Reducing nitrogen crossover in microbial reverse-electrodialysis cells by using adjacent anion exchange membranes and anion exchange resin†
Received
26th June 2015
, Accepted 10th August 2015
First published on 11th August 2015
Abstract
Microbial reverse electrodialysis cells (MRECs) combine power generation from salinity gradient energy using reverse electrodialysis (RED), with power generation from organic matter using a microbial fuel cell. Waste heat can be used to distill ammonium bicarbonate into high (HC) and low salt concentration (LC) solutions for use in the RED stack, but nitrogen crossover into the anode chamber must be minimized to avoid ammonia loses, and foster a healthy microbial community. To reduce nitrogen crossover, an additional low concentration (LC) chamber was inserted before the anode using an additional anion exchange membrane (AEM) next to another AEM, and filled with different amounts of anion or cation ion exchange resins. Addition of the extra AEM increased the ohmic resistance of the test RED stack from 103 Ω cm2 (1 AEM) to 295 Ω cm2 (2 AEMs). However, the use of the anion exchange resin decreased the solution resistance of the LC chamber by 74% (637 Ω cm2, no resin; 166 Ω cm2 with resin). Nitrogen crossover into the anode chamber was reduced by up to 97% using 50% of the chamber filled with an anion exchange resin compared to the control (no additional chamber). The added resistance contributed by the use of the additional LC chamber could be compensated for by using additional LC and HC membrane pairs in the RED stack.
Water impact
A microbial reverse electrodialysis cell (MREC) simultaneously treats and generates energy from wastewater, however the treated water cannot be discharged into the environment due to a high concentration of ammonia. Modifying the MREC cell by adding an additional membrane reduces the amount of discharged nitrogen by 97%, making it a more suitable process for wastewater treatment.
|
Introduction
Microbial fuel cells (MFCs) are showing promise as a potential alternative to conventional biological wastewater treatment systems such as activated sludge.1 In an MFC, organics and nutrients in the wastewater stream are consumed by bacteria and effectively removed from the water, resulting in wastewater treatment coupled to electricity generation. Power densities produced by MFCs can be increased by placing a reverse electrodialysis (RED) stack between the electrodes, producing a configuration referred to as a microbial reverse-electrodialysis cell (MREC). A RED stack consists of alternating cells of high (HC) and low concentration (LC) salt solutions, each encompassed by alternating positively and negatively charged ion exchange membranes. Positively charged salt ions are transported from the HC solution to LC solution through the cation exchange membrane (CEM), while negatively charged salt ions are transported from the HC solution to LC solution through the anion exchange membrane (AEM). This ion transport scheme induces positive ion flow in one direction and negative ion flow in the other, creating an electrical current. The use of thermolytic salt solutions, such as ammonium bicarbonate (AmB), enables electricity generation using waste heat as the HC and LC solutions can be produced with this salt using conventional distillation processes.2,3 Power densities as high as 5.4 W m−2 (normalized by the projected surface area of the cathode) have been produced in MRECs with AmB in the RED stack and acetate as a fuel, compared to 1.1 W m−2 using only acetate in an MFC.2
One problem with AmB in an MREC is that negatively-charged carbamate (NH2CO2−), which is produced when AmB is dissolved in water, can easily pass through an AEM and into the anode chamber.2 Total ammonia nitrogen (TAN) concentrations in the anolyte were reported to reach 590 mg L−1 after 24 h in one MREC study with AmB.2 This nitrogen concentration far exceeds discharge limits allowed for TAN in a wastewater, and high losses of nitrogen would also make a closed-loop energy generation system impractical from an economical point of view. TAN concentrations above 500 mg L−1 in the anode chamber can also inhibit acetate oxidation by exoelectrogenic bacteria.4 To date, only one study has addressed nitrogen crossover in membrane stacks using AmB. It was shown that placing a CEM adjacent to the anode chamber to form an additional low concentration cell could reduce nitrogen crossover by 60% (final concentration of 311 mg L−1 TAN) without affecting energy recovery.5 However, this TAN concentration is still too high for wastewater disposal, and therefore better methods are needed to reduce nitrogen crossover for further development of MRECs.
The objective of this work was to reduce the quantity of nitrogen that is transferred into the anode chamber of an MREC when using AmB in the RED stack, without greatly impacting power production. It was hypothesized that an additional LC cell formed by using an AEM facing an AEM (as opposed to CEM) could reduce nitrogen crossover into the anode. However, this change alone would result in a large increase in internal resistance due to the low ionic conductivity of this chamber. Ion exchange resins have been used in electrodialysis stacks6 and microbial desalination cells7–10 to improve solution conductivity in LC cells. Packing this additional cell with an ion exchange resin could therefore minimize the increase in solution resistance, which would help to mitigate power losses associated with adding an additional chamber, and it might also further reduce nitrogen crossover. Nitrogen crossover was therefore examined in two- and three-chamber electrochemical systems in the presence and absence of different ion exchange resins. Tests were conducted abiotically as ion crossover is only a chemical transport process, and thus a more complex reactor containing a biotic anode was not needed to study nitrogen transport through a two membrane system.
