DOI:
10.1039/C4EN00145A
(Perspective)
Environ. Sci.: Nano, 2015,
2, 114-119
Nanotechnology: nature's gift or scientists' brainchild?
Received
4th September 2014
, Accepted 2nd December 2014
First published on 2nd December 2014
Abstract
In the field of environmental nanotechnology, opinions on the novelty of engineered nanomaterials vary; some scientists believe that many engineered nanomaterials are indeed unique, while others are convinced that we are simply fabricating structures already designed in nature. In this article, we present balanced, objective evidence on both sides of the debate. While the idea of novel nanomaterials opens the mind to imagine truly unique structures with architectures unparalleled in nature, the idea that these structures have related analogs in nature has environmental relevance as scientists and engineers aim to design and manufacture more sustainable and environmentally benign nanomaterials.
Nano impact
The environmental nanotechnology community is growing rapidly and involves researchers in a wide array of disciplines. As the field grows, certain questions remain unanswered and leave room for new discoveries. One question is fundamental to the field: is nano new or not? This topic was debated at a recent conference and succeeded in provoking attendees to think more about the novelty of nanomaterials. We organized this paper in a similar manner as the debate. The introduction explains the importance of debating the novelty of nanomaterials; Michael Hochella and Michael Spencer then share their views of the topic. We provided a balanced, objective treatment of both sides of the debate in order to allow the reader to form his/her own conclusion.
|
A. Introduction
The discovery and application of nanomaterials and nanoscale devices have allowed for far-reaching developments in fields as diverse as nanomedicine, sensing, electronics, materials science, and environmental remediation.1–4 The rate and scale of these discoveries has increased astronomically over the last several decades spurred on in the 1980's by the development of revolutionary new materials (e.g. fullerenes) and instruments (e.g. scanning tunneling and atomic force microscopes).5,6 Since that time, nanoscale architectures have led to the development of new structures possessing unique chemical and physical properties and incredible potential and versatility.
On the other hand, with the proliferation of engineered nanomaterials comes uncertainty regarding the effect of these materials on the environment and on public health.7–10 When framing questions around the development and application of nanomaterials, it is important to consider whether contact with these engineered nanomaterials (ENMs) represents a new type of exposure, or whether organisms have been exposed to nanomaterials for millions (even billions) of years. If there exist naturally occurring materials that are similar in structure and function to ENMs, there would be a reduced likelihood of adverse ecosystem and health effects, and scientists could use naturally occurring structure relationships to inform manufacturing of new materials.
Prior to possibly accepting this proposition that ENMs are simply mimics of nature's handwork, we must first give the issue reasonable debate and thought. At a recent conference organized around the topic of environmental nanotechnology, the conference attendees participated in a spirited debate around this topic. The proposition statement, “The magic of nanomaterials is not new: nature has been playing these tricks for billions of years” was debated by two leading investigators: Michael Hochella, a geoscientist, debated the affirmative statement, while Michael Spencer, an electrical engineer/materials scientist, debated the negative statement. What follows is their treatment of the proposition statement.
B. Natural origins of nanomaterials
An atom switch is one of the latest and most remarkable achievements in nanoscience and engineering,11,12 but you won't see it imitated or even suggested by nature in a soil profile, or will you? Nano-materials scientists and engineers have been incredibly resourceful in creating “new” and novel nanomaterials and devices, all of which play roles in driving the nanotechnology revolution that is still in a fast moving form of adolescence in the early 21st century.13 However, and clearly taking nothing away for this truly remarkable and revolutionary materials science, are all of these advances really new, or have they been produced with or without our realization, at least in principle, by nature? For example, the 1996 Nobel Prize in Chemistry was awarded to Robert F. Curl Jr., Sir Harold Kroto, and Richard E. Smalley for the discovery of fullerenes in 1985. Since then, naturally-occurring and “incidental” fullerenes have been found in everything from soot14 to deep space.15,16 It is arguable that fullerenes are present in unimaginable quantities, in every conceivable configuration, throughout the universe.16 And there is a lot of room in our universe (currently measured at 1024 km across) to do it with the full compliment of the periodic table spread throughout. Temperatures and pressures just within our own solar system (not including our sun) range from 3 to 7000 K and from 10−7 to 106 atmospheres pressure. And in the Milky Way alone, there are over 100 billion stars, and roughly that many planets, including a remarkable number of Earth-sized planets orbiting Sun-like stars.17 Yet our galaxy is only one of more than 100 billion galaxies, meaning the number of stars, planets, comets, asteroids, etc. truly defy comprehension. Back here at our infinitesimally small corner of the universe, just on and near Earth's surface alone, it has been estimated that natural biogeochemical processes produce many thousands of terragrams (1 Tg = 1 million metric tons) of inorganic, organic, and “mixed” nanomaterials per year in a much wider variety than we can possibly presently know (Fig. 1).18 And the naturally-occurring nanomaterials that we have observed to date exhibit an astounding range of variety and complexity.19 In contrast, the current estimates of the annual manmade production of high-tonnage nanomaterials (nano-TiO2; nano-CeO2; carbon nanotubes; fullerenes; nano-Ag) are in the ballpark of hundredth to thousandth of Tg per year,20,21 roughly five to six orders of magnitude less than nature's bounty, and by comparison, limited in compositional and structural variation.
