In situ Nd isotope analyses in geological materials with signal enhancement and non-linear mass dependent fractionation reduction using laser ablation MC-ICP-MS†
Received
24th July 2014
, Accepted 7th October 2014
First published on 7th October 2014
Abstract
To improve the precision and accuracy of in situ Nd isotope analyses in geological samples, high analytical sensitivity and minimized non-linear mass dependent fractionation are desirable. In this study, we investigated the effects of three different cone combinations and the addition of N2 with the guard electrode, either grounded or floating, on the performance of Nd isotope analyses. Signal enhancements (2.5–3 folds) were observed for the Jet sample cone + X skimmer cone and for the standard sample cone + X skimmer cone, which were compared to those of the standard sample cone + H skimmer cone with the addition of N2 at 3 or 6 ml min−1. The influence of a guard electrode on the LA-MC-ICP-MS sensitivity was minimal for dry plasma conditions but became significant in the presence of N2. The addition of 3 or 6 ml min−1 N2 into the carrier gas increased the sensitivity for Nd by a factor of 1.6 with the GE-on mode for the Jet sample cone + X skimmer cone. However, in the GE-off mode, the addition of 2–3 ml min−1 N2 decreased the signal intensity of Nd by a factor of two to three. The makeup gas flow rate was found to have a significant effect on the mass bias stability of Nd isotopes, and this effect was different for different cone combinations. To stabilize the mass bias, measurements at elevated makeup gas flow rates was required for the combination of the X skimmer cone with the standard sample cone or Jet sample cone, at the expense of sensitivity. In notable contrast, the mass bias was stable for the combination of the standard sample cone and H skimmer cone under their corresponding optimum makeup gas flow rates. However, we found that the neodymium isotopes exhibited a large non-linear component at elevated makeup gas flow rates that could not be corrected by the accepted mass fractionation laws. The addition of 3–6 ml min−1 nitrogen to the central gas flow in laser ablation MC-ICP-MS was found to not only significantly enlarge the mass bias stability zone, but also to suppress the non-linear mass dependent fractionation for the combination of X skimmer cone with Jet sample cone or standard sample cone. The accuracy and precision of the developed analytical method for Nd isotope using Jet sample cone + X skimmer cone with the addition of nitrogen has been demonstrated by analyzing a series of reference glasses (JNdi-1 glass, LREE glass, NIST 610) and minerals (apatite, monazite and titanite). Results obtained for 143Nd/144Nd ratios in these reference materials are in excellent agreement with published values, demonstrating the capability of the developed analytical method to be an important tool for providing high-quality in situ Nd isotope data in geological samples.
Introduction
The Sm–Nd isotopic system and the isotopic compositions of neodymium (Nd) have been widely applied in the geological sciences as a geochronometer and isotopic tracer for the understanding of the crust–mantle evolution of the earth.1,2 Sm–Nd isotope determinations are conventionally obtained from bulk rock or mineral dissolutions using ion-exchange chromatography. Although conventional isotope measurements (e.g., ID-TIMS and MC-ICP-MS) can achieve high precision (0.001–0.002% for the best data),3 potentially useful and important geochemical information preserved at the sub-grain scale is lost.4 Moreover, ID-TIMS requires sample preparation that is time consuming.5 In contrast, the LA-MC-ICP-MS technique could provide direct and fast in situ Nd isotope analysis, as firstly reported by Foster and Vance,4 for geological materials with relatively high Nd concentrations (≥100 ppm; e.g., apatite, titanite, and ferromanganese nodules). It is well known that light rare earth element (LREE)-enriched minerals have the capability of recording the complex geological history of their host rocks.6 Recent work has demonstrated that LA-MC-ICP-MS is suitable for in situ Nd isotope measurements and for obtaining both accurate and precise results in LREE-enriched accessory minerals such as monazite, allanite, apatite, perovsikte, eudialyte loparite and titanite.4,7–12 The development of linking in situ U–Th–Pb data with Nd isotope data obtained at the same scale of analysis would provide an important tool for investigating the origin and evolution of crustal melts and fluids.7,13–15
The in situ analysis of Nd isotopes provides advantages for documenting complex growth histories, such as time-integrated Nd isotope ratios. However, LA-MC-ICP-MS is not always sufficient for geological dating and tracing at high spatial resolution analysis due to its limited sampling amount. To improve the precision and accuracy of in situ Nd isotope analysis at high spatial resolution, high sensitivity is desirable. The strategies for signal enhancement include modifications to the geometry of the sample and skimmer cones,16–18 the use of a guard electrode19 and the addition of gas (e.g., H2 and N2) to the cooling, auxiliary or central gas flow.20,21 A newly designed sample cone (Jet cone) and skimmer cone (X cone) have been developed to improve the sensitivity of Neptune MC-ICP-MS.22 Makishima and Nakamura23 have reported sensitivity improvements by 1.6 and 3 times for Hf and Pb, respectively, using solution-MC-ICP-MS and Jet cone. Iizuka and Hirata24 have reported sensitivity improvements by a factor of 2–3 for Hf, Lu and Yb when N2 was added into the carrier gas at a rate of 4 ml min−1. This N2 mixing technique was successfully used to improve the signal intensity and to attenuate the mass-independent fractionation effects for the in situ Hf isotope microanalysis of zircon using LA-MC-ICP-MS.17 Ground electrode (GE) inserted between the load coil and the quartz torch is a standard configuration for the Neptune MC-ICP-MS. With the GE-on mode, 10-fold and 3-fold enhancements in sensitivity were obtained with the use of a standard spray chamber and a dry plasma with a desolvation system, respectively,25 but the use of this mode might lead to a higher yield of polyatomic oxide and hydroxide ions19 and MO+/M+ ratios (M for REE).26 It was observed by Kimura et al.27 that Sr isotope ratio measurements with LA-MC-ICP-MS was very unstable with the use of high-sensitivity mode (Jet sample cone and X skimmer cone, and GE-on setting). Thus, the authors involved in this study suggest not applying any of the high-sensitivity settings for in situ Sr isotope analyses, despite the fact that high-sensitivity settings can significantly improve the signal intensity.
Furthermore, to obtain accurate Nd isotope measurements by MC-ICP-MS, the proper correction of the mass discrimination effect is required because mass discrimination observed in MC-ICP-MS is typically larger than that observed in TIMS.3 In addition, non-linear mass bias has been reported for Nd isotopes.3,16,28,29 The mechanisms of mass discrimination and non-linear mass bias in MC-ICP-MS remain unclear and are under debate by researchers.30,31 The conventional view suggests that mass-discrimination observed in MC-ICP-MS originates in the area of the interface—the sample and skimmer cones.3,32 Different sample and skimmer cone combinations behave differently on the instrumental mass fractionation and non-linear mass bias because of the geometry, construction and material of the cones.16,17,33 Nd isotope analysis by LA-MC-ICP-MS has been made complicated by the presence of the non-linear mass bias found in Nd isotopes.26,29 Kimura et al.26 found that a low-oxide molecular interface setup (normal sample and skimmer cones with the GE-off mode) required no external mass bias correction (standardless analysis) for Sm and Nd isotope analyses with a sacrifice of the instrumental sensitivity by ∼70%.
In the present study, we systematically investigated the effects of three different cone combinations, the addition of N2 in the central channel gas and the ICP parameters on the Nd isotope analysis by LA-MC-ICP-MS. The aim of this study was to obtain precise and accurate in situ Nd isotopic data from geological samples with high spatial resolution. In this study, a new analytical protocol for the enhancement of analytical sensitivity, improvement of mass bias stability and reduction of non-linear mass dependent fractionation is presented.
Experimental
Instrumentation
The results presented here were obtained using a Neptune Plus MC-ICP-MS (Thermo Fisher Scientific, Germany) combined with a Geolas 2005 excimer ArF laser ablation system (Lambda Physik, Göttingen, Germany) at the State Key Laboratory of Geological Processes and Mineral Resources, China, University of Geosciences in Wuhan, for a period of approximately one and a half years. The Neptune Plus with a double-focusing multi-collector ICP-MS is equipped with seven ion counters, eight motorized Faraday cups and one fixed central Faraday cup that can be exchanged with an ion counter. The maximum distance between the outermost cup positions corresponds to a relative mass range of ∼17%. Nine Faraday cups are used to monitor the isotopes (summarized in Table 1).