Methods
Reactor construction
A two-chamber (2C) electrochemical reactor was constructed to examine membrane resistance and nitrogen crossover by connecting in series six reactor sections, each a 4 cm acrylic cube with a 3 cm diameter working chamber (Fig. 1a). An AEM (Selemion AMV; Asahi Glass, Japan) or CEM (Selemion CMV; Asahi Glass, Japan) was installed in the center of the reactor between two gaskets to create a tight seal between the two equal-volume anode and cathode chambers. AEMs and CEMs were equilibrated in 1 M (HC) NaCl or 1.1 M AmB for at least 1 h and briefly rinsed with deionized water (Synergy Ultra Water System; EMD Millipore) before installation in the reactor. Both electrodes were platinum mesh. The chambers had inlets and outlets for electrolyte recirculation, an opening on the top for an overhead stirrer (Petite Digital, 50-2500 RPM; Caframo, Ltd.) that was operated at 800 RPM, and custom PTFE fittings used for insertion of Ag/AgCl reference electrodes (RE-4B; Bioanalytical Systems, Inc.) on either side of the membrane. The platinum mesh and reference electrodes were connected to a potentiostat (Multi Potentiostat VMP3, Bio-Logic, ±0.5 mV) for electrical measurements.
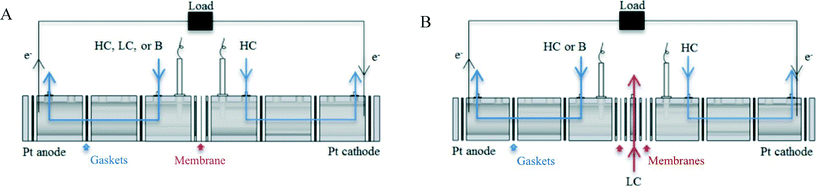 |
| Fig. 1 (A) The 2C reactor built with 6 cube sections, and (B) the 3C reactor containing an additional center chamber formed by two ion exchange membranes. The reference electrodes were placed as close as possible (1.138 cm) to the membrane to minimize the contribution of the solution to the measured resistance. The thin membranes are identified by small red arrows, while examples of gaskets are shown with small blue arrows. See text for the other abbreviations. | |
A three-chamber (3C) reactor was constructed to determine the impact of the additional chamber on nitrogen crossover and resistance. The center chamber was 0.54 cm wide and 3 cm in diameter (working area) (Fig. 1b). Two membranes were installed on either side of the center chamber, so that the anode and cathode chambers had the same volumes as the 2C reactor. The gaskets used to seal the center chamber were 0.158 cm each, producing a total center chamber length of 0.856 cm. The other components and operation of the 3C reactor were the same as those used for the 2C reactor. Variable amounts of ion exchange resin (0–3 g, as indicated) were added to the center chamber for conductivity measurements. For nitrogen crossover tests, 3 g of resin was used in the center chamber. Resin mass was measured based on dry weight using a balance (PB303-S, Mettler Toledo, ±0.5 mg). The resin was equilibrated in 500 mL HC NaCl or HC AmB and stirred for at least 8 h, rinsed with deionized water, and patted dry directly before use in the center chamber.
Experimental procedures
All membrane resistance, ion exchange resin conductivity, and nitrogen crossover measurements were performed in a temperature-controlled room (27.9 ± 0.6 °C). Solution conductivities (σ) were adjusted to 27.9 °C using the linear coefficient (2.1%) for salt solutions provided by Fisher Scientific.11 All reactors were equilibrated for 30 min under open circuit conditions before each test. The resulting voltage difference between the reference electrodes recorded for the rest of the test was used in resistance calculations.
Membrane resistance
Baseline resistances of the 2C and 3C reactors were measured weekly to ensure that the system resistance did not vary over time (Table S1†). HC solutions (1 M NaCl,
= 86.8 mS cm−1 at 25 °C) were pumped (single-pass) through the anode and cathode chambers at 3 mL min−1. In the 3C reactor, a HC NaCl solution flowed single-pass upward through the center chamber at 2 mL min−1. A potentiostat was used to set the current between platinum mesh electrodes at increments of 0.5 mA between 0 to 4 mA every 10 s.
Membrane resistances were measured using the 3C reactor with dissimilar (HC and LC) electrolytes for comparison with previous measurements.12,13 Two membranes of the same type (AEM or CEM) were installed in the reactor. The same solution conditions in previous tests were used except LC solution (10 mM NaCl,
= 1.07 mS cm−1 at 25 °C) flowed in a single-pass upward through the center chamber at 2 mL min−1 instead of HC solution. Current was increased from 0 mA to 4 mA in 0.5 mA increments every 10 s for AEM measurements. For CEM measurements, current data needed to be obtained more frequently (every 2 s) in order to measure intrinsic membrane resistance rather than boundary layer resistances which developed more slowly over time.
