Closing the loop: captured CO2 as a feedstock in the chemical industry
Received
21st August 2015
, Accepted 16th September 2015
First published on 16th September 2015
Abstract
The utilization of ‘captured’ CO2 as a feedstock in the chemical industry for the synthesis of certain chemical products offers an option for preventing several million tons of CO2 emissions each year while increasing independence from fossil fuels. For this reason, interest is increasing in the feasibility of deploying captured CO2 in this manner. Numerous scientific publications describe laboratory experiments in which CO2 has been successfully used as a feedstock for the synthesis of various chemical products. However, many of these publications have focused on the feasibility of syntheses without considering the ancillary benefits of CO2 emissions reduction if the CO2 is sourced from effluent or the potential profitability of this process. Evaluating these environmental and economic benefits is important for promoting the further development of benign CO2 applications. Given the multitude of CO2 utilization reactions in the laboratory context, an initial assessment must be undertaken to identify those which have the most potential for future technical exploration and development. To achieve this, 123 reactions from the literature were identified and evaluated with the help of selection criteria specifically developed for this project. These criteria incorporate both the quantitative potential of reducing CO2 and possible economic benefits of these syntheses. The selected reactions are divided into bulk and fine chemicals. Of the bulk chemicals, formic acid, oxalic acid, formaldehyde, methanol, urea and dimethyl ether, and of the fine chemicals, methylurethane, 3-oxo-pentanedioic acid, 2-imidazolidinone, ethylurethane, 2-oxazolidone and isopropyl isocyanate, mostly fulfil the selection criteria in each category.
Broader context
In order to achieve global greenhouse gas reduction targets, in addition to increasing the deployment of renewable energy technologies and improving efficiency, carbon capture and storage (CCS) will be an important means of CO2 reduction in the future. However, even with the complete decarbonization of electricity generation through the widespread implementation of renewable power, huge amounts of CO2 from process emissions will still be emitted by the cement, steel and chemical industries, which can be greatly reduced with CCS. The utilization of a portion of the collected CO2 as a feedstock in the chemical industry in turn presents a means of reusing, rather than merely disposing of it. Additionally, the sourcing of CO2 as a feedstock would reduce the chemical industry's dependence on fossil fuels. In the scientific literature, numerous experiments have successfully demonstrated the viability of CO2 as a feedstock. However, not all CO2 utilization reactions have the potential to utilize significant amounts of CO2 or be profitably implemented. Therefore, the present study focuses on those CO2 based reactions which do and thus merit further study and compares these to conventional process routes.
|
1. Introduction
Currently, only a handful of commercial processes use CO2 as a feedstock for the production of organic chemical products. These processes synthesize urea, methanol, salicylic acid, organic carbonates and polycarbonates (see Table 1). Globally, these processes entail approximately 116 million tons of CO2 consumption per year, 94% of which goes toward the production of urea. Although urea production currently accounts for the most quantitatively significant utilization of CO2 as a feedstock, it should also be noted that this process produces more CO2 than it consumes. The reason for this is that the origin of the CO2 utilized constitutes only part of the resulting CO2 emissions, which arise during the synthesis of the necessary co-reactant, ammonia.1
Table 1 Industrial production volume of products made of CO2, utilized mass of CO2 per mass product and bounded CO2 in the product
Product |
Production volume (million tons per annum) |
Bounded CO2 in product (million tons per annum) |
Ref. |
Admixing of CO2 to synthesis gas.