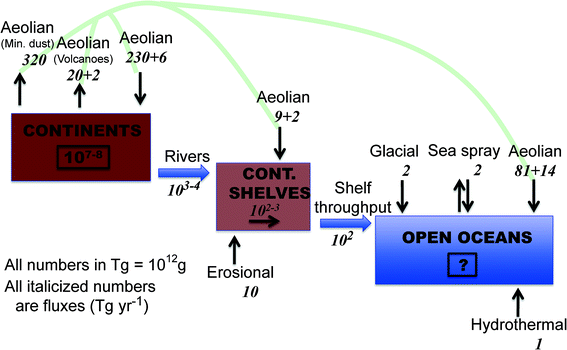 |
| Fig. 1 The global budget for naturally occurring inorganic nanoparticles. All numbers are in units of terragrams (Tg = 1012 g). All italicized numbers are fluxes (Tg per year), and the numbers in rectangular boxes are reservoir sizes, if known. Some of the nanomaterial fluxes are listed as two components, explained as follows: for the volcanic input to the atmosphere, 20 Tg is due to SO2 aerosol formation, and 2 Tg is due to mineral ash. For the three aeolian inputs to the continents, continental shelves, and the open oceans, the first number is due to the 320 Tg continental mineral dust output, and the second number is due to the 22 Tg volcanic output. From Hochella et al., 2012. | |
C. Evidence of natural nanostructures
All matter in the universe, except most of the H and the noble gases, has at some time existed in a one-, two-, or three-dimensional nanomaterial.16 Excluding hydrogen and the trace amounts of noble gases within you, this includes every atom in your body. To depict the starting point of this type of scenario, Fig. 2 (left) shows a NASA/Hubble Space Telescope image of a portion of the Eagle Nebula, only 6500 light-years from Earth, which are pillars of gas and dust many light-years in length. Here, stars are born with an accompanying disk-like cloud of dust and gas (mostly hydrogen) that will eventually form solar systems.22 This dust contains a vast assortment of oxide, silicate, carbide, nitride, carbonaceous, and organic nanomaterials as determined by astronomical observations (particularly infrared spectroscopy), as well as the direct analysis of “stardust” collected during space missions and isolated from meteorites.22 Diamonds found in the Murchison meteorite, measuring only a few nanometers in diameter (Fig. 2 – right), are particularly stunning examples of the nanoparticulate origin of everything associated with planetary system objects excluding stars themselves.23 These nanomaterials of all types are mixed, sorted and modified throughout the universe. Energy is provided by the full range of electromagnetic radiation, as well as dramatic temperature and pressure gradients, shock waves, and physical collisions.22 This is presumed to provide the widest range of isomerization and phase mixing/re-equilibration that can be imaged across the chemical spectrum, much of it on the nanoscale. These materials are cycled and re-cycled through astronomical bodies of many descriptions around and apart from stars, and eventually reinjected into the interstellar medium of galaxies, and sometimes between galaxies, typically in the later stages of stellar evolution and especially via supernova events. And besides happening throughout the entire universe, it also occurs over expansive time, in fact for 13.8 billion years since the Big Bang.