Ion exchange resin conductivity
The conductivities of four different high and low anion and cation ion exchange capacity (IEC) resins (100–200 mesh) were measured in the center chamber of the 3C reactor (Table S2†). The anionic (A) high IEC (H) resin (AH, Dowex 1X8) was tested in the 3C reactor between two AEMs (Table S3†) and the cationic (C) high IEC resin (CH, Dowex 50WX8) was tested between two CEMs using 1, 2 or 3 g of resin. These amounts of resin allowed examination on the effect of resin mass on transport, without completely filling the chamber (up to 50% of the center chamber volume), which would have increased pressure losses. The anionic low IEC (L) resin (AL, Dowex 1X2) was added between two AEMs, and the cationic low resin (CL, IEC Dowex 50WX2) was tested using CEMs, using 3 g of resin. A mixed system containing 50% AH and 50% CH resins was tested between one AEM and one CEM using 1, 2 or 3 g of resin for each test. HC NaCl solutions were pumped (single-pass) through the electrolyte chambers at 3 mL min−1, and LC solution was pumped through the center chamber at 2 mL min−1. The current was varied from 0 mA to 4 mA, in 0.5 mA steps every 2 s, using a potentiostat.
Low concentration chamber flow rate
The impact of center chamber flowrate was examined over the range of 0.5–30 mL min−1, corresponding to hydraulic retention times (HRTs) of 12 s–12 min (empty chamber volume of 6.2 mL). The anode and cathode chambers were operated with HC (1 M NaCl) solutions running single-pass at 15 mL min−1, and stirred at 800 RPM. LC solution (10 mM NaCl) was flowed in a single-pass upward through the center chamber. The potentiostat was operated at a stepped current between the platinum electrodes at 0.01, 0.1, 0.5, 1, 3, 7, and 10 mA at 2 s intervals to test the expected current range of the Selemion AMV during operation.14 Additional tests on flow were conducted with AH resin (3 g) added to the center chamber (50% void space). The solution and potentiostat conditions were the same as tests using the empty chamber, but the flowrate was varied between 0.25–15 mL min−1 to produce the same HRTs due to the volume occupied by the resin. The LC solution conductivity was measured at the outlet in a second series of tests using flow rates of 2–8 mL min−1 to analyze the effect that a conductivity change had on the calculated membrane resistance.
Nitrogen crossover
Nitrogen crossover through a single AEM was measured in the 2C reactor with solution strengths matching the anode chamber and adjacent HC RED cell of an MREC. The anode chamber contained a buffer (B) of either 50 mM NaHCO3 (pH = 8.5)5 or 50 mM NaHCO3–Na2CO3 (pH = 10.7). The cathode chamber contained HC solution (1.1 M NH4HCO3,
= 80 mS cm−1). Both solutions were recycled at 15 mL min−1 using 1 L storage reservoirs. The initial solution conductivities and anolyte pH were measured at room temperature. Current (4 mA) was set between the platinum electrodes for 24 h to simulate a typical MREC batch cycle.2 Nitrogen concentrations in the buffer were measured using standard methods [HACH Method 10031, High Range Test 'N Tube for Ammonia Nitrogen, and Orion 951007 or HACH Ammonia-Nitrogen Standard solutions (1000 mg L−1 ± 5 mg L−1)]. Standard additions (sample spike) and standard checks were performed to ensure accuracy. The final anolyte pH was measured at the end of every nitrogen crossover test.
Nitrogen crossover was measured in the 3C reactor containing two AEMs to determine the impact of using the additional chamber containing the LC solution. Solution conductivity, anolyte pH, and TAN were measured as described for the 2C reactor. The anolyte was 50 mM NaHCO3 buffer, and the catholyte was HC AmB. Both solutions were recycled at 15 mL min−1 using 1 L reservoirs. LC AmB (11 mM,
= 1.21 mS cm−1) flowed upward at 4 mL min−1 through the center chamber and was recycled in a 0.5 L reservoir. When filled with 3 g of AH resin (50% chamber volume) to measure if there was additional reduction in nitrogen crossover, LC AmB was recycled at 2 mL min−1 to produce the same residence time used for the empty middle chamber. Current was set at 4 mA between the two platinum electrodes for 24 h.
Ammonium bicarbonate chamber resistance
2C and 3C reactors containing AEMs were used to determine the resistance added by the center chamber. The 2C reactor was operated using 50 mM NaHCO3 buffer (pH = 8.5) in the anode chamber, and HC AmB in the cathode chamber (B | HC). Both solutions flowed in single-pass at 3 mL min−1. The current was stepped between the platinum electrodes from 0 to 4 mA in 0.5 mA steps every 2 s. The 3C reactor tests were made using the same anolyte and catholyte conditions as the 2C reactor, and tested with the same electrochemical measurements. LC AmB was pumped through the center chamber at 4 mL min−1 without resin (B | LC | HC) or 2 mL min−1 with 3 g of AH resin in the center chamber (B | LC + R | HC).
