Pathways for degradation of plastic polymers floating in the marine environment
Received
30th April 2015
, Accepted 3rd July 2015
First published on 9th July 2015
Abstract
Each year vast amounts of plastic are produced worldwide. When released to the environment, plastics accumulate, and plastic debris in the world's oceans is of particular environmental concern. More than 60% of all floating debris in the oceans is plastic and amounts are increasing each year. Plastic polymers in the marine environment are exposed to sunlight, oxidants and physical stress, and over time they weather and degrade. The degradation processes and products must be understood to detect and evaluate potential environmental hazards. Some attention has been drawn to additives and persistent organic pollutants that sorb to the plastic surface, but so far the chemicals generated by degradation of the plastic polymers themselves have not been well studied from an environmental perspective. In this paper we review available information about the degradation pathways and chemicals that are formed by degradation of the six plastic types that are most widely used in Europe. We extrapolate that information to likely pathways and possible degradation products under environmental conditions found on the oceans' surface. The potential degradation pathways and products depend on the polymer type. UV-radiation and oxygen are the most important factors that initiate degradation of polymers with a carbon–carbon backbone, leading to chain scission. Smaller polymer fragments formed by chain scission are more susceptible to biodegradation and therefore abiotic degradation is expected to precede biodegradation. When heteroatoms are present in the main chain of a polymer, degradation proceeds by photo-oxidation, hydrolysis, and biodegradation. Degradation of plastic polymers can lead to low molecular weight polymer fragments, like monomers and oligomers, and formation of new end groups, especially carboxylic acids.
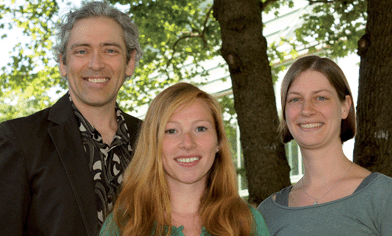
(From left to right) Matthew MacLeod, Berit Gewert and Merle M. Plassmann.
| Berit Gewert studied Environmental Sciences at the University of Greifswald, Germany. During her masters she focused on environmental and analytical chemistry. She did an internship and her master thesis at the Helmholtz Centre for Environmental Research in Leipzig, Germany. In February 2014 she started her PhD studies at the Department of Environmental Science and Analytical Chemistry at Stockholm University, Sweden and is focusing on chemical pollutants released to the marine environment by degradation of plastic debris. |
| Merle Plassmann studied Environmental Sciences at the University of Lueneburg, Germany. She received a PhD from Stockholm University, Sweden in 2011. After a three year postdoctoral fellowship at the Helmholtz Centre for Environmental Research in Leipzig, Germany, she went back to Stockholm University for a second postdoctoral project. Her field of studies include both targeted analysis of per- and polyfluorinated substances and non-target screening, focusing on LC- and GC-HRMS analysis. One of her current interest is the application of non-target screening methods to detect chemicals leaching from weathering plastics. |
| Matthew MacLeod is Associate Professor in the Department of Environmental Science and Analytical Chemistry at Stockholm University, Sweden. He holds a Bachelor of Science degree from the University of Victoria (British Columbia, Canada), and a Master of Science and PhD from Trent University (Ontario, Canada). He was formerly a post-doctoral fellow at the Lawrence Berkeley National Laboratory in Berkeley, California, USA, and Oberassistent at the Swiss Federal Institute of Technology (ETH) in Zürich Switzerland. His research program is focused on novel measurement and modeling techniques to study human and ecological exposure to environmental contaminants. |
Environmental Impact
The amount of plastic floating in the world's oceans is steadily increasing. Plastic in the marine environment is exposed to sunlight and oxidizing conditions that cause weathering of the polymers leading to degradation. The pathways for degradation of plastic polymers must be understood to detect and evaluate potential hazards. Many studies of polymer degradation reported in the literature describe experiments that were conducted under extreme conditions that are not environmentally relevant. We made extrapolations for degradation pathways of the six most widely used plastic polymers by considering relevant conditions found at the ocean's surface. This review thus summarizes relevant degradation pathways and presents possible degradation products that may be liberated from plastic as it ages in the marine environment.
