100% serum. (A) Raw 264.7 cells were grown in RPMI 1640 cell culture medium containing 10% FCS (circles), or in 100% FCS (triangles). After 24 h in the presence of the indicated concentrations of ZnSO4, the remaining vitality was determined by the MTT assay. All data are shown as means ± SEM from n = 3 independent experiments. Sigmoidal dose–response curves were fitted by non-linear regression. (B) PBMC were cultured for 24 h in the presence of the indicated concentrations of ZnSO4, either in RPMI 1640 cell culture medium containing 10% FCS or in 100% donor's own serum. The percentage of viable cells was determined by flow cytometry after staining with propidium iodide. Data are shown as means ± SEM from n = 3 donors (*** = p < 0.001; ANOVA with Bonferroni post hoc test). | |
Discussion
The reference range for the total concentration of Zn2+ in human plasma is between 76 and 125 μg dl−1 (roughly corresponding to 12–20 μM).14 In comparison, the free concentration is several orders of magnitude lower.15,16 This is mainly due to binding by albumin, which is present in a 30-fold molar excess to Zn2+,17 with a reference range in healthy controls between 35 and 51 mg ml−1 (corresponding to approximately 500–750 μM).18 Albumin has four known metal ion binding sites with different preferences and affinities. Physiologically, Zn2+-binding is mediated by two of these sites, but for one of them (site B), its affinity for Zn2+ and its exact localization in the protein remain unknown.5 Most reports for the site A, also known as the multi-metal binding site, which is considered the major Zn2+ binding site, give an affinity of approximately 100 nM.5 An exact calculation of the resulting free Zn2+ concentration is further complicated by competition between different metal ions present in biological fluids for the binding sites of albumin. Moreover, Zn2+ binding is also regulated by additional factors, such as fatty acids.19 In light of the excess of albumin, the vast majority of Zn2+ will be in a protein-bound state even if only site A is considered, resulting in low nanomolar free Zn2+ concentrations in biological fluids such as plasma and serum-supplemented cell culture media. We found even lower, sub-nanomolar free Zn2+ in our culture media, suggesting a contribution of other binding sites in albumin, but also of low molecular weight ligands and of other Zn2+-binding proteins, such as α2-macroglobulin and transferrin, contributing to the Zn2+ buffering capacity.6,20 In a comparable manner, cell lysates buffer Zn2+,4 and addition of micromolar concentrations of Zn2+ to cell lysates is required to obtain nanomolar concentrations of free Zn2+, for example, for inhibiting PTPs in vitro.21
In the present study extracellular Zn2+ was shown to be buffered from micromolar (added) to nanomolar or even picomolar (free) concentrations under normal cell culture conditions. The culture media used in our experiments contain a basal amount of 3 μM Zn2+, originating virtually entirely from the 10% FCS supplement (data not shown). The addition of 250 μM Zn2+ killed over 95% of Jurkat cells, while free Zn2+ concentrations remained below 30 nM. The effect of buffering seems to be more important than the cell type, as similar results were observed in other cell lines. Once the buffering capacity of the culture medium is exceeded, free Zn2+ increases to toxic levels, which are still nanomolar. In a similar manner, a different study has previously identified 100 nM as the toxic threshold for free extracellular Zn2+.11
Albumin has been reported to facilitate the uptake of Zn2+ into endothelial cells.22 If the ion would remain bound after its uptake, it would be invisible to detection by fluorescent probes and might also not be contributing to toxicity. Hence, Zn2+ may still be taken up in the presence of serum proteins, just not in its free form. This has been investigated by AAS. As shown in Fig. 5E, the uptake of Zn2+ (15 μM added to extracellular buffer) is significantly lower in the presence of BSA when it is present in tenfold (10 mg ml−1, corresponding to 150 μM) or thirtyfold (30 mg ml−1, corresponding to 450 μM) molar excess. However, there was no concentration-dependent effect of the addition of BSA. This might reflect that a maximum of albumin-bound Zn2+ is already taken up in the presence of 10 mg ml−1 BSA, with no further effect at 30 mg ml−1. Potentially only the uptake of additional, free Zn2+ is buffered by the protein. Whereas albumin certainly contributes significantly to buffering, there are also other relevant factors. Zinc phosphate is generally considered to be of very limited solubility.12 However, calculating zinc speciation in the presence of phosphate using CHEAQS software23 (for the concentrations used in our experiments, at neutral pH, and in the absence of other metals and ligands) shows that the predominant zinc species (>99%) is the soluble ZnH(PO4)23− complex. Accordingly, the presence of millimolar concentrations of additional phosphate had no effect on Zn2+ toxicity, indicating that precipitation of zinc phosphate is negligible under these conditions.