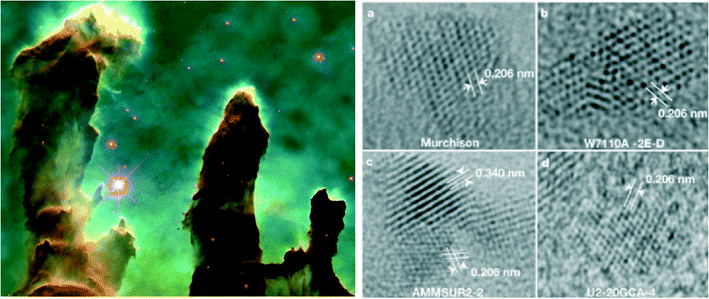 |
| Fig. 2 (Left) A portion of the Eagle Nebula. See text for details. NASA/Hubble Space Telescope image. (Right) Nanodiamonds (averaging 3 nm, as small at 1 nm with <150 carbon atoms) isolated from the Murchison meteorite. From Dai et al., 2002. | |
Back at home, our Earth is an important example of what can happen, among otherwise a dramatic array of possibilities, to nebular disk nanoparticles as they build first tiny clusters, and then eventually planetesimals, protoplanets, and ultimately planets over time.22 Now, on Earth during its 4.54 billion year life, nanomaterials are formed and destroyed continuously by planetary processes. This period of generation and destruction continually evolves with time. For example, Earth's early surface and atmosphere were markedly different from what we observe it to be today.24,25 Yet our young Earth would have still been conducive to the continuous formation of new nanomaterials, analogous to, but different from the vast production of naturally-occurring organic, inorganic, and mixed nanomaterials today. And later on, life on this planet, likely originating more than 3.5 billion years ago,26 did so in the presence of these “environmental” nanomaterials. All life is still bathed by, functions because of, has within it, and evolves among the vast variety of natural bottom-up and top-down produced nanomaterials present (Fig. 3).27,28
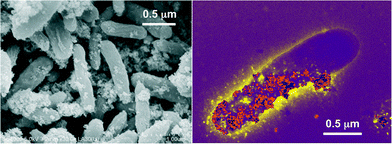 |
| Fig. 3 (Left) Geobacter sulfurreducens and Thiobacillus denitrificans accompanied by magnetite nanoparticles that act as interspecies electron transfer agents. This allows for microbial community cooperative functions, in this case promoting acetate oxidation coupled to nitrate reduction under anaerobic conditions. From Kato et al., 2012. (Right) This cell of Shewanella oneidensis MR-1, a facultative anaerobe capable of dissimilatory iron reduction, is respirating on 30 nm hematite particles. The rate of respiration depends on the nanoparticles' aggregation state, size, shape and exposed crystal faces. From Bose et al., 2009. | |
Until nanobiogeoscience advances and matures as a field, with so much more to survey in terms of nanomaterials in the vast oceans, the atmosphere, and on the continents, as well as in the deep Earth, we will not know the true extent of nanomaterial diversity even on this one celestial body.18 Eventually, we will have a much more complete understanding of nanomaterial function on this planet, and then there is always the rest of the universe to explore.
D. Novel nanoscale structures and materials
The proposition has been given to us that nano materials are not new but rather all of the novel and exciting properties of nanomaterials have been achieved in nature. To the layperson these astonishing proprieties can appear to be magical. So are we dealing with the magic of science or the magic of nature? We focus our discussion on engineers and applied scientists. In viewing the role of engineers we can see how man and nature can interact together in a harmonious way to produce a powerful result. This idea can be clearly demonstrated by examining a natural meadow (Fig. 4a) and a garden (Fig. 4b). The meadow is the subject of the scientist who seeks to find out the general physical laws, which underpin the structure and function of the meadow. The engineer can be closely identified with the artist, who in the garden weaves the natural element found in the meadow with powerful effect creating something, which is an amalgamation of nature and man.29 Florman30 summarizes this close relationship between the artist and engineer “But of course we rely upon the artist! He is our cousin, our fellow creator”. Man made nanomaterials distinguish themselves from natural materials through several properties. These properties include order, purity, and scale. These are properties that natural materials often do not have. It is clear that the ability of engineers to fabricate and control nanomaterials is not rivalled by nature. Over the past few decades we have seen science of nanotechnologies evolve in ways that even amaze the practitioners. And as Arthur Clarke,31 noted British science fiction writer, science writer, futurist and inventor, pointed out; “Any significantly advanced technology is indistinguishable from magic…”
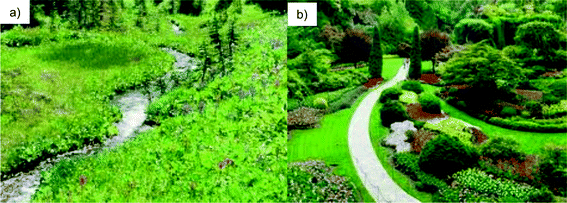 |
| Fig. 4 a) Untended meadow b) English style garden. Stock photos. | |