|
Introduction
Plastic plays an important role in our everyday lives, it is essential for the economy and we find it in a large variety of consumer products because it is light, durable and cheap. Mass production of plastics began in the 1940s and since then the amounts of plastic produced annually increased rapidly.1,2 Global plastic production reached a level of 299 Mt per year in 2013.3 The most common plastics, which account for about 80% of European plastic demand, are polyethylene (PE), polypropylene (PP), poly(vinyl chloride) (PVC), polystyrene (PS), poly(ethylene terephthalate) (PET) and polyurethane (PU) (Fig. 1).4 Over one-third of plastic in both the United States and Europe is used in disposable products such as packaging, eating utensils and trash bags, which are designed to be discarded within three years of their production.3,5 Due to their stability and high durability, plastics have long degradation times and tend to accumulate in the environment.5 The rising amounts in the environment and especially in the marine environment are of increasing environmental concern.6 Ocean currents and wind transport plastic in the oceans, and it fragments as it ages.7,8 Thus plastic can be found worldwide in oceans in different size fractions.5 Meso- and macroplastic includes all plastic particles of a size fraction of more than 5 mm in diameter, while microplastic includes particles less than 5 mm in diameter.9
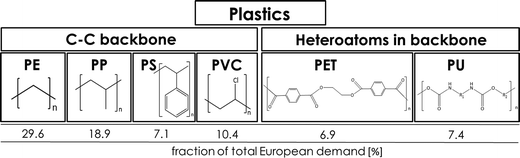 |
| Fig. 1 Polymer types with structures and their fraction of the total European demand (PE – polyethylene, PP – polypropylene, PS – polystyrene, PVC – poly(vinyl chloride), PET – poly(ethylene terephthalate), PU – polyurethane) (numbers from ref. 3). | |
A wide variety of chemical additives are incorporated into plastic polymers to impart particular properties, for example bisphenol A and phthalates.10,11 Some additives are designed to stabilize the polymer and make it more resistant towards degradation.10,12 The additives are usually not covalently bonded to the polymer and therefore they can leach out from the plastic as it degrades and enter the marine environment.13 Besides additives, attention has been drawn to persistent organic pollutants (POPs), such as PCBs, DDTs and PAHs, that may sorb to plastics in the environment. Plastic particles accumulate hydrophobic POPs, resulting in concentrations that can be several orders of magnitude higher than those in the surrounding sea water.14 Microplastic is more prone to sorb waterborne contaminants compared to meso- and macroplastic due to its higher surface area to volume ratio.9
Plastics are expected to persist in the environment for hundreds or even thousands of years.5 In a 1975 review article, Deanin wrote that polymers used in plastics are generally harmless.15 However, as plastic ages in the marine environment, it presents a potential chemical hazard that is not only due to the release of POPs from the plastic surface and chemical additives leaching out of the plastic, but also due to release of chemicals produced by degradation of the plastic polymer itself. In this article we review the scientific literature about pathways for degradation of plastic polymers and discuss their relevance to conditions encountered by plastic floating in the marine environment. We explicitly consider the six most important types of plastic in European commerce, and speculate about chemicals that might be liberated into the marine environment as plastic ages.
Polymer degradation
Much of the literature about degradation of plastic polymers has been authored by polymer engineers. From the perspective of a polymer engineer, the term “degradation” is most often used to describe processes that lead to a decline of polymer properties.16 Environmental chemists, however, are interested in the chemical reactions that cause the breakdown of polymers, and the properties and potential hazards associated with chemicals liberated by degradation of the polymers.
Degradation of plastic polymers can proceed by either abiotic or biotic pathways.17 Generally abiotic degradation precedes biodegradation, and is initiated thermally, hydrolytically, or by UV-light in the environment.14 Smaller polymer fragments formed by abiotic degradation can pass through cellular membranes and are biodegraded within the microbial cells by cellular enzymes, however some microbes also excrete extracellular enzymes that can act on certain plastic polymers.18 Most plastics degrade first at the polymer surface, which is exposed and available for chemical or enzymatic attack. Therefore degradation of microplastic proceeds faster than meso- and macroplastic, as microplastic has a higher surface to volume ratio. The first visual effects of polymer degradation are changes in color and crazing of the surface.19 Surface cracking makes the inside of the plastic material available for further degradation, which eventually leads to embrittlement and disintegration.19
Plastic materials in the environment are exposed to conditions that could promote weathering by any of the above processes. However, not all kinds of degradation pathways are effective on all polymer types. In the following sections we review the literature on degradation of each of the six most common polymer types separately to identify likely pathways for degradation of floating plastic. Many of the studies cited in this review report degradation experiments conducted under conditions that are not environmentally relevant. Therefore we have attempted some speculative extrapolations of the findings reported in literature to conditions encountered by plastic floating in the marine environment. Plastic floating on the ocean surface is exposed to moderate temperatures, solar radiation at wavelengths of 300 nm and longer, and oxidizing conditions. Since temperatures are moderate, the most important factors initiating abiotic degradation are oxygen and sunlight.11 Furthermore some plastic polymers can be hydrolyzed.
Plastics
When considering the degradation pathways for plastics it is useful to divide them into two groups: plastics with a carbon–carbon backbone and plastics with heteroatoms in the main chain (Fig. 1). PE, PP, PS and PVC have a backbone which is solely built of carbon atoms. PET and PU plastics have heteroatoms in the main chain.
Plastics with a carbon–carbon backbone
This group includes PE, PP, PS and PVC. The main packaging materials are made of these plastic polymers3,4 and as packaging will be discarded after a short time period it has a higher potential to enter the environment in high quantities.