The data in Fig. 1D indicate that some complexes that reduce free Zn2+ levels seem to be forming over time, especially in the first eight hours. However, two aspects should be kept in mind. First, these observations were made after addition of supraphysiological Zn2+ concentrations, which might be buffered by ligands that are normally not binding Zn2+. Second, plasma zinc has a high turnover rate, being exchanged approximately 150 times per day.24 Hence, events with slow kinetics will have to be taken into account only in cell culture, whereas they should not be of physiological relevance in vivo.
High micro- or even millimolar concentrations of Zn2+ are frequently applied to cell cultures to elicit a biological response, even though it is widely accepted that such concentrations are not physiologically relevant. Importantly, even the addition of so-called physiological concentrations of Zn2+, for example 10–15 μM, can also not be considered physiological in the absence of buffering proteins. Physiological concentrations of free Zn2+ are in the pico- to low nanomolar range.25 In buffers or serum-free media supplemented with micromolar amounts of Zn2+, the relevant free concentration acting on the cells in the absence of any buffering proteins will exceed the physiological concentrations by several orders of magnitude, even though the nominal total concentration may be identical. Investigations with cell lines or isolated primary cells are usually performed in culture media, typically containing between 5 and 20% serum, with most experiments in cell culture being performed in the presence of 10% FCS. This constitutes only one tenth of the physiological buffering capacity in vivo. The latter leads to a considerable reduction of the effective concentration of metal ions, and a significant over-estimation of the cellular effects that these toxic metal ions may have when toxic effects are deduced from cell culture experiments. For example, 1 mM Zn2+ killed virtually all Raw 264.7 cells under normal cell culture conditions (medium with 10% FCS), whereas viability remained well above 50% when the same amount of Zn2+ was added in the presence of 100% FCS. Of note, physiological concentrations of albumin in human plasma are between 35 and 51 mg ml−1.18 Consequently, the data in Fig. 8 confirm that culture in 100% serum, which is much closer to the physiological conditions that cells encounter in vivo, results in strongly elevated tolerance to Zn2+ toxicity.
The effects described above are not only relevant for Zn2+, but also for several other metal ions. Albumin has been characterized with regard to binding of Cu2+ and Zn2+ as a physiological transport mechanism, but has also been shown to bind several toxicologically relevant metal ions such as Ni2+, Co2+, or Cd2+.5 For Pb2+ it has been shown that the presence of FCS affects its solubility, most likely by binding to albumin and other serum proteins,26 and that FCS reduces the uptake of Pb2+ into cultured astroglia.27 As demonstrated in Fig. 7, buffering by serum is clearly an issue for several metal ions in addition to Zn2+. In conclusion, the complexity of serum and its many zinc-binding components makes it impossible to predict the exact zinc speciation in culture media. Especially the interaction of albumin and Zn2+ is still subject to investigation. Still, it is clear that free metal ion concentrations are far more relevant for toxicological considerations than total amounts of these metals. The extent to which the metal ions are buffered should be known and taken into consideration when designing an experiment. Notably, FCS is a natural product. Its composition varies between different suppliers, and even batches. Hence, some degree of variation must be expected for metal binding, and the values derived from this study can only serve as an indicator for the extent of Zn2+-binding by FCS. Ideally, free Zn2+ is determined under the particular conditions used by each lab individually. As recently postulated, metal ion buffers are appropriate and recommendable tools for applying controlled extracellular concentrations of free metal ions.11 Otherwise, experiments in cell culture might lead to a systematic over-estimation of the potential effects of extracellular metal ions in vivo.