E. Evidence of novel nanostructures
Nowhere is the ability of man to overcome the boundaries of nature more in evidence than in silicon nanotechnology. In the application of silicon technology to integrated circuits we have seen a doubling of size, speed and functionality every two years32 (dubbed Moore's Law after Gordon Moore, founder and CEO of Intel Corp.). Silicon materials are celebrated for their perfection and application into integrated circuits (Fig. 5a); this is in contrast to natural crystals or gemstones (Fig. 5b) that are often revered for their imperfections. Today's silicon is totally free of dislocations (the name given to microscopic displacements of lines of atoms in a crystal).33 In addition to the mechanical perfection, the silicon used in the semiconductor industry has almost a complete absence of un-intentional impurities in the crystal.33 Most of these unwanted impurities are in concentrations less that 100 parts per million (ppm) while there are many unwanted impurities that are present in concentrations that are literally beyond our ability to measure. As a result of unprecedented levels of concentrated research and development over the last sixty to seventy years we have a materials fabrication technology that has exquisite control over every facet of the crystal growth environment and the crystal growth process. There is no analog of this type of material's foundry in nature, no place on the earth where it is possible to go with a pick axe and slice off a bit on material on which to produce a microprocessor. We can say without exaggeration that this materials technology has totally transformed the world and is unparalleled in nature. The electron microscope micrograph of Fig. 6a illustrates another type of precision possible with man's current mastery of nanotechnology. Fig. 6a depicts one monolayer (single atom layer) of freestanding graphene produced by under-etching the silicon carbide layer onto which the graphene was grown.
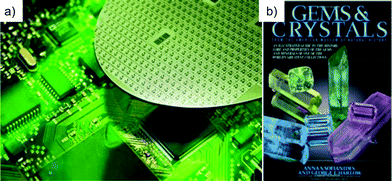 |
| Fig. 5 a) Silicon wafer with fabricated integrated circuits superimposed on electronic printed circuit board, from http://meroli.web.cern.ch/meroli/Lecture_silicon_floatzone_czochralski.html. b) A selection of precious gemstones, from Harlow and Sofianides, 1991. | |
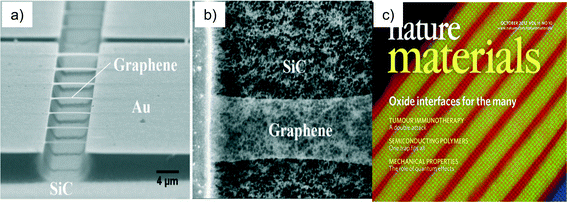 |
| Fig. 6 a) Electron micrograph of a freestanding graphene strip. The “epitaxial” graphene was produced by heating a silicon carbide (SiC) substrate at temperatures of 1700 °C in an argon environment. Suspended monolayer graphene strips approximately 5 μm long and .5 μm wide were produced by under etching the supporting SiC substrate, b) expanded view of one of the strips in a); the ghost image illustrates the monolayer nature of the graphene strip, c) large field of view, atomic resolution Electron Energy Loss Spectroscopy (EELS) elemental map from an n = 3 (LaMnO3)2n/(SrMNO3)n/SrTiO3 film measured by Angularly Resolved Photoemission spectroscopy (ARPES) showing La in green, Mn in red and Ti in blue, from Monkman et al., 2012. | |
Shown are thin strips of graphene one monolayer thick, which span the region between the silicon carbide supports. Fig. 6b is a close-up of one of the strips. In the close-up view, the strip appears as a ghost image; this is because the graphene is only one atom layer thick and as a consequence does not backscatter many of the electrons that ultimately provide the signal for the image.34 The ability to synthesize, manipulate and characterize films, which are literally one atomic layer thick, is an astonishing feat, currently being repeated daily in laboratories around the world. Fig. 6c35 illustrates a synthetic oxide grown layer-by-layer using molecular beam epitaxy (MBE). Molecular beam epitaxy is a material synthesis technology that allows the engineer to produce atomically controlled layers of material.36 In the MBE approach multiple heated ovens are used to evaporate material onto a heated surface. The multi-color image presented is a false color map of the atomic structure. Each individual ball represented in the image is a single atom. The materials designer and the MBE operator determine the number and sequence of the layers in the material structure. And just like we can write many pieces of literature revolving around the same general theme, we can develop an infinite number of combinations of the layers shown in Fig. 6c with each material flowing from the mind of some materials engineer.