Table 1 Operating conditions and measurement parameters
Neptune plus MC-ICP-MS |
RF power |
1250 W |
Plasma gas flow rate |
16.0 min−1 |
Auxiliary gas flow rate |
1.1 min−1 |
Interface cones |
(a) Standard sample cone + H skimmer cone |
(b) Standard sample cone + X skimmer cone |
(c) Jet sample cone + X skimmer cone |
Instrument resolution |
∼400 (low) |
Detection system |
Nine Faraday collectors |
Integration time |
1 s, total time 80 s |
Mass analyzer pressure |
5–9 × 10−9 mbar |
Masses analyzed in Faradays |
142(Ce + Nd), 143(Nd), 144(Nd + Sm), 145(Nd), 146(Nd), 147(Sm), 148(Nd + Sm), 149(Sm), 150(Nd + Sm) |
![[thin space (1/6-em)]](https://www.rsc.org/images/entities/char_2009.gif) |
GeoLas 2005 laser ablation system
|
Wavelength |
193 nm, excimer laser |
Pulse length |
15 ns |
Energy density |
5.3 J cm−2 |
Spot sizes |
32–120 μm |
Laser frequency |
3 or 8 Hz |
Ablation cell gas |
Helium (0.6 min−1) |
Makeup gas |
Argon (0.32–1.25 min−1) |
The Neptune Plus is a major upgrade of the Neptune MC-ICP-MS platform. Among the new features are the use of a large dry interface pump (pumping speed of 100 m3 h−1) and the newly designed X skimmer cone and Jet sample cone. These cones are commercially available for use with the Neptune Plus MC-ICP-MS. However, the detailed information, such as the geometry and structure of these cones is confidential. Three different combinations of cones were used in this study, including an H skimmer cone + standard sample cone, an X skimmer cone + standard sample cone and an X skimmer cone + Jet sample cone.
All the laser ablations were performed using the Geolas 2005 laser ablation system, which consists of a COMPexPro 102 ArF excimer laser (wavelength of 193 nm, maximum energy of 200 mJ, and maximum pulse repetition rate of 20 Hz) and a MicroLas optical system. We used the laser frequencies of 3 and 8 Hz with various Nd concentrations, and the spot sizes used in this study ranged from 32 to 120 μm. Helium was used as the carrier gas within the ablation cell and mixed with argon (makeup gas) after the ablation cell but before introduction into the ICP. A simple T-joint was used downstream from the sample cell to add small amounts of nitrogen to the argon makeup gas flow. The nitrogen was controlled by a mass flow controller (Aalborg DFC 26 mass flow controller) with a range of up to 50 ml min−1. Prior to the acquisition of MC-ICP-MS data, the additional gas flow was increased to the desired values (3 ml min−1 or 6 ml min−1) over the course of approximately 10 min.
Four types of samples were analyzed in our experiments, including synthetic glass: JNdi-1,34 LREE,35 and NIST 610;4 apatite: Durango,35 MAD,36 Otter Lake;37 titanite: Khan,38 OLT-1;39 and monazite: Trebilcock,35 Mae Klang,35 44069.15 All the samples for laser ablation were mounted in epoxy resin and polished to expose median sections and to obtain flat surfaces. The analyses discussed in this study were acquired in single spot ablation mode and operated with low resolution slits. The Neptune Plus was operated in the static mode using 80 cycles with an integration time of 1 s per cycle. An integration time of 20 s was used for acquiring the background signal prior to ablation, and a time of 60 s was used for ablation signal acquisition. The details of the instrumental operating conditions and measurement parameters are provided in Table 1.
Correction of isobaric interferences for LA-MC-ICP-MS
The lack of chemical separation makes the correction of isobaric interferences important. The major limitation for the accurate in situ Nd isotope measurements by LA-MC-ICP-MS is the very large isobaric interference from 144Sm on 144Nd. Efforts were made by researchers to obtain an accurate isobaric correction.4,7,9,39,40 As proposed by McFarlane and McCulloch7 and Yang et al.,9 the interference-free 147Sm/149Sm = 1.08680 (ref. 41) (eqn (1)) was used to calculate the mass bias of Sm (βSm), and 144Sm/149Sm = 0.22332 (ref. 40) (eqn (2)) was used to calculate the interference of 144Sm using an exponential corrected for mass bias as follows: | 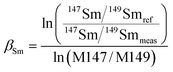 | (1) |
| 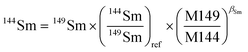 | (2) |
| 144Nd = 144total − 144Sm | (3) |
A second isobaric interference is 142Ce on 142Nd. To calculate the interference of 142Ce, the monitoring of another Ce isotope, 140Ce, is required to obtain Ce correction. Unfortunately, Ce concentration in apatite is typically too high which prevents the monitoring of Ce isotopes while maintaining adequate Nd signals.4 Consequently, the 142Nd/144Nd ratio was not used for the mass bias correction of neodymium. In addition, barium oxides can interfere at the masses of 146 (130Ba16O), 148 (132Ba16O), and 150 (134Ba16O); barium nitrides can interfere at a mass of 144 (130Ba14N on 144Nd); and REE hydride can interfere at a mass of 143 (142Ce1H on 143Nd).4 Typical Ba/Nd ratios in LREE- or MREE-enriched minerals are less than ∼0.004%.7,42 Although high Ba concentrations were reported in hydrothermal overgrowths on titanite,43 the low natural isotopic abundances of 130Ba (0.106%) and 132Ba (0.102%) preclude the significant generation of Ba-oxide.35 Foster and Vance4 have shown that 130Ba16O is negligible for Ba/Nd as high as 10 and resulted in no significant impact on 143Nd/144Nd at oxide production rates (measured using 238U16O/238U) of 3%. They also found that even with relatively large amounts of nitrogen gas added, the interference of BaN+ was negligible. Therefore, molecular interferences generated by Ba on Nd are generally considered to be insignificant, and no corrections are made for these interferences.
Nd isotope mass bias correction for LA-MC-ICP-MS
It is widely accepted that the exponential law is the most compatible for correcting the mass bias of Nd isotopes, and the correction factor for mass bias can be obtained by different isotope ratios, such as 146Nd/144Nd, 146Nd/145Nd, 142Nd/144Nd, 146Nd/142Nd or 145Nd/142Nd.3 Vance and Thirlwall3 suggested that the route for obtaining more accurate and precise isotope ratios was to normalize the ratio that was as close as possible in average mass to that of the ratio of interest. For example, the value of the 143Nd/144Nd ratio (average mass = 143.5), obtained using 142Nd/145Nd (average mass = 143.5) as a normalizing ratio, was the most identical to the TIMS value. In the case of in situ Nd measurements, the determination of 142Nd is complicated by significant 142Ce interference, and would require the monitoring of multiple Ce isotopes to implement the Ce interference and mass bias correction. However, there are no effective techniques used for reducing the 142Ce interference on 142Nd to insignificant levels, in particular for LREE-enriched accessory minerals. Because the interference of Ce is more significant than the interference of Sm for apatites, titanites and monazites,35 we have used 146Nd/144Nd rather than 142Nd/145Nd to normalize the 143Nd/144Nd ratio and 145Nd/144Nd = 0.348415 (ref. 44) as a quality control check on the mass bias. In our study, the 143Nd/144Nd ratios were corrected for mass discrimination effects by normalizing to the known invariant ratio of 146Nd/144Nd = 0.7219 (ref. 45 and 46) (eqn (4)) using an exponential law46 (eqn (5)) as follows: | 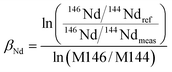 | (4) |
| 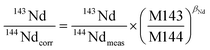 | (5) |
Results and discussion
Effect of interface cones and nitrogen on signal intensity
Fig. 1 illustrates the maximum signal intensities of 146Nd (measured at their corresponding optimum makeup gas flow rates) from JNdi-1 glass for both the normal and the nitrogen modes using the three different sample and skimmer cone combinations. An improvement in analytical sensitivity was observed when using the high-efficiency cones, compared to the standard cones. Nitrogen showed a negligible enhancement of signal intensity for the standard sample cone + H skimmer cone but showed significantly improved signal intensity for the high-efficiency cones. Without the addition of nitrogen, increases in signal intensity of ∼17% (standard sample cone + X skimmer cone) and ∼75% (Jet sample cone + X skimmer cone), respectively, were observed compared to the standard sample cone + H skimmer cone. When nitrogen was added at 3 ml min−1, the signal enhancements of 2.8-fold for the Jet sample cone + X skimmer cone and 2.5-fold for the standard sample cone + X skimmer cone were observed, relative to that of the standard sample cone + H skimmer cone. When nitrogen was added at 6 ml min−1, the signal enhancements of 3-fold for the Jet sample cone + X skimmer cone and 2.6-fold for the standard sample cone + X skimmer cone were observed, relative to that of the standard sample cone + H skimmer cone.