Experimental
Materials
FluoZin-3 acetoxymethyl ester and FluoZin-3 tetrapotassium salt were both obtained from Invitrogen (Karlsruhe, Germany). ZnSO4 × 7H2O was obtained from Merck (Darmstadt, Germany). Bovine Serum Albumin (BSA) was procured from PAA (Cölbe, Germany). N,N,N′,N′-Tetrakis(2-pyridylmethyl)ethylenediamine (TPEN) and Na-pyrithione were purchased from Sigma-Aldrich (Germany). HNO3, H2O2 and H2O for atomic absorption were of appropriate quality for trace element analysis (TraceSelect, Fluka, Germany). All other reagents were of analytical purity and obtained from standard sources.
Cell culture
All cells were cultured at 37 °C and saturated humidity in a mixture of 95% air and 5% CO2. The human acute T-cell leukemia cell line Jurkat was grown in RPMI 1640 supplemented with 2 mM L-glutamine, 100 U ml−1 penicillin, 100 μg ml−1 streptomycin, 1 mM Na-pyruvate, and the non-essential amino acid supplement (all from Lonza, Verviers, Belgium). Raw 264.7 murine macrophages and L929 murine fibroblasts were cultured in Roswell Park Memorial Institute (RPMI) 1640 medium supplemented with 2 mM L-glutamine, 100 U ml−1 penicillin, and 100 μg ml−1 streptomycin, and the murine microglial cell line BV-2 in Dulbecco's Modified Eagle's Medium (DMEM) supplemented with 2 mM L-glutamine, 100 U ml−1 penicillin, and 100 μg ml−1 streptomycin. Peripheral blood mononuclear cells (PBMC) were isolated from heparinized peripheral venous blood from healthy consenting donors by density gradient centrifugation using Ficoll as described,28 and cultured in the same medium as Raw 264.7 cells. For standard cell culture, all media were supplemented with 10% low endotoxin fetal calf serum (FCS, obtained from PAA Germany) that had been heat-inactivated at 56 °C for 30 min prior to use. Alternative serum concentrations used for the experiments are indicated in the respective figure legends.
Cytotoxicity tests
All cells were seeded onto 96-well plates and incubated for 24 h under the conditions described in the respective figure legends. Subsequently, cells in the suspension culture (Jurkat, PBMC) were collected by centrifugation, taken up in Phosphate Buffered Saline (PBS), incubated with propidium iodide (1 μg ml−1) for 10 min at room temperature and dye uptake (indicating membrane damage as a result of cell death) was analyzed by flow cytometry on a Becton Dickinson FACSCalibur (BD Biosciences, Heidelberg, Germany). A control with Na2SO4 confirmed that the toxicity was not caused by the anion. Adherent cells (Raw 264.7, BV-2, L929) were incubated for three hours with 0.01% (w/v) methylthiazolyldiphenyl-tetrazolium bromide (MTT) in normal culture medium. Cells were then lysed in isopropanol and the absorption determined at 570 nm, using a reference wavelength of 700 nm, on a Tecan Sunrise well plate reader (Crailsheim, Germany). Data were analyzed using GraphPad Prism software version 5.01, applying a non-linear regression, fitting a sigmoidal dose–response curve with variable slope as a function of the logarithm of concentration.