Reactor resistance and resin conductivity calculations
The total measured resistance between the reference electrodes (Rmeas, Ω) for both the 2C and 3C reactors was calculated using Ohm's law as: |  | (1) |
where U is the voltage measured with a set current (I).
The volume of resin (Vresin, mL) present in the center chamber was calculated as:
| 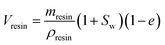 | (2) |
where
mresin is the mass (g) of resin added,
ρresin is the moist density of the resin beads reported by the manufacturer (0.730 g mL
−1, Dowex 1; 0.821 g mL
−1, Dowex 50 W),
Sw = 0.2 is the increase in resin volume assuming a conservative resin swelling of 20% from values reported by the manufacturer, and
e = 0.33 is the void space ratio reported by the manufacturer for this resin in low conductivity solution.
15,16 The volume fraction of resin used in the center chamber was calculated as:
|  | (3) |
where
VC is the empty bed volume of the center chamber.
The solution resistances (Rsoln, Ω) in the presence or absence of resin is:
| 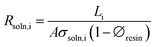 | (4) |
where
Li is the characteristic length scale of the measurement,
A is the cross-sectional area of the reactor (7.26 cm
2), and
σsoln,i is the solution conductivity (mS cm
−1), where i indicates the anode chamber (An), center chamber (C), or cathode chamber (Cat). For the 2C and 3C reactors, the anode and cathode chambers had equal lengths where
LAn =
LCat 1.138 cm. The center compartment in the 3C reactor included the thickness of the surrounding gaskets (
LC = 0.856 cm).
Rmeas (Ω) is the sum of the individual resistances, or
| Rmeas = Rsoln,An + nRmem + Rsoln,C + Rsoln,Cat | (5) |
where
n is the number of membranes (
n = 1 for the 2C system, and
n = 2 for the 3C system). Membrane area-resistance (
Rmem,A, Ω cm
2) was calculated as
Rmem,A =
RmemAG, where
AG = 6.55 cm
2 is the measured area of the gasket.
The resistance of the center compartment (RC, Ω) was used to determine the resin conductivity (σresin, mS cm−1). When ∅resin ≠ 0, the center compartment resistance is calculated from measured resistances as Rmeas = Rsoln,An + 2Rmem + RC + Rsoln,Cat. The resin (Rresin, Ω) and solution resistances in the center chamber acted like resistors in a parallel circuit, and therefore:
| 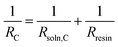 | (6) |
The resistance of the resin in the center chamber can be expressed as a function of the resin conductivity as:
| 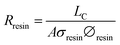 | (7) |
Rearranging eqn (6), and using eqn (7) and (4), the conductivity of the resin can be calculated as:
| 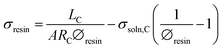 | (8) |
The average resin conductivity (
resin) was calculated from individual measurements using different volume fractions of resin (∅resin). The resistance of the center chamber, on average (RC,fit, Ω), can therefore be predicted using
resin and eqn (5)–(7), as:
| 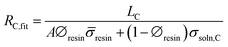 | (9) |
In experiments using mixed-resin, RC,fit was calculated using the average of the AH and CH resin conductivities (
resin,AH and
resin,CH).
Results
Membrane resistance in NaCl solutions
The resistances of the membranes were first examined using NaCl to demonstrate accuracy of our measurements through comparisons of membrane resistances obtained here with previous reports using slightly different methodologies. The resistance of the AEM in the 3C reactor calculated to be 64 ± 13 Ω cm2, which was slightly larger than that reported by Geise et al.13 (49 ± 20 Ω cm2) using the same membrane and LC and HC NaCl concentrations used here. However, they measured the resistance of the membrane and solution without flow past the membrane. The CEM had a resistance of 52 ± 15 Ω cm2, which was lower than that reported by Geise et al.13 (76 ± 21 Ω cm2) under lower experimental temperatures, but higher than the 42 Ω cm2 measured by Galama et al.12 using 0.9 M (HC) and 10 mM (LC) NaCl solutions on either side of one membrane, compared to 1 M and 10 mM NaCl solutions used here. These results validate the accuracy of this new experimental setup for measuring membrane resistance.
Resin conductivity and center chamber resistance
AH resin had the highest conductivity of all resins tested (11.7 ± 2.9 mS cm−1), with lower conductivities in the order: CL resin, 9.2 ± 0.5 mS cm−1; AL resin, 8.8 ± 0.5 mS cm−1; and CH resin, 7.0 ± 1.0 mS cm−1. The low conductivity of the CH resin was unexpected given its high IEC, but could be due to the high degree of crosslinking.17
There was an 82% reduction in the center chamber resistance, (RC = 97.2 ± 0.0 to 17.6 ± 0.1 Ω) using 3 g of AH resin (∅resin = 0.5) (Fig. 2). There was relatively good agreement between the resistances measured for this resin at different volume fractions with those predicted using the average resin conductivity (
resin = 11.7 mS cm−1; eqn (9)) (Fig. 2). It is predicted using eqn (9) that there would be little further reduction in the center chamber resistance by filling the center chamber with more than ~50% of the volume with resin, and thus there was no practical reason to increase the resin volume fraction further. The volume fraction of resin in the chamber must be balanced against the pumping losses associated with solids in the flow chamber. The AH resin (3 g) was used in further tests because it had the highest tested performance of the resins examined here.