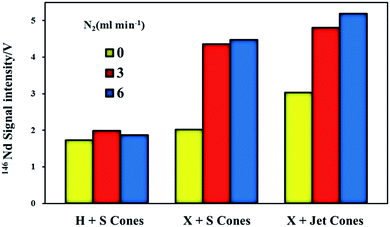 |
| Fig. 1 The integrated average signal intensities of 146Nd from JNdi-1 glass using a spot size of 44 μm for the three different sample and skimmer cone combinations obtained in both the normal and the nitrogen (N2 = 3 and 6 ml min−1) modes at their corresponding optimum makeup gas flow rates. | |
Hu et al.17 hypothesized that the maximum signal intensity was observed using the Jet sample cone and X skimmer cone because of the increased height of the Jet sample cone compared to that of the standard sample cone, which resulted in the Jet sample cone tipping closer to the torch in comparison to the standard sample cone mode. Flamigni et al.47 suggested that the Jet cone had a larger orifice diameter (1.2 mm) than the standard cone (1.0 mm); thus, it had an approximately 1.3-fold higher ion extraction efficiency relative to the standard sample cone mode. The N2-related intensity enhancement may result from increased temperature48 and/or thermal conductivity49 or from a wider and more diffuse ICP central channel.48
Effect of guard electrode (GE) on signal intensity
A guard electrode provides lower plasma offset potentials, secondary discharge effects, narrower ion energy distributions and higher ion transmission efficiencies, which can enhance the signal intensity.50,51 The signal intensities of 146Nd as a function of the makeup gas flow rate in the normal and nitrogen (N2 = 2, 3 and 6 ml min−1) modes with the guard electrode either grounded or floating are presented in Fig. 2. Two experiments with each set of operating parameters were performed on the same day.
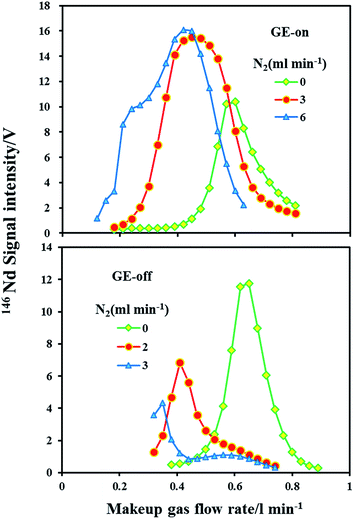 |
| Fig. 2 The integrated average signal intensities of 146Nd in JNdi-1 glass obtained by LA-MC-ICP-MS using a spot size of 60 μm as a function of the makeup gas flow rate for the Jet sample cone with X skimmer cone at different levels of nitrogen (0, 2, 3 and 6 ml min−1) with or without the GE-on mode. | |
It is clear that under the normal mode (N2 = 0 ml min−1), the guard electrode either grounded or floating did not significantly influence the sensitivity for 146Nd. As evident under the nitrogen mode, the guard electrode had a significant effect on the Nd signal intensity. Compared to the normal mode, the maximum sensitivity for 146Nd increased by a factor of 1.5–1.6 in the nitrogen (N2 = 3 and 6 ml min−1) mode with the guard electrode grounded. In significant contrast, with the guard electrode floating, the maximum sensitivity for 146Nd decreased by factors of 1.7 and 2.7 with the addition of nitrogen at 2 ml min−1 and 3 ml min−1, respectively. Hutton and Eaton52 found that positive ions have a narrow, low energy distribution (ca. 4–5 eV) in dry plasma. Thus, the use of a guard electrode may not enhance signals in dry plasma. The effect of the guard electrode on the LA-MC-ICP-MS sensitivity in the presence of N2 is significantly different. This difference may result from the change in the ion kinetic energy distribution. The addition of nitrogen to plasma significantly increases and broadens the ion kinetic energy distributions, which reduces the ion transportation efficiency and alleviates the signal enhancement effect induced by nitrogen.49 Compared with the GE floating mode, the GE grounded mode suppresses the change in the ion energy distribution in the presence of nitrogen, which most likely accounts for this phenomenon.
Intensity versus precision
Analytical precision is strongly dependent upon the intensity of the Nd signal, which is related to the Nd content in the analyzed target material and the amount of sample consumed during analysis. Fig. 3 plots the 146Nd intensity (the average signal of a single spot analysis) versus the internal uncertainty (2σ) on individual 143Nd/144Nd isotope ratio measurements for in situ Nd isotope analyses using the three different cone combinations in this study. Clearly, measurement precision for 143Nd/144Nd significantly increased as 146Nd signal intensity increased from 0 to 0.5 V, followed by more gradual improvements at higher signal intensities. The correlation between the precision of 143Nd/144Nd (2σ) and the signal intensity of 146Nd presents an exponential function (Fig. 3). Under the chosen instrument conditions, the well within-run precision (2σ) obtained for 143Nd/144Nd was approximately 25–35 ppm (Fig. 3). These data strongly suggest that efforts should be made to improve the signal intensities above 4 V for laser ablation analyses.
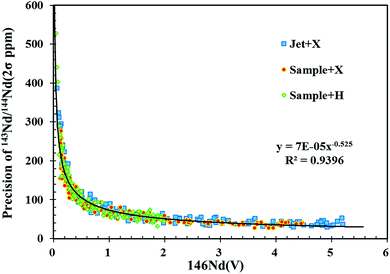 |
| Fig. 3 Plot of 146Nd intensity (the average signal of single spot analysis) versus internal uncertainty for individual 143Nd/144Nd (2σ) isotope ratio measurements of JNdi-1 glass using three different sample and skimmer cone combinations. | |
By increasing the spot size or the laser ablation energy density, signal intensity can be significantly improved. However, these approaches are at the expense of the spatial and depth resolution and are ineffective for in situ mineral analysis due to limited sample size. To overcome these limitations, it is necessary to increase the analytical sensitivity of the MC-ICP-MS instrument itself. As illustrated in Fig. 1, the use of the newly designed X skimmer cone and Jet sample cone in combination with the addition of nitrogen could improve sensitivity by 2.5–3 folds for Nd, compared to the use of the standard sample cone and H skimmer cone. The same level of precision (2σ) for 143Nd/144Nd in NIST 610 was obtained by the use of a smaller spot size of ∼70 μm under X skimmer cone and nitrogen mode, compared to the use of a larger spot size of 120 μm under standard sample cone mode. Therefore, it is clear that signal enhancement is an attractive and important way to improve precision, and to increase spatial resolution for the minerals containing a low amount of Nd, when LA-MC-ICP-MS is used for measurements.
Effect of makeup gas flow rate on measured Nd isotopes for JNdi-1 glass
Fig. 4 shows the effect of the makeup gas flow rate on the determined 143Nd/144Nd ratios in JNdi-1 glass for both the normal and the nitrogen modes (3 and 6 ml min−1) using three different sample and skimmer cone combinations. The JNdi-1 glass was used as the Nd isotope ratio standard. This glass has a low abundance of neighboring elements (Ce and Sm) and a high 143Nd/144Nd ratio (0.512115 ± 0.000007), which is closer to those of chondritic and mantle-derived materials.34 All the three combinations of interface cones were found to contribute to a non-linear mass dependent fractionation, which could not be corrected using the standard exponential laws (eqn (4) and (5)) in either normal or N2 addition mode at high makeup gas flow rate. The deviations could not be attributed to an incorrect interference calibration of 144Sm on 144Nd because there is a negligible amount of Sm in JNdi-1 glass.35 In addition, Ba concentration is low (<10 ppm), and thus interferences on 146Nd (Ba130O16) is negligible. Therefore, large deviations observed here may be due to the different behaviors of neodymium isotopes in LA-MC-ICP-MS. It is evident that the measured 143Nd/144Nd ratios significantly decreased at higher makeup gas flow rates under different cones and nitrogen modes.