PE, PP and PS are susceptible to photo-initiated oxidative degradation, which is believed to be their most important abiotic degradation pathway in aerobic outdoor environments.20 This degradation pathway (Fig. 2) can be generally divided into three steps: initiation, propagation and termination.11 In the initiation step chemical bonds in the main polymer chain are broken by light (or by heat in case of thermal initiation) to produce a free radical. In order for photo-initiation to occur, the polymers must contain unsaturated chromophoric groups that absorb light energy.20 PE and PP do not contain any unsaturated double bonds in their polymer backbone, and thus might be expected to be immune to photo-initiated degradation.21 However, small amounts of external impurities or structural abnormalities incorporated into the macromolecular structure can allow for photo-initiated degradation to some extent.20–23 Free radicals are formed when UV-light breaks C–H bonds on the polymer backbone.16,24 In the propagation step the polymer radical reacts with oxygen and forms a peroxy radical.25,26 Besides the formation of hydroperoxides, further complex radical reactions take place and lead to autoxidation.11,26 Propagation ultimately leads to chain scission or crosslinking.27 Termination of the radical reaction occurs when inert products are formed from the combination of two radicals.21,28 Therefore due to oxidation, random chain scission or end-chain scission, branching, crosslinking and formation of oxygen-containing functional groups take place.11,22 Olefins, aldehydes and ketones are expected products of radical termination reactions, and these should be more susceptible to photo-initiated degradation, because they contain unsaturated double bonds. As the molecular weight of the polymers is reduced, the material gets brittle29 and is more susceptible to fragmentation which makes a higher surface area available for further reactions.
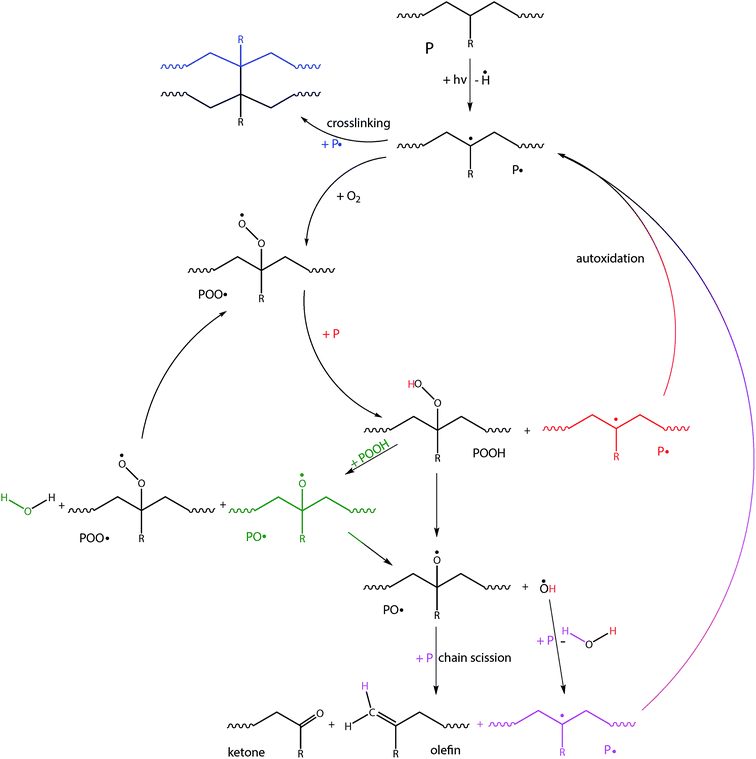 |
| Fig. 2 Abiotic degradation pathways for PE (R = H), PP (R = CH3) and PS (R = aromatic ring); after initiation by photolytic cleavage of a C–H bond on the polymer backbone (P = polymer backbone). | |
Due to the high molecular weight and the lack of functional groups biodegradation of long polyolefin chains is limited.30,31 Therefore high molecular weight PE, PP and PS polymers must be broken down by abiotic degradation to smaller pieces before biodegradation at a measurable rate takes place. If the polymers are too large, they cannot pass through the microbial cellular membranes.18 In addition, abiotic degradation produces carbonyl groups that increase the hydrophilicity of the polymer and thus increase its availability for biodegradation.19 During biodegradation the polymer is converted to its monomers, followed by the mineralization of the monomers.18
Anti-oxidants and stabilizers used as additives inhibit the degradation of the polymer,31 while pro-oxidant additives make it more sensitive towards degradation.32 An optimized amount of starch can be added as well to increase biodegradability, but this will not work without a pro-oxidant.33 Thus degradation rates depend strongly on used additives.
Polyethylene (PE)
Abiotic oxidation of PE by exposure to ultraviolet radiation is the initial and rate-determining step for degradation in the environment.23,26,28,34 During the propagation phase, autoxidation by complex radical reactions takes place,27,28 leading to the formation of oxygenated low molecular weight fragments, like aliphatic carboxylic acids, alcohols, aldehydes, and ketones.23 As oxygen is incorporated into the polymers, the presence of oxygen during this process is essential.28 Random chain scission and cross-linking lead to a decrease or increase in the molecular weight, respectively. A wide range of degradation products are known including propane, propene, ethane, ethene, butene and hexene.19 The material becomes brittle and subject to fragmentation.34
Microorganisms can attack PE at any terminal methyl group35 and biodegradation is found to be faster when the molecular weight is less than 500 Da.23 Smaller polymer fragments produced by abiotic reactions can be biodegraded to shorter segments like esters and acids.19,23 After enzymatic action humus, CO2 and H2O are the ultimate products.19,23
The service life of PE depends strongly on additives and therefore the estimates vary widely. Vasile (2005) suggests that stabilized or cross-linked PE has a service life of 15–20 years in moderate climates.23 Another study calculated that only about 0.1% per year of the carbon that makes up the polymer is transformed into CO2 by biodegradation under best laboratory exposure conditions.11 In the marine environment the degradation processes will be even slower, as the conditions are not optimized for polymer degradation. However, both abiotic and biodegradation are possible but on time scales of decades or longer.