Free Zn2+ measurements in cell culture medium
Complete culture medium was preincubated (w/o cells) for 24 h at 37 °C, 5% CO2, at saturated humidity in 96-well plates (100 μl per well). Zinc sulfate was added and the medium was incubated under the same conditions for additional 24 h. FluoZin-3 (tetrapotassium salt) was added to a final concentration of 1 μM. Samples were equilibrated for 30 min, wells were sealed with adhesive sealing foil (Nunc, Roskilde, Denmark) to preserve the atmosphere during the measurements, ensuring conditions identical to the ones during cell culture. The resulting fluorescence was recorded on a Tecan Ultra 384 fluorescence well plate reader (Tecan, Crailsheim, Germany) at 37 °C, using excitation and emission wavelengths of 485 nm and 535 nm, respectively. Free zinc concentrations were calculated using the formula of Grynkiewicz et al.29 For the determination of Fmin and Fmax, the medium was incubated with TPEN (10 μM) or ZnSO4 (10 mM), respectively.
Intracellular free Zn2+ measurements
Free intracellular Zn2+ was measured as previously described.30 Briefly, cells were loaded with 1 μM FluoZin-3 acetoxymethyl ester for 30 min at 37 °C and their fluorescence was measured on a FACSCalibur (BD Biosciences, Heidelberg, Germany) flow cytometer (Jurkat) or on a Tecan Ultra 384 fluorescence well plate reader (Raw 264.7, PBMC), using the same wavelengths as above. Free zinc concentrations were calculated using a dissociation constant for the Zn2+–FluoZin-3 complex of 8.9 nM,4 determining the minimal and maximal fluorescence after addition of TPEN (50 μM) or a combination of Zn2+ (100 μM) and the ionophore pyrithione (50 μM) for at least 15 min.
Atomic absorption spectrometry (AAS)
PBMC (2 × 106) were incubated as indicated in the respective figure legend for 30 min in a buffer containing 25 mM HEPES, pH 7.35, 120 mM NaCl, 5.4 mM KCl, 5 mM glucose, 1.3 mM CaCl2, 1 mM MgCl2, and 1 mM NaH2PO4. Cells were dissolved in a mixture (1
:
1) of ultrapure HNO3 (67%) and H2O2 (30%) and dried at 85 °C overnight. The residue was dissolved in ultrapure water containing 0.2% (v/v) HNO3. Samples were analyzed by flame AAS on a Perkin Elmer AAnalyst 800 instrument.
Western blot
Western blot analysis was performed as described.28 Antibodies against phosphorylated p38 (phos p38, Thr180/Tyr182) and total β-actin were obtained from New England Biolabs, Germany. Densitometry was performed using ImageJ (http://rsb.info.nih.gov/ij/index.html).
Statistical analysis
Statistical significance was calculated by one- or two-way Analysis of Variance (ANOVA), depending on the number of variables, followed by the Bonferroni post hoc test, using GraphPad Prism Version 5.01 (GraphPad Software, USA). A p value ≤0.05 was considered statistically significant. All experiments have been performed at least three times independently with comparable results.
Notes and references
- W. Maret, BioMetals, 2009, 22, 149–157 CrossRef CAS PubMed.
- H. Haase and W. Maret, Exp. Cell Res., 2003, 291, 289–298 CrossRef CAS.
- R. A. Colvin, W. R. Holmes, C. P. Fontaine and W. Maret, Metallomics, 2010, 2, 306–317 RSC.
- A. Krezel and W. Maret, JBIC, J. Biol. Inorg. Chem., 2006, 11, 1049–1062 CrossRef CAS PubMed.
- W. Bal, M. Sokolowska, E. Kurowska and P. Faller, Biochim. Biophys. Acta, 2013, 1830, 5444–5455 CrossRef CAS PubMed.
- B. J. Scott and A. R. Bradwell, Clin. Chem., 1983, 29, 629–633 CAS.
- M. Schmidt, B. Raghavan, V. Muller, T. Vogl, G. Fejer, S. Tchaptchet, S. Keck, C. Kalis, P. J. Nielsen, C. Galanos, J. Roth, A. Skerra, S. F. Martin, M. A. Freudenberg and M. Goebeler, Nat. Immunol., 2010, 11, 814–819 CrossRef CAS PubMed.