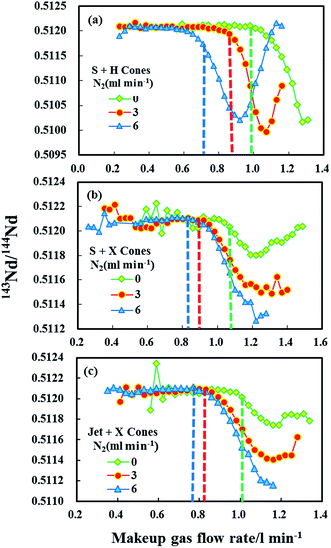 |
| Fig. 4 Effect of makeup gas flow rate on 143Nd/144Nd ratio obtained in JNdi-1 glass using three different sample and skimmer cone combinations: (a) H skimmer cone + standard sample cone; (b) X skimmer cone + standard sample cone; (c) X skimmer cone + Jet sample cone; in both the normal and the nitrogen (N2 = 3 and 6 ml min−1) modes. The vertical dashed lines show their optimum makeup gas flow rates for the normal and the nitrogen (3 and 6 ml min−1) modes that yielded the maximum signal intensity for Nd+. | |
For the standard sample cone and H skimmer cone in the normal mode, accurate 143Nd/144Nd ratio (∼10 ppm) can be obtained at its corresponding optimum makeup gas flow rate for Nd intensity. With the addition of N2 at 3 ml min−1, the deviation of ∼160 ppm in 143Nd/144Nd ratio was observed at its optimum makeup gas flow rate for Nd intensity. The largest deviation (∼370 ppm) in 143Nd/144Nd ratio was observed at the optimum central gas flow rate for Nd intensity when N2 was added at 6 ml min−1.
For the standard sample cone and X skimmer cone in the normal mode, contrary to the above observation, a deviation of ∼80 ppm in 143Nd/144Nd ratio was observed at its optimum makeup gas flow rate for Nd intensity. With the nitrogen mode (3 or 6 ml min−1), negligible deviations from the reference values by approximately 15–25 ppm were obtained for 143Nd/144Nd ratios. As clearly shown in Fig. 4, the measured 143Nd/144Nd ratios significantly varied at low makeup gas flow rates (0.6–0.8 l min−1) under normal mode. Fortunately, with the addition of nitrogen, better 143Nd/144Nd ratios were obtained. More the nitrogen was added, the more stable and accurate 143Nd/144Nd ratios were obtained.
For the Jet sample cone and X skimmer cone in normal mode, similar results were obtained as compared to standard sample cone and X skimmer cone. A relatively inaccurate 143Nd/144Nd ratio (∼100 ppm) was obtained at its corresponding optimum makeup gas flow rate for Nd intensity. In the 3 ml min−1 nitrogen mode, a negligible deviation (∼25 ppm) was obtained, whereas a negligible and smaller deviation (∼10 ppm) was obtained with 6 ml min−1 nitrogen mode.
As can be seen in Fig. 4, the neodymium isotope ratios measured using the X cone displayed larger non-linear mass bias compared to those obtained using the H cone, similar to that reported in 2012 by Newman.29 The magnitude of the non-linear mass bias observed for W isotopes in another recent study was about 50% smaller with the use of the high-sensitivity X cone compared to the H cone.33 In the case of Hf isotopes, the use of the high-sensitivity Jet sample cone contributes to a non-linear mass dependent fractionation, but this can be overcome to a certain extent by the addition of nitrogen.17 Nd isotope ratio measurements using the X cone on Neptune29 or a high-sensitivity skimmer cone on Nu Plasma MC-ICP-MS instruments16 exhibited large deviations (up to ∼750 ppm for Nd) from the reference values. These deviations are not a linear function of mass, and therefore could not be corrected using the standard mass fractionation laws. For Nd isotope analysis, a clear relationship between non-linear instrumental mass fractionation and an increase in the formation of NdO+ has been obtained.16 Newman et al.16 reported that a relatively small deviation in the measured Nd isotope ratios from the true value could be observed when the NdO+/Nd+ ratio was reduced by changing the plasma operation conditions or by adding a small quantity of N2 to the carrier gas flow (it is well known that the addition of nitrogen may reduce the oxide ratio49). Similar results were observed for the Jet sample cone + X skimmer cone or the standard sample cone + X skimmer cone in this study. However, this was not the case for the standard sample cone + H skimmer cone because biased 143Nd/144Nd ratios were obtained at the optimum makeup gas flow rates with the addition of nitrogen. These observations suggest that the increased NdO+/Nd+ ratio may not be the only reason for the inaccurate Nd isotope ratios obtained; it could be influenced by a specific cone. To date, the non-linear mass-dependent fractionation occurring in MC-ICP-MS is not fully understood. Further investigations are required to identify the specific mechanisms in the future.
Linear and non-linear mass dependent fractionation of Nd isotopes
The greater inaccuracy in the 143Nd/144Nd ratio leads one to suspect an inappropriate use of the mass bias correction because of the large mass difference between the numerator and denominator isotopes for this ratio.3 Using the exponential law for normalization, the mass bias factor (β or f) is defined as eqn (6).42,46 | 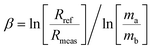 | (6) |
where Rref and Rmeas are the accurate and measured isotope ratios of the two isotopes a and b; ma and mb are the respective atomic masses of these isotopes and β is a correction factor called the mass bias factor.
Fig. 5 reveals the changes in the different mass bias factors (β) of Nd isotopes calculated from various 143Nd/144Nd, 145Nd/144Nd, 146Nd/144Nd, 145Nd/143Nd and 146Nd/143Nd ratios as a function of the sample makeup gas flow rate in the three different sample and skimmer cone combinations, both in the normal and the nitrogen modes (N2 = 3 and 6 ml min−1) obtained in the glass standard JNdi-1. As evident, the calculated different mass bias factors (β) of Nd isotopes remained the same at low makeup gas flow rates using the three different sample and skimmer cone combinations. However, when makeup gas flow rates increased beyond optimum makeup gas flow rates (corresponding to the maximum signal intensity), the mass bias factors (β) of Nd isotopes began to deviate from each other for all the three different cone combinations. If the mass bias is a simple exponential function of mass, then these calculated beta values of Nd isotopes should be the same across the entire makeup gas flow rate range. Results presented here suggest that the mass discrimination of the Nd isotopes does not display a simple exponential dependence on mass at high makeup gas flow rates. The separation of these calculated beta values indicates the non-linear mass dependent fractionation of Nd isotopes at high makeup gas flow rates.
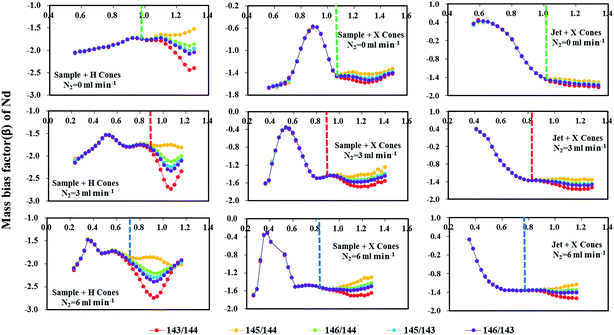 |
| Fig. 5 Effect of makeup gas flow rate on mass bias factors (β) of five Nd isotope ratios measured in JNdi-1 glass using three different sample and skimmer cone combinations: H skimmer cone + standard sample cone; X skimmer cone + standard sample cone; X skimmer cone + Jet sample cone; in both the normal and the nitrogen (N2 = 3 and 6 ml min−1) modes. The vertical dashed lines show corresponding optimum makeup gas flow rates for the normal and the nitrogen (3 and 6 ml min−1) modes that yielded the maximum signal intensity for Nd+. | |
For the combination of the H skimmer cone and standard sample cone, the calculated five different mass bias factors (β) of Nd isotopes remained the same at its optimum makeup gas flow rate (corresponding to the maximum signal intensity) in the normal mode. Unfortunately, the calculated five different mass bias factors (β) were all significantly separated from each other at their optimum makeup gas flow rates with the addition of nitrogen at 3 or 6 ml min−1. Therefore, the tuning to obtain maximum intensity for Nd isotope analyses may result in substantial variations in mass bias in the nitrogen modes. Contrary to those observed in using the H skimmer cone + standard sample cone, the five different calculated mass bias factors (β) are separated under their optimum makeup gas flow rates in the normal mode, which efficiently agree with each other for the Jet sample cone + X skimmer cone or standard sample cone + X skimmer cone with the addition of nitrogen at 3–6 ml min−1. These observed changes in the measured 143Nd/144Nd values as a function of the makeup gas flow rates (Fig. 4) can also be well-explained by the corresponding changes in the calculated mass bias factors (Fig. 5).