Polypropylene (PP)
PP has a lower stability than PE because every other carbon atom in the backbone is a tertiary carbon that is more prone to abiotic attack than the secondary carbons found in PE.21,36 The reaction mechanisms are similar to PE. Trace impurities in the polymer permit the formation of a PP radical, which will react with oxygen.37 Several radical reactions make both random chain scission and cross-linking possible, but reactions leading to lower molecular weight fragments are predominant.30,38 Besides the reduction of the molecular weight, the formation of new functional groups, especially carbonyl and hydroperoxides are the main effects.19 Products are among others pentane, 2-methyl-1-pentene and 2,4-dimethyl-1-heptene.36 According to the “rules of thumb” the chemical structure has an effect on biodegradability.39 One rule says that chain branching increases the resistance to aerobic biodegradation. Because of the tertiary carbon of PP the susceptibility to microbial degradation is decreased.39,40
Polystyrene (PS)
PS is more susceptible to outdoor weathering.41 When the polymer is irradiated with UV-light, the phenyl ring gets excited and the excitation energy is transferred to the nearest C–H bond.16 This causes cleavage of the hydrogen and the formation of a polymer radical.16 Cross-linking and chain scission are the results with the formation of ketones and olefins.26,36 Because end-chain scission is predominant, styrene monomers have been identified as the main volatile product of degradation.36 Other known products from thermo-oxidation are oligomers of styrene, such as dimer and trimer, benzene, ethylbenzene, α-methylstyrene, phenol, benzyl alcohol, benzaldehyde, ketones (acetophenone) and benzoic acid.43 Since thermo-oxidation and photo-oxidation are similar degradation pathways differing only in their initiation, these known products from thermo-oxidation are also expected degradation products in the marine environment by photo-oxidation. Contrary to abiotic mechanisms, PS is considered to be the most durable thermoplastic polymer towards biodegradation.44 The “rapid” biodegradation observed with PE and PP residues does not occur with PS.45 In order to improve physical properties depending on the end use of the polymer, specific additives can be incorporated.46 Anti-oxidants and UV-stabilizers decrease the degradability and addition of starch promotes biodegradability.42
Poly(vinyl chloride) (PVC)
PVC is the least stable of the high tonnage polymers as it has the highest sensitivity towards UV radiation47,48 and therefore photo-degradation is of highest importance.49 Since PVC monomer units contain only saturated chemical bonds, impurities are required for photo-initiation by UV radiation.48 When exposed to sunlight, dechlorination of PVC is the first step, which leads to the formation of conjugated double bonds in a polyene polymer and hydrochloric acid (Fig. 3), along with a very low amount of other products.50,51 The rate of photo-induced dechlorination of PVC is enhanced under aerobic conditions, in the presence of HCl and for lower molecular weight polymers.36 Humidity, mechanical stress, the presence of other chemicals, and high temperatures also enhance the rate of degradation of PVC.52 Since the dechlorination is autocatalytic, it may continue until there are only traces of chlorine left in the macromolecule.47 Formed unsaturated C
C double-bonds are less stable towards photo-degradation and thus the polymer backbone degrades into smaller fragments. The visual result of degradation is the discoloration of the polymer.47,49 It has been shown that PVC has a high resistance towards biodegradation.29 According to the “rules of thumb” for biodegradation, halogens and especially chlorines increase the resistance to aerobic biodegradation.39 Therefore it is expected that abiotic degradation resulting in dechlorination of the polymer will precede biodegradation.
 |
| Fig. 3 Dechlorination of PVC and formation of polyene. | |
PVC can accept a wide range of additives and plasticizers, which are incorporated to change and improve the characteristics of the plastic material.53,54 Due to the improved application characteristics offered by additives, pure PVC is rarely used.13 Thermal and UV stabilizers are added to extend the lifetime and stability of PVC products that are exposed to sunlight.47 In order to improve the flexibility, plasticizers or flexibilizers are added.36 Stabilizers can be lead compounds, organotin compounds and those of other metals.47 Of particular concern are semi-volatile organotin compounds, which are used in PVC products as stabilizers, and are expected to migrate into the environment over time.55 Since the stabilizers are not chemically bound with the PVC polymer, they can leach out from the polymers, volatilize to the air or be degraded by microbes.13
Plastics with heteroatoms in the main chain
Plastic materials made of polymers consisting of carbon and hetero atoms in the main chain have increased thermal stability compared to polymers with a solely carbon backbone.56 They are potentially susceptible to hydrolytic cleavage of e.g. ester or amide bonds57
Poly(ethylene terephthalate) (PET)
Under marine environmental conditions photo, photo-oxidative and hydrolytic degradation are relevant pathways of PET degradation. Photodegradation leads to cleavage of the ester bond forming a carboxylic acid end group and a vinyl end group directly (Fig. 4), or to radicals, which eventually lead to the formation of a carboxylic acid end group.58 Similar to carbon–carbon backbone plastic polymers (Fig. 2), PET can undergo photo-induced autoxidation via radical reactions.24,56 A hydroperoxide is formed at the methylene group.56 Photo-oxidation results mainly in chain scission.56 The formed carboxylic acid end group has a promoting effect on thermo-oxidative degradation59 and therefore also on photo-oxidative degradation.