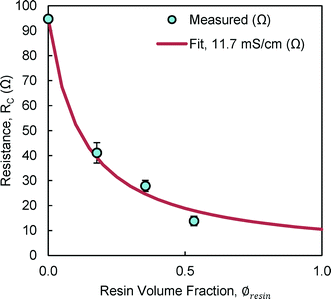 |
| Fig. 2 The center compartment resistance (RC) using NaCl solutions for different AH resin volume fractions (∅resin) to that predicted using an average resin conductivity of 11.7 mS cm−1 (RC,fit). | |
The center chamber resistance when filled with resin was not well predicted based on the average conductivities of the AH and CH resins (
resin,avg = 9.4 mS cm−1) (Fig. 3). The difference between calculated and measured data for the case with no resin (∅resin = 0) was due to small temperature changes in the controlled temperature room. The calculated fit of the mixed resin results indicated that the mixed resin had on average a conductivity of
resin = 3.1 ± 1.0 mS cm−1. This lower conductivity and high deviation suggested that the use of both the anionic and cationic resins between an AEM and CEM impeded charge transfer through the middle chamber, and could have had a bipolar effect at the membrane interface.
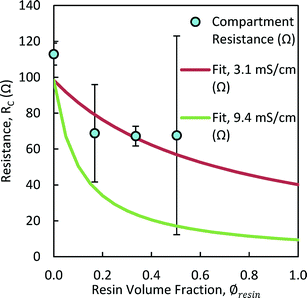 |
| Fig. 3 Area resistances using NaCl with mixed AH and CH resins (50 : 50) compared to that calculated (RC,fit) using the average conductivities of the two resins of resin,avg = 9.4 mS cm−1, and a best-fit regression line (RC,fit) obtained by using a resin conductivity of resin = 3.1 mS cm−1. | |
Impact of flow rates on center chamber resistance
In the absence of resin in the center chamber, the AEM resistance increased by 14% to 85 ± 8.7 Ω cm2, as the HRT was decreased from 3.1 min to 47 s (Fig. 4a). For the same range of HRTs using AH resin (∅resin = 0.5), AEM resistance increased by 10% to 78 ± 3.8 Ω cm2. At the highest flow rate (30 mL min−1) without resin, membrane resistance increased to 110 Ω cm2 (Fig. 4b). Data using resin could not be obtained at this higher flowrate as the resin could not be retained in the center chamber due to fluidization.
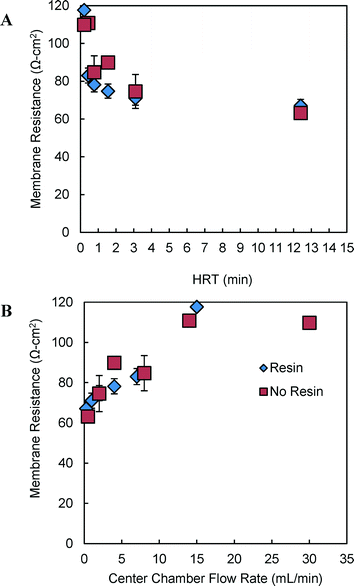 |
| Fig. 4 Membrane resistance in the LC chamber as a function of (A) HRT and (B) flowrate. | |
Solution conductivities increased only 1–3% at the center chamber outlet due to salt intrusion from the HC to the LC compartments, with and without resin, at flowrates of 2–8 mL min−1 in the center compartment. However, even these small changes in LC solution conductivities increased the measured membrane resistances up to 23%, based on the outlet conductivities of the LC solution, because a higher proportion of the total resistance was due to the membranes. Using the average conductivity of inlet and outlet solutions, membrane resistance was estimated to be increased by <12% (Fig. S1†).
Nitrogen crossover
Nitrogen crossover measured in the 3C reactor using 3 g (50% void volume) of AH resin (HC | LC + R | B) showed that the TAN concentration could be reduced by 96.5% to 26 ± 7 mg L−1 after 24 h, compared to the control (2 chamber reactor; HC | B) lacking the center chamber (741 ± 39 mg L−1) (Fig. 5). This TAN crossover was also 36% less than that obtained using a center chamber with no resin (HC | LC | B). These results therefore demonstrate that nitrogen crossover in an MREC could be greatly reduced by using an additional LC chamber (two AEMs) loaded with resin.