The examples of nanotechnology shown in this section represent only a small sampling of the magic of nanotechnology, which has transformed and will continue to transform the world.
F. Summary and implications
Naturally occurring nanomaterials are ubiquitous through the universe. The variety of nanomaterials and distribution throughout the Earth's terrestrial and extraterrestrial systems indicates that life evolved in the presence of inorganic nanomaterials, and is in fact made in part of organic nanomaterials. The discovery of nanoscale structures in the lab has led to a proliferation of novel new structures and applications of nanomaterials tailored to specific applications. These application-specific structures rely on levels of purity, order and scale not yet identified in natural systems. When determining whether ENMs are truly novel or not, one must realize that we have only just begun to interrogate the Earth's surface and atmosphere for evidence of these structures, and newly identified, naturally occurring structures are being discovered everyday. At the same time, creative engineers are pushing the limits of discovery to design nanostructures with novel shapes, configurations and properties. At some point, the discovery of naturally-occurring nanomaterials may converge with new ENMs, but in the meantime, scientists and engineers must work together to increase the speed of discovery on both sides of the debate. As we continue to develop nanomaterials for applications, it is important to be aware of natural analogues in order to predict potential environmental and health impacts as well as inform the design and manufacture of nanomaterials with lower likelihood of environmental risks.
References
- Z. Fan, J. C. Ho, Z. A. Jacobson, H. Razavi and A. Javey, Proc. Natl. Acad. Sci. U. S. A., 2008, 105, 11066–11070 CrossRef CAS PubMed.
- E. Lavik and H. von Recum, ACS Nano, 2011, 5, 3419–3424 CrossRef CAS PubMed.
- M. S. Mauter and M. Elimelech, Environ. Sci. Technol., 2008, 42, 5843–5859 CrossRef CAS.
- O. V. Yazyev and Y. P. Chen, Nat. Nanotechnol., 2014, 9, 755–767 CrossRef CAS PubMed.
- R. M. Feenstra, J. A. Stroscio and A. P. Fein, Surf. Sci., 1987, 181, 295–306 CrossRef CAS.
- H. W. Kroto, J. R. Heath, S. C. O'Brien, R. F. Curl and R. E. Smalley, Nature, 1985, 318, 162–163 CrossRef CAS.
- C. E. H. Beaudrie, M. Kandlikar and T. Satterfield, Environ. Sci. Technol., 2013, 47, 5524–5534 CrossRef CAS PubMed.
- R. Fears, P. Gehr and E. Anklam, Nature, 2012, 488, 281–281 CrossRef CAS PubMed.
- M. P. Holsapple, W. H. Farland, T. D. Landry, N. A. Monteiro-Riviere, J. M. Carter, N. J. Walker and K. V. Thomas, Toxicol. Sci., 2005, 88, 12–17 CrossRef CAS PubMed.
- A. Nel, Y. Zhao and L. Mädler, Acc. Chem. Res., 2013, 46, 605–606 CrossRef CAS PubMed.
- C. Schirm, M. Matt, F. Pauly, J. C. Cuevas, P. Nielaba and E. Scheer, Nat. Nanotechnol., 2013, 8, 645–648 CrossRef CAS PubMed.
- S. J. van der Molen, Nat. Nanotechnol., 2013, 8, 622–623 CrossRef CAS PubMed.
-
Nanotechnology Research Directions for Societal Needs in 2020: Retrospective and Outlook, ed. M. Roco, C. A. Mirkin and M. C. Hersam, Springer, 2011 Search PubMed.
- W. Kratschmer, L. D. Lamb, K. Fostiropoulos and D. R. Huffman, Nature, 1990, 347, 354–358 CrossRef.