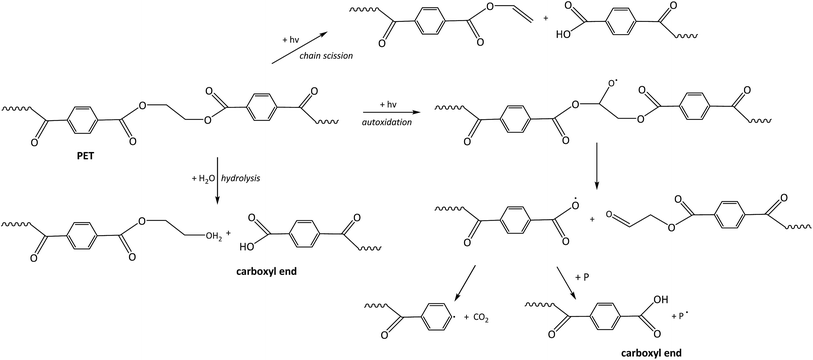 |
| Fig. 4 Abiotic degradation of PET: chain scission induced by radiation (cleavages that lead to radical formation are not shown), photo-induced autoxidation (the initiation and some propagation reactions of the photo-oxidation, which follow the same pattern as for PE, are left out to simplify the mechanism) and hydrolytic degradation. | |
In water PET is susceptible to hydrolytic degradation,29 which is the reverse reaction of one part of the esterification of PET.60 Even though it is known that hydrolysis of PET under room temperature conditions is extremely slow, it is the most important process in the low temperature degradation of PET.56,61 During this process carboxylic acid and alcohol functional groups are formed.60 The rate of hydrolysis is higher under acidic or basic conditions,61 thus hydrolysis of PET is an autocatalytic reaction when carboxylic end groups are formed.60,62
Abiotic weathering of PET in the marine environment is likely to occur predominantly by photo-induced oxidation and hydrolytic degradation processes. The visual effect of these degradation processes is the yellowing of the polymer.56 For thermo-oxidative degradation the results are a reduction in molecular weight of the main polymer and an increase in carboxylic acid end groups.62 Since the photo-oxidative degradation follows similar degradation pathways, these findings can be extrapolated to the environmental situation when the degradation is likely photo-initiated. Hydrolysis also leads to a reduction in molecular weight and an increase in carboxylic acid end groups. Thus carboxylic acids could be formed as free chemicals from photo-induced radical reactions and hydrolysis of PET. PET is highly resistant to environmental biodegradation63 because of its compact structure.64 But Zhang et al. (2004) were able to show a very weak degradation of PET by microbes and lipase.64
Polyurethane (PU)
PU plastics are composed of extremely large and complex polymers with carbon, oxygen and nitrogen in the main chain.65 The ester bond in the backbone is generally more susceptible to degradation.65 Photo-oxidation, hydrolysis and biodegradation of PU materials likely account for the most important degradation processes in the marine environment. Photo-induced oxidation can occur at the α-methylene position.66 After photo-initiation, radical reactions lead to hydroperoxides, following similar processes as shown above for plastic polymers with a carbon–carbon backbone (Fig. 2).66 Hydrolysis of the ester bond of PU is the most prevalent hydrolytic degradation reaction65,67 (Fig. 5). Besides the ester bond, urea and urethane bonds can also be degraded by hydrolysis, but at slower rates.65,66 Acidic conditions accelerate hydrolysis68 and since carboxylic acid end groups are formed, this process is autocatalytic.