Fontaine et al.30 have found that an elevated carrier gas flow rate helped to stabilize the mass bias in Nu Plasma MC-ICP-MS, at the expense of sensitivity. For the combination of the standard sample cone and H skimmer cone in Neptune Plus MC-ICP-MS, the mass bias is already stable at their corresponding optimum makeup gas flow rate. It was found that stable mass-bias for Nd isotope ratios were obtained at elevated makeup gas flow rates for the combination of the X skimmer cone with standard sample cone or Jet sample cone in the normal mode. Although elevated carrier gas flow rates improved the mass bias stability, neodymium isotopes displayed larger non-linear mass bias, harmful to the accurate Nd isotope measurements using exponential law for mass bias correction. Fortunately, the addition of nitrogen at 3–6 ml min−1 to the central gas flow rate in laser ablation MC-ICP-MS not only significantly enlarged the mass bias stability zone, but it also suppressed the non-linear mass dependent fractionation for the combination of X skimmer cone with Jet sample cone or standard sample cone. As illustrated in Fig. 4 and 5, the measured 143Nd/144Nd ratios are accurate at the optimum makeup gas flow rates and the mass bias factors (β) are stable over a relatively large range of makeup gas flow rates in the presence of nitrogen at 3 and 6 ml min−1 using the Jet sample cone + X skimmer cone. Therefore, the use of high-sensitivity cones and a nitrogen addition is highly recommended for Nd isotope analysis.
LA-MC-ICP-MS analyses of Nd isotope ratios
Based on the above studies, the Jet cone and X cone combination with the addition of N2 at 6 ml min−1 in the GE grounded mode was selected for 143Nd/144Nd ratio measurements. The laser ablation spot size was adjusted depending on the Nd concentration in the target material. The long-term reproducibility was carefully evaluated through replicate measurements on international standards and potential reference materials over a period of nearly one and a half years. Table 2 summarizes the determined neodymium isotope ratios in eleven reference materials.
Table 2 Summary of LA-MC-ICP-MS results for the neodymium isotope ratios of eleven samples
Sample |
143Nd/144Nd (2SD) |
145Nd/144Nd (2SD) |
Number |
Ref. (2SD) |
Ref. (by LA, 2SD) |
The reference values are taken from Tanaka et al. (2000).34
The reference values are taken from Fisher et al. (2011).35
The reference values are taken from Liu et al. (2012).15
The reference values are taken from Woodhead and Hergt (2001).53
The reference values are taken from Foster and Vance (2006).4
The reference values are taken from Kimura et al. (2013).26
The reference values are taken from McFarlane and McCulloch (2008).8
The reference values are taken from Yang et al. (2014).55
|
Synthetic glass
|
JNdi-1 |
0.512092 ± 0.000016 |
0.348411 ± 0.000010 |
125 |
0.512115 ± 0.000007a |
0.512089 ± 0.000023b |
|
|
|
|
0.512098 ± 0.000033c |
LREE |
0.512100 ± 0.000018 |
0.348411 ± 0.000012 |
74 |
0.512115 ± 0.000007a |
0.512097 ± 0.000033b |
|
|
|
|
0.512101 ± 0.000030c |
NIST 610 |
0.511900 ± 0.000054 |
0.348401 ± 0.000028 |
72 |
0.511927 ± 0.000004d |
0.512921 ± 0.000013f |
|
|
|
0.511908 ± 0.000008e |
|
![[thin space (1/6-em)]](https://www.rsc.org/images/entities/char_2009.gif) |
Apatite
|
Durango |
0.512479 ± 0.000030 |
0.348418 ± 0.000018 |
68 |
0.512489 ± 0.0000012b |
0.512490 ± 0.000046g |
|
|
|
0.512483 ± 0.0000004e |
0.512463 ± 0.000048b |
|
|
|
|
0.512490 ± 0.000018f |
Otter Lake |
0.511919 ± 0.000020 |
0.348418 ± 0.000012 |
37 |
|
0.511942 ± 0.000045h |
MAD 1 |
0.511321 ± 0.000030 |
0.348418 ± 0.000014 |
45 |
|
0.511322 ± 0.000053h |
MAD 2 |
0.511292 ± 0.000026 |
0.348419 ± 0.000012 |
48 |
|
|
MAD 3 |
0.511294 ± 0.000032 |
0.348418 ± 0.000018 |
48 |
|
|
![[thin space (1/6-em)]](https://www.rsc.org/images/entities/char_2009.gif) |
Titanite
|
Khan |
0.511635 ± 0.000028 |
0.348415 ± 0.000018 |
60 |
|
|
OLTI |
0.512270 ± 0.000024 |
0.348422 ± 0.000016 |
64 |
|
|
![[thin space (1/6-em)]](https://www.rsc.org/images/entities/char_2009.gif) |
Monazite
|
Trebilcock |
0.512613 ± 0.000028 |
0.348420 ± 0.000008 |
105 |
0.512616 ± 0.000011b |
0.512607 ± 0.000026b |
Mae Klang |
0.512646 ± 0.000016 |
0.348422 ± 0.000012 |
111 |
0.512646 ± 0.000010b |
0.512635 ± 0.000024b |
44069 |
0.512186 ± 0.000028 |
0.348422 ± 0.000012 |
105 |
|
0.512175 ± 0.000040c |
JNdi-1 glass
A piece of JNdi-1 glass was analyzed by LA-MC-ICP-MS using laser spot sizes of 44 μm and 60 μm, yielding mean 146Nd intensities of 8.5–14 V. A total of 125 measurements were performed and the values of 0.512092 ± 0.000016 and 0.348411 ± 0.000010 (mean, 2SD) were obtained for 143Nd/144Nd and 145Nd/144Nd, respectively (Fig. 6a and b). The results obtained here were slightly lower than 143Nd/144Nd value of 0.512115 for JNdi-1 standard by Tanaka et al.,34 but within the error of the published data using LA-MC-ICP-MS by Fisher et al.35 (0.512089 ± 0.000023, 2SD) and Liu et al.15 (0.512098 ± 0.000033, 2SD). The 145Nd/144Nd ratio was in agreement with the recommended value of Wasserburg et al.44
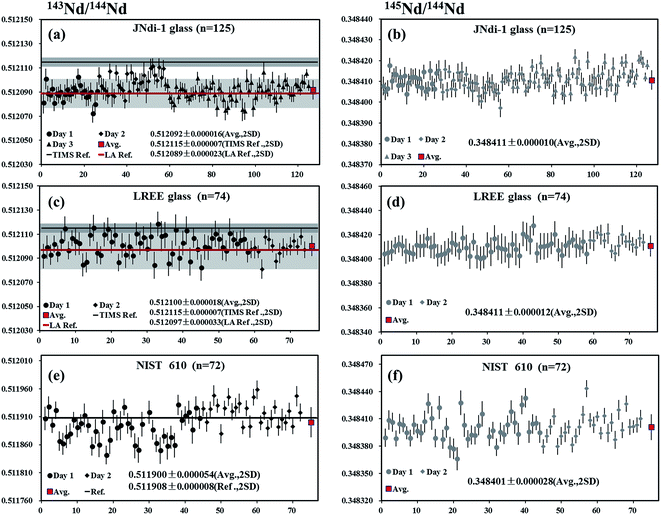 |
| Fig. 6 Measured 143Nd/144Nd and 145Nd/144Nd isotope ratios obtained in 2–3 days of synthetic glasses using LA-MC-ICP-MS. Error bars are 2SE (2 standard in-run errors). Red bars (2SD) show the mean measured Nd isotope ratios of the present study. The dark or red lines and grey shadows are the reference values of 143Nd/144Nd. | |
LREE glass
A piece of LREE glass was analyzed by LA-MC-ICP-MS using the laser spot sizes of 44 μm or 60 μm, producing 146Nd intensities in the range of 3.6–4.9 V. A total of 74 analyses yielded values of 0.512100 ± 0.000018 and 0.348411 ± 0.000012 (mean, 2SD) for 143Nd/144Nd and 145Nd/144Nd, respectively (Fig. 6c and d). These values were slightly lower than 143Nd/144Nd ratio reported by Tanaka et al.34 but in agreement with data obtained by Fisher et al.35 (0.512097 ± 0.000033, 2SD) and Liu et al.15 (0.512101 ± 0.000030, 2SD) and the natural 145Nd/144Nd ratios reported by Wasserburg et al.44
NIST 610
A piece of NIST 610 glass was analyzed by LA-MC-ICP-MS using a laser spot size of 120 μm, producing 146Nd intensities of 0.8–1.1 V. A total of 72 analyses yielded the values of 0.511900 ± 0.000054 and 0.348401 ± 0.000028 (mean, 2SD) for 143Nd/144Nd and 145Nd/144Nd, respectively (Fig. 6e and f). Results obtained here were in agreement with the results reported by Foster and Vance4 (143Nd/144Nd = 0.511908 ± 0.000008) and the natural 145Nd/144Nd value by Wasserburg et al.44 But the 143Nd/144Nd ratios determined by us are slightly lower than those reported by Woodhead and Hergt53 (0.511927 ± 0.000004) using solution MC-ICP-MS and Kimura et al.26 (0.511921 ± 0.000013) using LA-MC-ICP-MS.