- P. Ehrenfreund and B. H. Foing, Science, 2010, 329, 1159–1160 CrossRef CAS PubMed.
-
A. Tielens, in Nature's Nanostructures, ed. A. S. Barnard and H. Guo, Pan Stanford Publishing Pte. Ltd., Singapore, 2012, ch. 14, pp. 361–384 Search PubMed.
- E. A. Petigura, A. W. Howard and G. W. Marcy, Proc. Natl. Acad. Sci. U. S. A., 2013, 110, 19273–19278 CrossRef CAS PubMed.
-
M. F. Hochella Jr, D. Aruguete, B. Kim and A. S. Madden, in Nature's Nanostructures, ed. A. S. Barnard and H. Guo, Pan Stanford Publishing Pte. Ltd., Singapore, 2012, pp. 1–42 Search PubMed.
- M. F. Hochella, S. K. Lower, P. A. Maurice, R. L. Penn, N. Sahai, D. L. Sparks and B. S. Twining, Science, 2008, 319, 1631–1635 CrossRef CAS PubMed.
- C. O. Hendren, X. Mesnard, J. Dröge and M. R. Wiesner, Environ. Sci. Technol., 2011, 45, 2562–2569 CrossRef CAS PubMed.
- N. C. Mueller and B. Nowack, Environ. Sci. Technol., 2008, 42, 4447–4453 CrossRef CAS.
-
F. J. Rietmeijer and J. A. Nuth, in Nature's Nanostructures, ed. A. S. Barnard and H. Guo, Pan Stanford Publishing Pte. Ltd., Singapore, 2012, ch. 13, pp. 329–360 Search PubMed.
- Z. R. Dai, J. P. Bradley, D. J. Joswiak, D. E. Brownlee, H. G. M. Hill and M. J. Genge, Nature, 2002, 418, 157–159 CrossRef CAS PubMed.
- J. W. Valley, A. J. Cavosie, T. Ushikubo, D. A. Reinhard, D. F. Lawrence, D. J. Larson, P. H. Clifton, T. F. Kelly, S. A. Wilde, D. E. Moser and M. J. Spicuzza, Nat. Geosci., 2014, 7, 219–223 CrossRef CAS.
- K. Zahnle, L. Schaefer and B. Fegley, Cold Spring Harbor Perspect. Biol., 2010, 2(10), a004895 CAS.
- N. T. Arndt and E. G. Nisbet, Annu. Rev. Earth Planet. Sci., 2012, 40, 521–549 CrossRef CAS.
- S. Bose, M. F. Hochella Jr, Y. A. Gorby, D. W. Kennedy, D. E. McCready, A. S. Madden and B. H. Lower, Geochim. Cosmochim. Acta, 2009, 73, 962–976 CrossRef CAS PubMed.
- S. Kato, K. Hashimoto and K. Watanabe, Proc. Natl. Acad. Sci. U. S. A., 2012, 109, 10042–10046 CrossRef CAS PubMed.
-
W. Wulff, http://www.michaelschultheis.com/index.php?m=3.
-
S. C. Florman, The Existential Pleasures of Engineering, St. Martin's Griffin, New York, 2nd edn, 1976 Search PubMed.
-
A. C. Clarke, in Profiles of the Future: An Inquiry into the Limits of the Possible, Phoenix (an Imprint of The Orion Publishing Group Ltd), 1962, p. 256 Search PubMed.
-
G. E. Moore, Solid-State Circuits Society Newsletter, IEEE, 2006, vol. 11, pp. 33–35 Search PubMed.
-
J. D. Plummer, M. Deal and P. D. Griffin, Silicon VLSI Technology: Fundamentals, Practice, and Modeling, Prentice Hall, New Jersey, 1st edn, 2000 Search PubMed.
- A. K. Geim, Science, 2009, 324, 1530–1534 CrossRef CAS PubMed.
- E. J. Monkman, C. Adamo, J. A. Mundy, D. E. Shai, J. W. Harter, D. Shen, B. Burganov, D. A. Muller, D. G. Schlom and K. M. Shen, Nat. Mater., 2012, 11, 855–859 CrossRef CAS PubMed.
-
A. Y. Cho and J. R. Arthur, Progress in Solid State Chemistry, Pergamon, New York, 1975, vol. 10, part 3, pp. 157–191 Search PubMed.
|
This journal is © The Royal Society of Chemistry 2015 |