- S. L. Sensi, P. Paoletti, A. I. Bush and I. Sekler, Nat. Rev. Neurosci., 2009, 10, 780–791 CrossRef CAS PubMed.
- J. Vas and M. Monestier, Ann. N. Y. Acad. Sci., 2008, 1143, 240–267 CrossRef CAS PubMed.
- C. Driessen, K. Hirv, N. Wellinghausen, H. Kirchner and L. Rink, J. Leukocyte Biol., 1995, 57, 904–908 CAS.
- R. A. Bozym, F. Chimienti, L. J. Giblin, G. W. Gross, I. Korichneva, Y. Li, S. Libert, W. Maret, M. Parviz, C. J. Frederickson and R. B. Thompson, Exp. Biol. Med., 2010, 235, 741–750 CrossRef CAS PubMed.
- H. L. Clever, M. E. Derrick and S. A. Johnson, J. Phys. Chem. Ref. Data, 1992, 21, 941–1004 CrossRef CAS PubMed.
- S. Cooper, FASEB J, 2003, 17, 333–340 CrossRef CAS PubMed.
- I. J. Davies, M. Musa and T. L. Dormandy, J. Clin. Pathol., 1968, 21, 359–363 CrossRef CAS PubMed.
- G. R. Magneson, J. M. Puvathingal and W. J. Ray Jr., J. Biol. Chem., 1987, 262, 11140–11148 CAS.
- P. Zhang and J. C. Allen, J. Nutr., 1995, 125, 1904–1910 CAS.
-
R. Cousins, Systemic Transport of Zinc, in Zinc in Human Biology, ed. C. Mills, Springer, 1989, pp. 79–93 Search PubMed.
- A. Peretz, J. Neve, O. Jeghers and F. Pelen, Am. J. Clin. Nutr., 1993, 57, 690–694 CAS.
- J. Lu, A. J. Stewart, D. Sleep, P. J. Sadler, T. J. Pinheiro and C. A. Blindauer, J. Am. Chem. Soc., 2012, 134, 1454–1457 CrossRef CAS PubMed.
- J. K. Chesters and M. Will, Br. J. Nutr., 1981, 46, 111–118 CrossRef CAS.
- J. Kaltenberg, L. M. Plum, J. L. Ober-Blobaum, A. Honscheid, L. Rink and H. Haase, Eur. J. Immunol., 2010, 40, 1496–1503 CrossRef CAS PubMed.
- E. C. Tibaduiza and D. J. Bobilya, J. Cell. Physiol., 1996, 167, 539–547 CrossRef CAS.
-
W. Verweij, CHEAQS pro (CHemical Equilibria in AQuatic Systems), 2012, http://home.telfort.nl/cheaqs/index.html Search PubMed.
- J. C. King, D. M. Shames and L. R. Woodhouse, J. Nutr., 2000, 130, 1360S–1366S CAS.
- W. Maret, Biochemistry, 2004, 43, 3301–3309 CrossRef CAS PubMed.
- R. A. Mayer and H. A. Godwin, Anal. Biochem., 2006, 356, 142–144 CrossRef CAS PubMed.
- M. E. Legare, R. Barhoumi, E. Hebert, G. R. Bratton, R. C. Burghardt and E. Tiffany-Castiglioni, Toxicol. Sci., 1998, 46, 90–100 CAS.
- H. Haase, J. L. Ober-Blobaum, G. Engelhardt, S. Hebel, A. Heit, H. Heine and L. Rink, J. Immunol., 2008, 181, 6491–6502 CrossRef CAS.
- G. Grynkiewicz, M. Poenie and R. Y. Tsien, J. Biol. Chem., 1985, 260, 3440–3450 CAS.
- H. Haase, S. Hebel, G. Engelhardt and L. Rink, Anal. Biochem., 2006, 352, 222–230 CrossRef CAS PubMed.
|
This journal is © The Royal Society of Chemistry 2015 |
Click here to see how this site uses Cookies. View our privacy policy here.