 |
| Fig. 5 Hydrolytic degradation of the ester bond of PU. | |
PU is well known to be susceptible to fungal biodegradation,67 but bacterial degradation and degradation by polyurethanase enzymes are also possible.33,69 Similar to hydrolysis, the microbial degradation process can roughly be divided into the degradation of urethane bonds and the degradation of polyol segments, which are the major constituents of PU materials.67 Microorganisms are able to degrade polyester segments of PU relatively easily, while polyether segments of PU are more resistant to microbial attack.30,65
Enzymatic degradation is a more specific degradation mechanism. The enzyme cleaves the polymer chain,70 but as the enzyme is unlikely to diffuse into the bulk of the material due to its size, this process is limited to the surface.71
Degradation leads to polymers with lower molecular weight and can cause deep, usually random cracks on the surface of the plastic material.66 Most of the current resorbable polyurethanes have an ester linkage, which is a hydrolytically labile group and more susceptible to abiotic and biodegradation.65,72
Conclusion
Degradation of plastic polymers floating in the marine environment will take place. For the polymers with a carbon backbone (PE, PP, PS and PVC) abiotic degradation likely precedes biodegradation. Photo-initiated oxidative degradation of PE, PP and PS leads to a decrease in the molecular weight and formation of carboxylic end groups, and UV-light is particularly effective at initiating dechlorination of PVC. Polymer fragments with lower molecular weight formed by chain scission after UV-initiation can then be biodegraded. Plastics that contain heteroatoms in the main chain, like PET and PU, can be degraded by hydrolysis, photo-oxidation, and biodegradation. This leads to the formation of smaller fragments and carboxylic end groups. Several degradation pathways might take place simultaneously, since various factors initiate degradation in the marine environment and therefore degradation products might be more diverse than those expected for any specific pathway.
Polymers are rarely used in pure form and almost all commercial plastics include additives. Thus the described degradation pathways and products are not the only chemicals liberated to ocean water as plastic is weathered. Additives are also released15 and these substances may also degrade to form other environmental pollutants. Stabilizers added to the polymers will enhance resistance towards degradation, but the amounts used in plastic products vary. Generally the degradation rates in the environment will depend on the additives. Thus it is hard to make quantitative statements about the degradation rates, since most experiments we reviewed here were conducted at non-environmentally relevant conditions, and also because different products can vary in their composition. Furthermore other factors can influence the rate. Floating plastic might be shielded from UV radiation by water or a biofilm, which would lead to a reduction in photo-initiated degradation. The state of the weathered plastic also plays a role, as smaller particles and plastics with a high surface cracking are more susceptible towards degradation and some degradation pathways are autocatalytic.
There have so far been very few studies of degradation mechanisms for plastic polymers in the marine environment. Therefore in this review we have relied mostly upon literature from the material science and polymer chemistry literature, and have made extrapolations about potentially relevant degradation pathways and products for the marine environment. In the engineering literature high temperatures that far exceed the temperatures in the marine environment are often used to induce oxidation reactions and these experiments are thus not environmentally relevant. Initiation of oxidation by UV-radiation is possible in the marine environment, but mechanisms and products must be extrapolated from laboratory studies that often rely on short wavelength radiation and strong oxidizing conditions that are also not necessarily environmentally relevant. Therefore more studies of reaction pathways and potential degradation products of plastic polymers under conditions that more closely approximate the marine environment are needed. These experiments would also help to make quantitative extrapolations about degradation rates in the marine environment possible.
Acknowledgements
This research was supported by The Swedish Research Council FORMAS Project Grant #2012-621, “Hazard assessment of chemical pollutants released to the marine environment by degradation of plastic debris”. We thank Marko Filipovic and Sofia Bejgarn for helpful comments on this paper.
Notes and references
-
I. Roy and P. M. Visakh, Polyhydroxyalkanoate (PHA) based blends, composites and nanocomposites, Royal Society of Chemistry, Cambridge, U.K., 2015 Search PubMed
.
-
T. N. Hofer, Marine Pollution: New Research, Nova Science Publishers, New York, 2008 Search PubMed
.
-
PlasticsEurope, Plastics – the Facts 2014/2015-An analysis of European plastics production, demand and waste data, http://www.plasticseurope.org/Document/plastics-the-facts-20142015.aspx?Page=DOCUMENT%26FolID=2, 2015
.
-
PlasticsEurope, Plastics – the Facts 2012 An analysis of European plastics production, demand and waste data for 2011, http://www.plasticseurope.org/documents/document/20121120170458-final_plasticsthefacts_nov2012_en_web_resolution.pdf., 2012
.
- D. K. A. Barnes, F. Galgani, R. C. Thompson and M. Barlaz, Philos. Trans. R. Soc., B, 2009, 364, 1985–1998 CrossRef CAS PubMed
.
- M. R. Gregory, Philos. Trans. R. Soc., B, 2009, 364, 2013–2025 CrossRef PubMed
.
-
K. J. Noone, Managing ocean environments in a changing climate: sustainability and economic perspectives, Elsevier, Burlington, MA, 1st edn, 2013 Search PubMed
.
-
Encyclopedia of consumption and waste: the social science of garbage, SAGE Reference, ed. C. A. Zimring and W. L. Rathje, Thousand, Oaks, Calif, 2012 Search PubMed
.
- M. Cole, P. Lindeque, C. Halsband and T. S. Galloway, Mar. Pollut. Bull., 2011, 62, 2588–2597 CrossRef CAS PubMed
.
-
J. Murphy, Additives for plastics handbooks, Elsevier Science Ltd, Kidlington, Oxford, UK, New York, NY, USA, 2nd edn, 2001 Search PubMed
.
-
Plastics Additives, ed. G. Pritchard, Springer, Netherlands, Dordrecht, 1998, vol. 1 Search PubMed
.