Durango apatite
Durango apatite occurs within the Cerro de Mercado open-pit iron mine, located in the northern portion of Durango City, Mexico.54 A piece of Durango apatite was analyzed by LA-MC-ICP-MS using a laser spot size of 90 μm, producing 146Nd intensities in the range of 1.8–3.4 V depending on Nd concentrations within the sample. The internal precision of 143Nd/144Nd for individual analysis determined at two standard error of the mean (2SE) was ∼24 ppm (0.24 ε-unit). A total of 68 LA-MC-ICPMS analyses of Durango apatite over three analytical sessions were acquired, yielding the values of 0.512479 ± 0.000030 and 0.348418 ± 0.000018 (mean, 2SD) for 143Nd/144Nd and 145Nd/144Nd, respectively (Fig. 7a and b). The results obtained by LA-MC-ICP-MS in this study were in agreement with the value of 143Nd/144Nd obtained by Fisher et al.35 (0.512489 ± 0.000012), Kimura et al.26 (0.512490 ± 0.000018), McFarlane and McCulloch8 (0.512490 ± 0.000046) and the canonical value 0.348415 for 145Nd/144Nd by Wasserburg et al.44
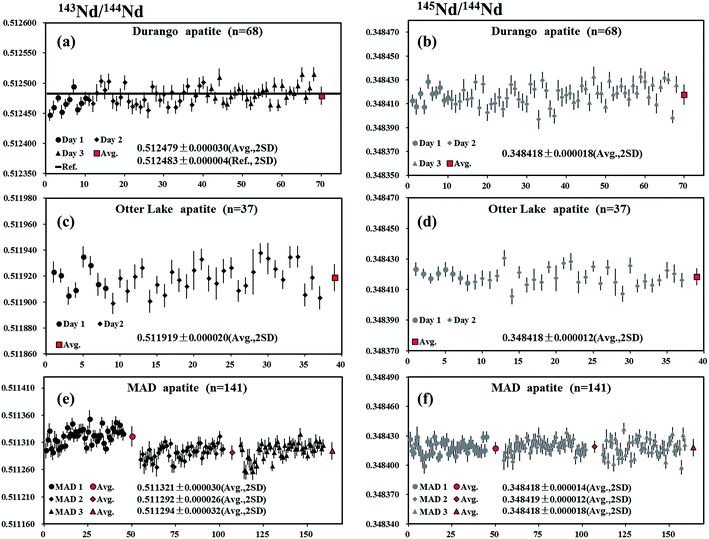 |
| Fig. 7 Measured 143Nd/144Nd and 145Nd/144Nd isotope ratios obtained in 2–3 days of apatites using LA-MC-ICP-MS. Error bars are 2SE (2 standard in-run errors). Red bars (2SD) show the mean measured Nd isotope ratios of the present study. The dark lines are the reference values of 143Nd/144Nd. | |
Otter Lake apatite
The Otter Lake apatite is a brown crystal from Québec, located north of the Bancroft domain within the Grenville province.37 A piece of Otter Lake apatite was analyzed by LA-MC-ICP-MS using laser spot sizes of 60 or 90 μm, producing 146Nd intensities of 3.5–9 V. The internal precision of 143Nd/144Nd based on individual analysis at two standard error of the mean (2SE) was ∼23 ppm (0.23 ε-unit). The values of 0.511919 ± 0.000020 and 0.348418 ± 0.000012 (mean, 2SD) for 143Nd/144Nd and 145Nd/144Nd, respectively, were obtained (Fig. 7c and d), in agreement with the value of 143Nd/144Nd obtained by Yang et al.55 (0.511942 ± 0.000045) and the natural 145Nd/144Nd value reported by Wasserburg et al.44
MAD apatite
Three pieces of blue/green gem roughs of Madagascan apatite from the “1st mine discovery” (MAD apatite)36 were analyzed using LA-MC-ICP-MS with a laser spot size of 90 μm, producing mean 146Nd intensities of 1.5–3 V. The internal precision of 143Nd/144Nd for individual analysis determined at two standard error of the mean (2SE) was ∼24 ppm (0.24 ε-unit). For the first piece (MAD1), a total of 45 analyses yielded values of 0.511321 ± 0.000030 and 0.348418 ± 0.000014 (mean, 2SD) for 143Nd/144Nd and 145Nd/144Nd, respectively. A total of 48 analyses yielded the values of 0.511292 ± 0.000026 and 0.348419 ± 0.000012 (mean, 2SD) for 143Nd/144Nd and 145Nd/144Nd, respectively, for the second piece (MAD 2). A total of 48 analyses yielded the values of 0.511294 ± 0.000032 and 0.348418 ± 0.000018 (mean, 2SD) for 143Nd/144Nd and 145Nd/144Nd, respectively, for the third piece (MAD 3) (Fig. 7e and f). Results obtained for 145Nd/144Nd ratios in the three pieces of MAD apatite were in agreement with the value of 143Nd/144Nd obtained by Yang et al.55 (0.511322 ± 0.000053) and the natural 145Nd/144Nd value reported by Wasserburg et al.44
Khan titanite
Khan titanite is a large, euhedral crystal of black titanite that occurs in copper-mineralized pegmatite at the Khan mine, Namibia.38 Two pieces of Khan titanite were analyzed by LA-MC-ICP-MS using the laser spot sizes of 60 or 90 μm, producing 146Nd intensities of 2–5 V. The internal precision of 143Nd/144Nd for individual analysis determined at two standard error of the mean (2SE) was 23 ppm (0.23 ε-unit). The values of 0.511635 ± 0.000028 and 0.348415 ± 0.000018 (mean, 2SD) for 143Nd/144Nd and 145Nd/144Nd, respectively, obtained for a total of 60 analyses, were in agreement with the natural 145Nd/144Nd value reported by Wasserburg et al.44 (Fig. 8a and b).
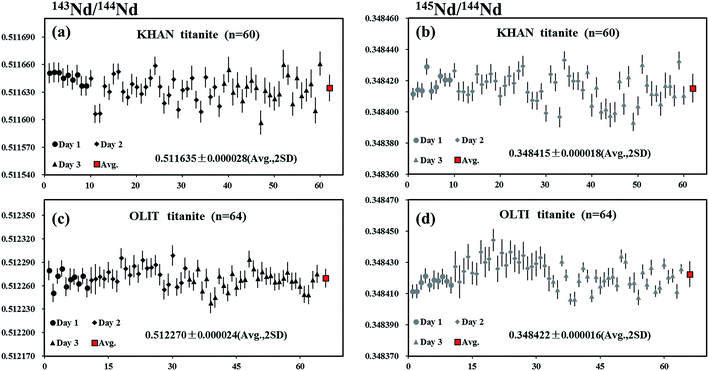 |
| Fig. 8 Measured 143Nd/144Nd and 145Nd/144Nd isotope ratios obtained in 3 days of titanites using LA-MC-ICP-MS. Error bars are 2SE (2 standard in-run errors). Red bars (2SD) show the mean measured Nd isotope ratios of the present study. | |
OLT-1 titanite
OLT-1 titanite is a dark brown titanite grain collected from the pegmatite skarns of the Grenville province of the Canadian shield.39 A piece of OLT-1 titanite was analyzed by LA-MC-ICP-MS using laser spot sizes of 60 or 90 μm, producing 146Nd intensities of 2.5–4 V. The internal precision of 143Nd/144Nd for individual analysis determined at two standard error of the mean (2SE) was ∼24 ppm (0.24 ε-unit). The values of 0.512270 ± 0.000024 and 0.348422 ± 0.000016 (mean, 2SD) for 143Nd/144Nd and 145Nd/144Nd, respectively, obtained for a total of 64 analyses, were in agreement with the natural 145Nd/144Nd value reported by Wasserburg et al.44 (Fig. 8c and d).