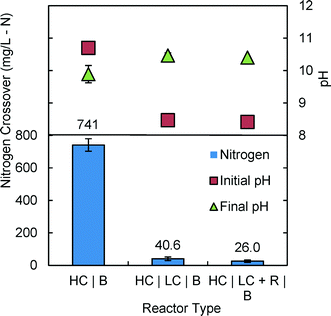 |
| Fig. 5 Nitrogen crossover based on TAN concentrations in the anolyte after 24 h, and anolyte initial and final pH. | |
The pH of the anolytes in the 3C tests increased from 8.5 ± 0.0 to 10.4 ± 0.0 with resin, and to 10.5 ± 0.1 without resin (Fig. 5). This increase in pH is different than that reported for MRECs where the pH decreased or remained neutral.2,18 In the control 2C reactor, the anode pH decreased from 10.7 to 9.9 ± 0.3. This pH decrease resulted from water electrolysis at the platinum electrode.
Low concentration chamber resistance with ammonium bicarbonate
The resistance due to membranes was 2.9 times higher in the 3C reactor (295 ± 37 Ω cm2) than in the 2C reactor (103 ± 18 Ω cm2) (Fig. 6). The Selemion AMV resistance in the 2C reactor was lower than the ~115 ± 20 Ω cm2 reported by Geise et al.,19 who used less conductive solutions. The resistance observed in this study was expected because membrane resistance decreases as resistance is measured using increasingly conductive solutions.
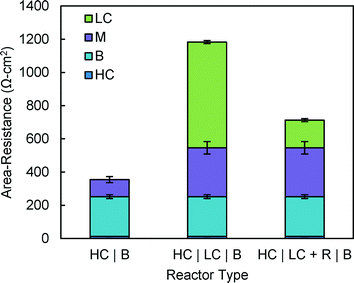 |
| Fig. 6 Area-resistances measured in 2C and 3C reactors using ammonium bicarbonate: R = resin, HC = high concentration solution, LC = low concentration solution, B = buffer solution, and M = membrane. | |
When 50% of the LC chamber volume was filled with AH resin (HC | LC + R | B), the LC chamber resistance (637 ± 35 Ω cm2) decreased by 74% to 166 ± 9 Ω cm2 (Fig. 5). The majority of the resistance (64%) came from the membranes, with a total resistance of 461 ± 38 Ω cm2 for membranes and solution. Because the membrane resistance was the same as controlled by the solution concentrations, the majority of the resistance (68%) in the absence of the resin (HC | LC | B) was due to the low conductivity solution. The resistance of the 2C reactor (HC | B) was lower than either of the 3C configurations because there was no resistance due to a LC solution or additional membrane.
Discussion
The use of a high IEC anion exchange resin (AH) reduced the LC chamber resistance using both NaCl and AmB LC salt solutions. The resistance was reduced by 82% (to 115 ± 1 Ω cm2) using NaCl, and only slightly less (by 74%, to 166 ± 9 Ω cm2) with AmB. The beneficial effect of resin addition was not further increased by filling more than 50% v/v of the chamber with resin (Fig. 2). Although the total internal resistance was still higher with the LC chamber and resin than a system without an additional chamber, the LC chamber was needed to reduce nitrogen crossover. The loss of power in an MREC by using an additional LC chamber near the anode could be overcome by adding additional membrane pairs to boost the stack voltage (see calculations in the ESI†).
The LC chamber resistance could be further reduced by decreasing the width of the LC chamber. The chamber used here (8.56 mm) was much wider than a typical RED stack cell20,21 or cells used in a previous MREC study (1.3 mm).5 For example, using eqn (4), we estimate that the LC AmB area-resistance could be reduced from 166 Ω cm2 to 25 Ω cm2 by using a 1.3 mm thick gasket. It is not known, however, how changing the width of the chamber might affect nitrogen crossover, although it is likely that crossover could increase.
A further reduction in the LC chamber resistance may be possible using anion exchange resins with a higher IEC. Three factors that can increase conductivity are: a higher concentration of fixed ionic groups; a lower degree of cross-linking; and a low valence and smaller size counter ions in the resin.17 The AH resin used here (IEC of 1.2 meq L−1) was 33% more conductive than the AL resin (IEC of 0.7 meq L−1) due to the high concentration of fixed anionic groups, despite the higher degree of cross-linking (Table S2†). Resins with higher IECs could therefore likely improve resin conductivity. One potential anionic resin with higher IEC is Dowex Marathon A (1.3 meq L−1, 30 mesh). It is larger than the resins tested here but it would still fit in the resign chamber (1.3 mm).22 The impact of resin size was not examined here.