- S. A. Savrik, B. C. Erdogan, D. Balköse and S. Ulku, J. Appl. Polym. Sci., 2010, 116, 1811–1822 CAS
.
- R. Stringer and P. Johnston, Environ. Sci. Pollut. Res., 2001, 8, 146 CrossRef
.
- A. L. Andrady, Mar. Pollut. Bull., 2011, 62, 1596–1605 CrossRef CAS PubMed
.
- R. D. Deanin, Environ. Health Perspect., 1975, 11, 35–39 CrossRef CAS
.
- E. Yousif and R. Haddad, SpringerPlus, 2013, 2, 398 CrossRef PubMed
.
-
Ageing and stabilisation of paper, ed. M. Strlič and J. Kolar, National and University Library, Ljubljana, 2005 Search PubMed
.
- A. A. Shah, F. Hasan, A. Hameed and S. Ahmed, Biotechnol. Adv., 2008, 26, 246–265 CrossRef CAS PubMed
.
-
Handbook of polyolefins, ed. C. Vasile, Marcel Dekker, New York, 2nd ed., rev. and expanded, 2000 Search PubMed
.
- P. Gijsman, G. Meijers and G. Vitarelli, Polym. Degrad. Stab., 1999, 65, 433–441 CrossRef CAS
.
-
N. Grassie and G. Scott, Polymer degradation & stabilisation, Cambridge University Press, Cambridge England, New York, 1988 Search PubMed
.
-
G. Scott, Degradable Polymers Principles and Applications, Springer, Netherlands, Dordrecht, 2002 Search PubMed
.
-
C. Vasile, Practical guide to polyethylene, RAPRA Technology, Shrewsbury, 2005 Search PubMed
.
- E. Pearce, J. Polym. Sci., Polym. Lett. Ed., 1975, 13, 621–622 CrossRef PubMed
.
-
J. F. Rabek, Polymer photodegradation mechanisms and experimental methods, Springer, Dordrecht, 1995 Search PubMed
.
- B. Singh and N. Sharma, Polym. Degrad. Stab., 2008, 93, 561–584 CrossRef CAS PubMed
.
-
M. Tolinski, Additives for polyolefins getting the most out of polypropylene, polyethylene and TPO, William Andrew Pub., Oxford, 2009 Search PubMed
.
-
A. J. Peacock, Handbook of polyethylene: structures, properties and applications, Marcel Dekker, New York, 2000 Search PubMed
.
-
J. W. Summers and E. B. Rabinovitch, in Weathering of Plastics, Elsevier, 1999, pp. 61–68 Search PubMed
.
-
M. Chanda, Plastics technology handbook, CRC Press/Taylor & Francis Group, Boca Raton, FL, 4th edn, 2007 Search PubMed
.
- I. Jakubowicz, Polym. Degrad. Stab., 2003, 80, 39–43 CrossRef CAS
.
-
T. Hamaide, R. Deterre and J.-F. Feller, Environmental impact of polymers, ISTE Ltd/John Wiley and Sons Inc, Hoboken, NJ, 2014 Search PubMed
.
- Y. Zheng, E. K. Yanful and A. S. Bassi, Crit. Rev. Biotechnol., 2005, 25, 243–250 CrossRef CAS PubMed
.
-
M. Hakkarainen and A.-C. Albertsson, in Long Term Properties of Polyolefins, ed. A.-C. Albertsson, Springer, Berlin, Heidelberg, 2004, vol. 169, pp. 177–200 Search PubMed
.
- M. Booma, S. E. Selke and J. R. Giacin, J. Elastomers Plast., 1994, 26, 104–142 CrossRef CAS PubMed
.
-
C. Beyler and M. Hirschler, Thermal Decomposition of Polymers, in SFPE Handbook of Fire Protection Engeniering, 3rd edn, 2002 Search PubMed
.
-
Handbook of fiber chemistry, ed. M. Lewin and E. M. Pearce, Marcel Dekker, New York, 2nd ed., rev. and expanded, 1998 Search PubMed
.
- A. V. Shyichuk, D. Y. Stavychna and J. R. White, Polym. Degrad. Stab., 2001, 72, 279–285 CrossRef CAS
.
-
G. S. Sayler, J. Sanseverino and K. L. Davis, Biotechnology in the Sustainable Environment, Springer, US, Boston, MA, 1997 Search PubMed
.
-
D. P. Singh and S. K. Dwivedi, Environmental microbiology and biotechnology, New Age International Ltd., New Delhi, 2004 Search PubMed
.
- M. D. Faber, Enzyme Microb. Technol., 1979, 1, 226–232 CrossRef CAS
.
- S. Kiatkamjornwong, M. Sonsuk, S. Wittayapichet, P. Prasassarakich and P.-C. Vejjanukroh, Polym. Degrad. Stab., 1999, 66, 323–335 CrossRef CAS
.
- A. Hoff, S. Jacobsson, P. Pfäffli, A. Zitting and H. Frostling, Scand. J. Work, Environ. Health, 1982, 8, 1–60 Search PubMed
.