Trebilcock monazite
The Trebilcock monazite is obtained from an ∼270 Ma Trebilcock pegmatite, a New Hampshire pegmatite of the Northern series, which is located in the Topsham area of southwestern Maine.56 A piece of Trebilcock monazite was analyzed by LA-MC-ICP-MS using a laser spot size of 32 μm and a frequency of 3 Hz, producing 146Nd intensities of 7.2–14.4 V. The internal precision of 143Nd/144Nd for individual analysis determined at two standard error of the mean (2SE) was ∼19 ppm (0.19 ε-unit). The values of 0.512613 ± 0.000028 and 0.348420 ± 0.000008 (mean, 2SD) for 143Nd/144Nd and 145Nd/144Nd, respectively, obtained for a total of 105 analyses, were in agreement with the results obtained by Fisher et al.35 and Wasserburg et al.44 (Fig. 9a and b).
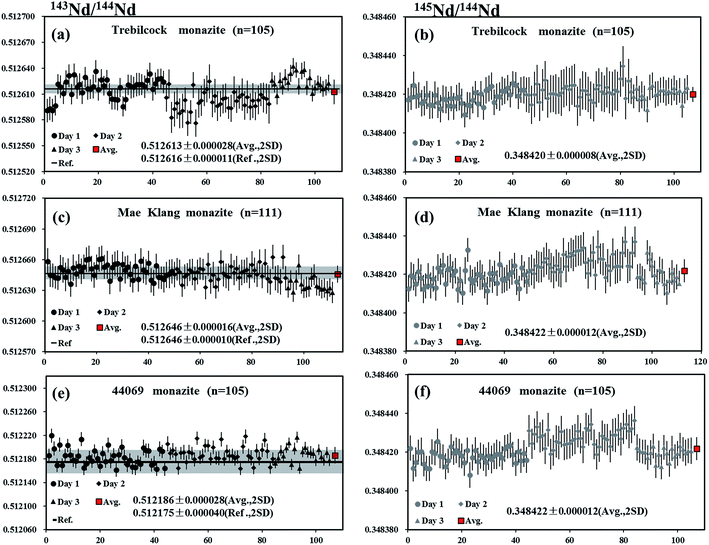 |
| Fig. 9 Measured 143Nd/144Nd and 145Nd/144Nd isotope ratios obtained in 3 days of monazites using LA-MC-ICP-MS. Error bars are 2SE (2 standard in-run errors). Red bars (2SD) show the mean measured Nd isotope ratios of the present study. The dark lines and grey shadows are the reference values of 143Nd/144Nd. | |
Mae Klang monazite
A piece of Mae Klang monazite, which is obtained from medium-grained, foliated biotite granite near the eastern margin of the Mae Klang pluton in the Doi Inthanon core complex, northern Thailand57 was analyzed by LA-MC-ICP-MS using a laser spot size of 32 μm and a frequency of 3 Hz, producing 146Nd intensities of 5.9–13 V. The internal precision on 143Nd/144Nd for individual analysis determined at two standard error of the mean (2SE) was ∼15 ppm (0.15 ε-unit). The values of 0.512646 ± 0.000016 and 0.348422 ± 0.000012 (mean, 2SD) for 143Nd/144Nd and 145Nd/144Nd, respectively, obtained for a total of 111 analyses, were within the error of the recommended value35 and the natural value of Wasserburg et al.44 (Fig. 9c and d).
44069 monazite
The 44069 monazite standard is extracted from a psammitic gneiss layer of the Wilmington Complex, northern Delaware, that occurs within a metasedimentary sequence, which is primarily composed of pelitic paragneiss and amphibolites.58 Liu et al.15 reported the values of 0.512175 ± 0.000040 and 0.348428 ± 0.000023 (mean, 2SD, n = 26) for 143Nd/144Nd and 145Nd/144Nd, respectively, in this material. A laser spot size of 32 μm and a frequency of 3 Hz, producing 146Nd intensities of 7.8–17.2 V, were used for the measurements. The internal precision of 143Nd/144Nd for individual analysis determined at two standard error of the mean (2SE) was ∼13 ppm (0.13 ε-unit). The values of 0.512186 ± 0.000028 and 0.348422 ± 0.000012 (mean, 2SD) for 143Nd/144Nd and 145Nd/144Nd, respectively, obtained for a total of 105 analyses, were in agreement with the recommended value15 of 143Nd/144Nd and for the natural 145Nd/144Nd value reported by Wasserburg et al.44 (Fig. 9e and f).
Conclusions
The results presented here indicate that selecting different sample and skimmer cones and the addition of small amounts of nitrogen to the central gas flow play important roles in improving the performance of LA-MC-ICP-MS for Nd isotope analyses. Compared to the standard configuration, the Nd signal intensity is improved by a factor of 2.5–3 with the use of the newly designed X skimmer cone and Jet sample cone in combination with the addition of nitrogen at 3–6 ml min−1. This sensitivity enhancement is important for the in situ Nd isotope analyses of geological samples using LA-MC-ICP-MS, wherein an improved precision or an increased spatial resolution is important. However, in the case of Nd, the high-sensitivity X skimmer cone and/or Jet sample cone geometry induced larger non-linear mass dependent fractionation at their corresponding optimum makeup gas flow rates (corresponding to the maximum Nd signal intensity). Fortunately, the addition of nitrogen at 3–6 ml min−1 to the central gas flow in laser ablation MC-ICP-MS is found to not only suppress this anomalous mass fractionation effect but also significantly enlarge the mass bias stability zone of the makeup gas flow rates. Our results also suggest that the guard electrode has a minimal effect on the Nd sensitivity in dry plasma, whereas it exhibits a significant effect on the Nd sensitivity in the presence of nitrogen. This study is the first to emphasize the importance of a guard electrode on the signal intensity in the presence of nitrogen for LA-MC-ICP-MS analyses. The results obtained for eleven reference standards (synthetic glass, apatite, titanite and monazite) show excellent agreement with the values reported in the literature, demonstrating the capability of the developed analytical technique for achieving reliable in situ Nd isotope measurements in geological samples.
Acknowledgements
We are grateful to Yueheng Yang and Allen K. Kennedy for providing apatite and titanite standards. Thoughtful comments by two anonymous referees have significantly improved the manuscript. This research is supported by the National Nature Science Foundation of China (Grants 41273030, 41322023, 41373026, and 41173016), the Fundamental Research Funds for National Universities, the Specialized Research Fund for the Doctoral Program of Higher Education (20130145110007), the CERS-China Equipment and Education Resources System (CERS-1-81), the State Administration of the Foreign Experts Affairs of China (B07039), and the MOST Special Fund from the State Key Laboratory of Geological Processes and Mineral Resources.
References
- R. Carlson, J. Macdougall and G. Lugmair, Geophys. Res. Lett., 1978, 5, 229–232 CrossRef CAS.
- R. Carlson and G. Lugmair, Earth Planet. Sci. Lett., 1979, 45, 123–132 CrossRef CAS.
- D. Vance and M. Thirlwall, Chem. Geol., 2002, 185, 227–240 CrossRef CAS.
- G. L. Foster and D. Vance, J. Anal. At. Spectrom., 2006, 21, 288–296 RSC.
- A. Ali and G. Srinivasan, Int. J. Mass Spectrom., 2011, 299, 27–34 CrossRef CAS PubMed.
- M. L. Williams, M. J. Jercinovic and C. J. Hetherington, Annu. Rev. Earth Planet. Sci., 2007, 35, 137–175 CrossRef CAS.
- C. McFarlane and M. McCulloch, Chem. Geol., 2007, 245, 45–60 CrossRef CAS PubMed.
-
C. McFarlane and M. McCulloch, Laser Ablation-ICPMS in the Earth Sciences, Mineralogical Association of Canada Short Course, Vancouver, BC, 2008, pp. 117–133 Search PubMed.
- Y. H. Yang, J. F. Sun, L. W. Xie, H. R. Fan and F. Y. Wu, Chin. Sci. Bull., 2008, 53, 1062–1070 CrossRef CAS.