Nitrogen crossover and pH changes
The use of an additional AEM to form the LC chamber (no resin) reduced nitrogen crossover by 94.5%. Adding the AH resin (50% v/v) reduced TAN concentrations in the anode chamber even further to 96.5% (from 741 mg L−1 to 26 mg L−1) (Table S4†). The improved reduction in nitrogen crossover obtained here was primarily due to the use of two adjacent AEMs, and to a smaller extent the addition of the resin. Although the reduction in nitrogen crossover by adding the resin is small, compared to just the use of the two AEMs, the resin was needed to avoid large increases in internal resistance and thus large power losses. Luo, et al.5 reduced nitrogen crossover by 60% (to 311 mg L−1) by placing a CEM in between the anode chamber and the AEM to form an additional LC chamber. The use of a CEM next to the anode chamber by Luo, et al.,5 however, likely facilitated crossover of NH4+ cations or uncharged soluble NH3, which are present at higher concentrations than carbamate anions. For example, at a pH of 7 and temperature of 28 °C, the concentration of NH4+ is greater than 5 times that of carbamate.23 Thus, while the inclusion of an additional LC chamber in the RED stack by Luo, et al. decreased nitrogen crossover, and did not adversely impact power generation,5 nitrogen crossover still remained relatively high due to the transfer of NH4+ ions through the CEM into the anode chamber.
The pH of the anolyte increased due to the additional LC chamber with two AEMs. In tests both with and without resin, anolyte pH increased from 8.5 to 10.4–10.5 after 24 h. This increase was likely due to carbamate transfer into the anolyte, and proton and HCO3− transfer out of the anolyte through the AEM.24 AEMs are not 100% permselective, and protons can be quite permeable in AEMs.25 A slightly alkaline anolyte can improve anode performance.26 However, a pH of ≥10 can impair current generation. The impact of the ion crossover on pH would therefore depend on the initial pH of the wastewater and its buffering capacity.
Membrane resistance and impacts on LC chamber flowrate
Ion exchange membrane resistance is significantly increased when the membranes are exposed to LC solutions compared to HC solutions.12,13 For example, the resistance of the Selemion AEM used here was 64 ± 13 Ω cm2 when the membrane was placed in between a HC solution (1 M NaCl) and LC solution (0.01 M NaCl), compared to 3.7 ± 0.1 Ω cm2 when the conductivity was measured using a membrane in contact with two HC solutions13 (1 M NaCl). When an ion exchange membrane is placed between solutions that have different conductivities, two different factors can increase membrane resistance: the low conductivity of the solution on the LC side; and mass transfer limitations due to the formation of a concentration boundary layer at the solution-membrane interface. The main impact on the measured resistance was due to the intrinsic change in the membrane resistance by the use of an LC solution when adding the additional chamber. The membrane resistance increased to 103 ± 18 Ω cm2 when the AEM was placed in between the buffer and HC AmB, likely due to the decreased mobility of the anions in AmB compared to NaCl.13,19 In the 3C reactor with the low conductivity buffer, and LC and HC AmB, membrane resistance further increased to 295 ± 37 Ω cm2 due to the use of the two low conductivity solutions (
= 4.3 mS cm−1, buffer, and
= 1.2 mS cm−1 AmB).
When concentration boundary layers contribute to internal resistance, they can be decreased by increasing the solution interfacial velocity at the membrane interface.14 However, the opposite effect was obtained here with fluid velocity, suggesting concentration boundary layer impacts on resistance were relatively small for our test conditions. When the flowrate was increased from 0.5 to 15 mL min−1, the membrane resistance increased from ~60 to ~110 Ω cm2 with or without resin in the LC chamber (Fig. 4). Further increase in the flowrate (no resin) did not impact resistance. The increase in membrane resistance with an increase in flowrate, rather than a decrease in resistance with flowrate, is consistent with lower effective salt crossover to the LC compartment with higher flowrates. Any salt crossover from the HC to the LC compartment will drastically change the measured LC and membrane resistances since the conductivity versus ion concentration curve is very steep at low concentrations. Membrane resistance was reduced at low flow rates (0.25 to 8 mL min−1, 47 s to 12.4 min HRTs) likely due to salt crossover into the LC solution from the HC solution, which would have decreased the membrane resistance by increasing the salt concentration on the LC side.13
The LC chamber fluid velocities were slightly greater in experiments with the resin, as the volume available for fluid flow through the chamber was reduced when the resin was added, and the solution flowrate into the chamber was held constant. This difference in fluid velocities likely did not significantly impact membrane resistance in nitrogen crossover tests as the membrane resistance at the same HRT (1.5 min) in these tests (76 ± 4 Ω cm2 with resin, compared to 68 ± 9.5 Ω cm2 without resin) was within the measured membrane standard deviation (64 ± 13 Ω cm2) (Fig. 4 and S1†).
Conclusions
These results show that nitrogen crossover can be reduced, but not completely eliminated by using an additional low concentration chamber with two adjacent AEMs, and an anion exchange resin with a high IEC. This work suggests that methods to recover the nitrogen from the wastewater stream, using for example efficient ammonia adsorption materials such as clinoptilolite,27,28 may be needed to enable the use of AmB in closed loop systems. The increase in internal resistance produced by using this chamber could be offset by using a few additional cell pairs in the RED stack. Overall, low cost ion exchange membranes and ammonia removal in downstream processes will be required to further develop MRECs based on thermally-regenerated AmB.
Acknowledgements
This research was supported by Award KUS-I1-003-13 from the King Abdullah University of Science and Technology (KAUST), and Department of Energy Cooperative Agreement DE-EE0005750.