- R. Mor and A. Sivan, Biodegradation, 2008, 19, 851–858 CrossRef CAS PubMed
.
- P. H. Jones, D. Prasad, M. Heskins, M. H. Morgan and J. E. Guillet, Environ. Sci. Technol., 1974, 8, 919–923 CrossRef CAS
.
- J. L. Gurman, L. Baier and B. C. Levin, Fire Mater., 1987, 11, 109–130 CrossRef CAS PubMed
.
-
J. W. Nicholson, The chemistry of polymers, RSC Pub., Cambridge, 2012 Search PubMed
.
-
Degradation and Stabilisation of PVC, ed. E. D. Owen, Springer, Netherlands, Dordrecht, 1984 Search PubMed
.
- D. Feldman, J. Polym. Sci., Part C: Polym. Lett., 1986, 24, 355 CrossRef PubMed
.
- T. T. Nagy, B. Ivan, B. Turcsanyi, T. Kelen and F. Tudös, Polym. Bull., 1980, 3, 613–620 CrossRef CAS
.
-
K. Pielichowski and Rapra Technology Limited, Thermal degradation of polymeric materials, Rapra Technology, Shawbury, 2005 Search PubMed
.
- I. Jakubowicz, N. Yarahmadi and T. Gevert, Polym. Degrad. Stab., 1999, 66, 415–421 CrossRef CAS
.
-
Handbook of thermoplastics, ed. O. Olabisi, Marcel Dekker, New York, 1997 Search PubMed
.
- T. Yoshioka, T. Kameda, M. Ieshige and A. Okuwaki, Polym. Degrad. Stab., 2008, 93, 1822–1825 CrossRef CAS PubMed
.
- C. J. Weschler, Atmos. Environ., 2009, 43, 153–169 CrossRef CAS PubMed
.
-
S. Venkatachalam, S. G. Nayak, J. V. Labde, P. R. Gharal, K. Rao and A. K. Kelkar, in Polyester, ed. H. E.-D. Saleh, InTech, 2012 Search PubMed
.
- R.-J. Müller, I. Kleeberg and W.-D. Deckwer, J. Biotechnol., 2001, 86, 87–95 CrossRef
.
-
D. R. Fagerburg and H. Clauberg, in Wiley Series in Polymer Science, ed. J. Scheirs and T. E. Long, John Wiley & Sons, Ltd, Chichester, UK, 2004, pp. 609–641 Search PubMed
.
- D. N. Bikiaris and G. P. Karayannidis, Polym. Degrad. Stab., 1999, 63, 213–218 CrossRef CAS
.
-
Handbook of Engineering and Speciality Thermoplastics: Polyethers and Polyesters, ed. S. Thomas and P. M. Visakh, John Wiley & Sons, Inc., Hoboken, NJ, USA, 2011 Search PubMed
.
- N. S. Allen, M. Edge, M. Mohammadian and K. Jones, Eur. Polym. J., 1991, 27, 1373–1378 CrossRef CAS
.
-
B. Culbert and A. Christel, in Wiley Series in Polymer Science, ed. J. Scheirs and T. E. Long, John Wiley & Sons, Ltd, Chichester, UK, 2004, pp. 143–194 Search PubMed
.
- H. Webb, J. Arnott, R. Crawford and E. Ivanova, Polymers, 2012, 5, 1–18 CrossRef PubMed
.
- J. Zhang, X. Wang, J. Gong and Z. Gu, J. Appl. Polym. Sci., 2004, 93, 1089–1096 CrossRef CAS PubMed
.
-
M. Szycher, Szycher's handbook of polyurethanes, Taylor & Francis, Boca Raton, FL, 2nd edn, 2013 Search PubMed
.
-
N. M. K. Lamba, Polyurethanes in biomedical applications, CRC Press, Boca Raton, 1998 Search PubMed
.
- T. Nakajima-Kambe, Y. Shigeno-Akutsu, N. Nomura, F. Onuma and T. Nakahara, Appl. Microbiol. Biotechnol., 1999, 51, 134–140 CrossRef CAS
.
-
C. Hepburn, Polyurethane Elastomers, Springer, Netherlands, Dordrecht, 1992 Search PubMed
.
- L. H. G. Morton and S. B. Surman, Int. Biodeterior. Biodegrad., 1994, 34, 203–221 CrossRef CAS
.
- D. G. Duguay, R. S. Labow, J. P. Santerre and D. D. McLean, Polym. Degrad. Stab., 1995, 47, 229–249 CrossRef CAS
.
- G. A. Skarja and K. A. Woodhouse, J. Biomater. Sci., Polym. Ed., 2001, 12, 851–873 CrossRef CAS PubMed
.
- A. E. Hafeman, K. J. Zienkiewicz, A. L. Zachman, H.-J. Sung, L. B. Nanney, J. M. Davidson and S. A. Guelcher, Biomaterials, 2011, 32, 419–429 CrossRef CAS PubMed
.
|
This journal is © The Royal Society of Chemistry 2015 |