- Y. H. Yang, F. Y. Wu, S. A. Wilde, X. M. Liu, Y. B. Zhang, L. W. Xie and J. H. Yang, Chem. Geol., 2009, 264, 24–42 CrossRef CAS PubMed.
- F. Y. Wu, Y. H. Yang, M. A. Marks, Z. C. Liu, Q. Zhou, W. C. Ge, J. S. Yang, Z. F. Zhao, R. H. Mitchell and G. Markl, Chem. Geol., 2010, 273, 8–34 CrossRef CAS PubMed.
- R. H. Mitchell, F.-Y. Wu and Y. H. Yang, Chem. Geol., 2011, 280, 191–199 CrossRef CAS PubMed.
- C. J. Gregory, C. R. McFarlane, J. Hermann and D. Rubatto, Chem. Geol., 2009, 260, 73–86 CrossRef CAS PubMed.
- Y. Amelin, Chem. Geol., 2009, 261, 53–61 CrossRef PubMed.
- Z. C. Liu, F. Y. Wu, Y. H. Yang, J. H. Yang and S. A. Wilde, Chem. Geol., 2012, 334, 221–239 CrossRef CAS PubMed.
- K. Newman, P. A. Freedman, J. Williams, N. S. Belshaw and A. N. Halliday, J. Anal. At. Spectrom., 2009, 24, 742–751 RSC.
- Z. C. Hu, Y. S. Liu, S. Gao, W. G. Liu, W. Zhang, X. R. Tong, L. Lin, K. Q. Zong, M. Li, H. H. Chen, L. Zhou and L. Yang, J. Anal. At. Spectrom., 2012, 27, 1391–1399 RSC.
- J. M. Cottle, A. J. Burrows, A. Kylander-Clark, P. A. Freedman and R. S. Cohen, J. Anal. At. Spectrom., 2013, 28, 1700–1706 RSC.
- P. K. Appelblad, I. Rodushkin and D. C. Baxter, J. Anal. At. Spectrom., 2000, 15, 359–364 RSC.
- S. F. Durrant, Fresenius' J. Anal. Chem., 1994, 349, 768–771 CrossRef CAS.
- M. Guillong and C. A. Heinrich, J. Anal. At. Spectrom., 2007, 22, 1488–1494 RSC.
- J. Schwieters, D. Tuttas, C. Bouman and N. Quaas, Geochim. Cosmochim. Acta, 2006, 70, A567 CrossRef PubMed.
- A. Makishima and E. Nakamura, J. Anal. At. Spectrom., 2010, 25, 1712–1716 RSC.
- T. Iizuka and T. Hirata, Chem. Geol., 2005, 220, 121–137 CrossRef CAS PubMed.
- M. P. Field, J. T. Cullen and R. M. Sherrell, J. Anal. At. Spectrom., 1999, 14, 1425–1431 RSC.
- J.-I. Kimura, Q. Chang and H. Kawabata, J. Anal. At. Spectrom., 2013, 28, 1522–1529 RSC.
- J.-I. Kimura, T. Takahashi and Q. Chang, J. Anal. At. Spectrom., 2013, 28, 945–957 RSC.
- T. Iizuka, S. M. Eggins, M. T. McCulloch, L. P. J. Kinsley and G. E. Mortimer, Chem. Geol., 2011, 282, 45–57 CrossRef CAS PubMed.
- K. Newman, J. Anal. At. Spectrom., 2012, 27, 63–70 RSC.
- G. H. Fontaine, B. Hattendorf, B. Bourdon and D. Günther, J. Anal. At. Spectrom., 2009, 24, 637–648 RSC.
- N. Kivel, I. Günther-Leopold, F. Vanhaecke and D. Günther, Spectrochim. Acta, Part B, 2012, 76, 126–132 CrossRef CAS PubMed.
- M. F. Thirlwall and R. Anczkiewicz, Int. J. Mass Spectrom., 2004, 235, 59–81 CrossRef CAS PubMed.
- N. Shirai and M. Humayun, J. Anal. At. Spectrom., 2011, 26, 1414–1420 RSC.
- T. Tanaka, S. Togashi, H. Kamioka, H. Amakawa, H. Kagami, T. Hamamoto, M. Yuhara, Y. Orihashi, S. Yoneda and H. Shimizu, Chem. Geol., 2000, 168, 279–281 CrossRef.
- C. M. Fisher, C. R. M. McFarlane, J. M. Hanchar, M. D. Schmitz, P. J. Sylvester, R. Lam and H. P. Longerich, Chem. Geol., 2011, 284, 1–20 CrossRef CAS PubMed.
- S. N. Thomson, G. E. Gehrels, J. Ruiz and R. Buchwaldt, Geochem., Geophys., Geosyst., 2012, 13 DOI:10.1029/2011gc003928.
- D. M. Chew, P. J. Sylvester and M. N. Tubrett, Chem. Geol., 2011, 280, 200–216 CrossRef CAS PubMed.
- L. M. Heaman, Chem. Geol., 2009, 261, 43–52 CrossRef PubMed.
- A. K. Kennedy, S. L. Kamo, L. N. Nasdala and N. E. Timms, Can. Mineral., 2010, 48, 1423–1443 CrossRef CAS.
- H. Isnard, R. Brennetot, C. Caussignac, N. Caussignac and F. Chartier, Int. J. Mass Spectrom., 2005, 246, 66–73 CrossRef CAS PubMed.
- J. Dubois, G. Retali and J. Cesario, Int. J. Mass Spectrom. Ion Processes, 1992, 120, 163–177 CrossRef CAS.
- B. Luais, P. Telouk and F. Albaréde, Geochim. Cosmochim. Acta, 1997, 61, 4847–4854 CrossRef CAS.
- S. Jung and E. Hellebrand, Chem. Geol., 2007, 241, 88–107 CrossRef CAS PubMed.
- G. Wasserburg, S. Jacousen, D. DePaolo, M. McCulloch and T. Wen, Geochim. Cosmochim. Acta, 1981, 45, 2311–2323 CrossRef CAS.
- R. O'nions, P. Hamilton and N. Evensen, Earth Planet. Sci. Lett., 1977, 34, 13–22 CrossRef.
- W. Russell, D. Papanastassiou and T. Tombrello, Geochim. Cosmochim. Acta, 1978, 42, 1075–1090 CrossRef CAS.
- L. Flamigni, J. Koch and D. Günther, Spectrochim. Acta, Part B, 2012, 76, 70–76 CrossRef CAS PubMed.
- A. E. Holliday and D. Beauchemin, Spectrochim. Acta, Part B, 2004, 59, 291–311 CrossRef PubMed.
- Z. C. Hu, S. Gao, Y. S. Liu, S. H. Hu, H. H. Chen and H. L. Yuan, J. Anal. At. Spectrom., 2008, 23, 1093–1101 RSC.
- A. L. Gray, J. Anal. At. Spectrom., 1986, 1, 247–249 RSC.
- N. S. Nonose, N. Matsuda, N. Fudagawa and M. Kubota, Spectrochim. Acta, Part B, 1994, 49, 955–974 CrossRef.
- R. C. Hutton and A. N. Eaton, J. Anal. At. Spectrom., 1987, 2, 595–598 RSC.
- J. D. Woodhead and J. M. Hergt, Geostand. Newsl., 2001, 25, 261–266 CrossRef CAS PubMed.
- F. W. McDowell, W. C. McIntosh and K. A. Farley, Chem. Geol., 2005, 214, 249–263 CrossRef CAS PubMed.
- Y. H. Yang, F. Y. Wu, J. H. Yang, D. M. Chew, L. W. Xie, Z. Y. Chu, Y. B. Zhang and C. Huang, Chem. Geol., 2014, 385, 35–55 CrossRef CAS PubMed.
- P. B. Tomascak, E. J. Krogstad and R. J. Walker, Can. Mineral., 1998, 36, 327–337 CAS.
- G. Dunning, A. Macdonald and S. Barr, Tectonophysics, 1995, 251, 197–213 CrossRef CAS.
- J. N. Aleinikoff, W. S. Schenck, M. O. Plank, L. Srogi, C. M. Fanning, S. L. Kamo and H. Bosbyshell, Geol. Soc. Am. Bull., 2006, 118, 39–64 CrossRef PubMed.
Footnote |
† This manuscript is intended for the themed issue for Barry Sharp. |
|
This journal is © The Royal Society of Chemistry 2015 |