References
-
B. E. Logan, Microbial fuel cells, John Wiley & Sons, Inc., Hoboken, NJ, 2008 Search PubMed.
- R. D. Cusick, Y. Kim and B. E. Logan, Science, 2012, 335, 1474–1477 CrossRef CAS PubMed.
- R. L. McGinnis, J. R. McCutcheon and M. Elimelech, J. Membr. Sci., 2007, 305, 13–19 CrossRef CAS PubMed.
- J.-Y. Nam, H.-W. Kim and H.-S. Shin, J. Power Sources, 2010, 195, 6428–6433 CrossRef CAS.
- X. Luo, J.-Y. Nam, F. Zhang, X. Zhang, P. Liang, X. Huang and B. E. Logan, Bioresour. Technol., 2013, 140, 399–405 CrossRef CAS PubMed.
- N. Shehab, G. Amy, B. E. Logan and P. E. Saikaly, J. Membr. Sci., 2014, 469, 364–370 CrossRef CAS PubMed.
- A. Morel, K. Zuo, X. Xia, J. Wei, X. Luo, P. Liang and X. Huang, Bioresour. Technol., 2012, 118, 43–48 CrossRef CAS PubMed.
- K. Zuo, L. Yuan, J. Wei, P. Liang and X. Huang, Bioresour. Technol., 2013, 146, 637–642 CrossRef CAS PubMed.
- K. Zuo, J. Cai, S. Liang, S. Wu, C. Zhang, P. Liang and X. Huang, Environ. Sci. Technol., 2014, 48, 9917–9924 CrossRef CAS PubMed.
- F. Zhang, M. Chen, Y. Zhang and R. J. Zeng, J. Membr. Sci., 2012, 417–418, 28–33 CrossRef CAS PubMed.
- Conductivity temperature compensation, In Thermo Fisher Scientific, http://thermoscientific.com/waterlibrary, 2011, p. 2.
- A. H. Galama, D. A. Vermaas, J. Veerman, M. Saakes, H. H. M. Rijnaarts, J. W. Post and K. Nijmeijer, J. Membr. Sci., 2014, 467, 279–291 CrossRef CAS PubMed.
- G. M. Geise, A. J. Curtis, M. C. Hatzell, M. A. Hickner and B. E. Logan, Environ. Sci. Technol. Lett., 2013, 1, 36–39 CrossRef.
- D. A. Vermaas, M. Saakes and K. Nijmeijer, J. Membr. Sci., 2014, 453, 312–319 CrossRef CAS PubMed.
- Dowex resins technical information bulletin, In Sigma-Aldrich, http://sigmaaldrich.com/chemistry/chemical-synthesis/learning-center/technical-bulletins, 2014.
- Dow ion exchange resins - Void volume, In Dow Answer Center, http://dowac.custhelp.com/app/answers, 2015.
-
F. G. Helfferich, Ion exchange, McGraw-Hill, 1962 Search PubMed.
- Y. Kim and B. E. Logan, Proc. Natl. Acad. Sci. U. S. A., 2011, 108, 16176–16181 CrossRef CAS PubMed.
- G. M. Geise, M. A. Hickner and B. E. Logan, ACS Macro Lett., 2013, 2, 814–817 CrossRef CAS.
- D. A. Vermaas, E. Guler, M. Saakes and K. Nijmeijer, Energy Procedia, 2012, 20, 170–184 CrossRef CAS PubMed.
- D. A. Vermaas, M. Saakes and K. Nijmeijer, Environ. Sci. Technol., 2011, 45, 7089–7095 CrossRef CAS PubMed.
- Industrial water resins, In Dow Water & Process Solutions, http://dowwaterandprocess.com/en/products/ion_exchange_resins/industrial_water_resins, 2013.
- N. Wen and M. H. Brooker, J. Phys. Chem., 1995, 99, 359–368 CrossRef CAS.
- F. Mani, M. Peruzzini and P. Stoppioni, Green Chem., 2006, 8, 995–1000 RSC.
- H. Strathmann, A. Grabowski and G. Eigenberger, Ind. Eng. Chem. Res., 2013, 52, 10364–10379 CrossRef CAS.
- S. Puig, M. Serra, M. Coma, M. Cabre, M. D. Balaguer and J. Colprim, Bioresour. Technol., 2010, 101, 9594–9599 CrossRef CAS PubMed.
- D. Karadag, Y. Koc, M. Turan and B. Armagan, J. Hazard. Mater., 2006, 136, 604–609 CrossRef CAS.
- X. Li, C. Lin, Y. Wang, M. Zhao and Y. Hou, Procedia Environ. Sci., 2010, 2, 1598–1612 CrossRef PubMed.
Footnote |
† Electronic supplementary information (ESI) available: One figure and four tables are provided. See DOI: 10.1039/c5ew00160a |
|
This journal is © The Royal Society of Chemistry 2015 |