Direct hydrogenation of CO2 with H2.
|
Urea |
150 |
109.5 |
2
|
Methanola |
4.4 |
6 |
3
|
Methanolb |
0.004 |
0.00548 |
4
|
Salicylic acid |
0.17 |
0.054 |
4
|
Organic carbonate |
0.1 |
0.043–0.049 |
5
|
Bisphenol-A polycarbonate |
0.6 |
0.102 |
6
|
Polypropylene carbonate |
0.07 |
0.03 |
7
|
|
|
Sum ≈ 116 |
|
Notwithstanding of this, the current use of CO2 as a feedstock for chemicals corresponds to only 0.36% of global CO2 emissions, which amounted to 32.3 billion tons in 2014.8 In its World Energy Outlook 2012,9 the International Energy Agency (IEA) outlines the “450 Scenario”, that the growth of average global temperature could be limited to 2 °C if CO2 emissions total no more than 22 billion tons a year by 2035. To achieve this aim, in addition to improvements in efficiency, renewable energy technology and electricity saving, Carbon Capture and Storage (CCS)10–12 will be an important CO2 reducing option in the future.13,14 The IEA states that by 2035, 2.1 billion tons of CO2 per year should be prevented from atmospheric emission by means of CCS. In a green paper, the German DECHEMA (Society for Chemical Engineering and Biotechnology)15 estimates the future potential of CO2 as a feedstock for polymers and basic chemicals to be at least 178 million tons per year, a tenfold potential for synthetic fuels. Compared to the huge demand for geological storage that CCS entails (see above), the utilization of CO2 as a feedstock for chemicals therefore has less quantitative potential for mitigating CO2 emissions by comparison to that of reusing CO2 as a feedstock for the synthesis of fuels. Moreover, in the future, there is potential for new applications of CO2 as a feedstock, for example in the context of so-called power-to-gas technologies. These technologies are primarily for the purpose of storing temporary excess wind and solar power through the production of hydrogen via water electrolysis, followed by the conversion of hydrogen, with CO2, into fuels like synthetic methane.16,17 However, these technologies will only consume large amounts of CO2 in conjunction with the expansion of renewable power and a high degree of capacity utilization by the electrolyzers.
Although the reuse potential of captured CO2 as a feedstock for the synthesis of chemicals is limited, it offers other benefits, such as increasing the chemical industry's independence from fossil feedstock such as naphtha, natural gas, oil and coal. Additionally, if the CO2 utilization process is located directly at the emission source, the energy and costs required for CO2 transport and the need for storage can be avoided. In the literature contains numerous scientific publications that deal with the utilization of carbon dioxide as a feedstock, but usually concerning the feasibility of the syntheses, new catalysts or research into the reaction mechanism, without paying attention to CO2 reduction potential or the profitability of these reactions. Furthermore, there are several reviews concerning the kind of reaction mechanisms and products that can be produced, depending on the reactant of CO2 (e.g., ref. 3, 18–21). For the present study, 123 chemical reactions that utilize CO2 as a feedstock were identified. The synthesis of polymers or fuels will not be considered, as the focus is on the conversion of CO2 into organic products that can substitute conventional chemical products. To evaluate the CO2 reduction and economic potential of the identified chemicals, special selection criteria were developed. These criteria should identify the most suitable products with the greatest potential for a detailed direct comparison of the CO2 based and conventional manufacturing process, without incurring enormous expenditure for the evaluation of each published reaction. Using these selection criteria, it was possible to rank the numerous reactions in the literature into a list to determine their potential for CO2 re-utilization.
2. Identification of CO2 utilization reactions
For the identification of CO2 utilization reactions in the literature, the knowledge of the possible synthesis products is a basic requirement. For this reason, Table 2 displays an overview of important substance groups that can be synthesized with CO2 as a feedstock, depending on the nature of the reactants. On this basis, a search was conducted in the online database SCI-Finder22 for reactions that, when combined with the particular reactants, lead to the substance groups described (see Tables 3 and 5). For the purpose of this research, 123 CO2 utilization reactions were collected. The products of the registered reactions were divided into 23 bulk chemicals (Table 3) and 100 fine chemicals (Table 5). By definition, a bulk chemical is a substance with a global production volume of over 10
000 tons per annum, while a fine chemical constitutes less.23 In the present study, the production rates of chemicals related to the production of the 27 states of the European Union in 2011. As no exact information on the boundary between bulk and fine chemicals in the EU could be found in the literature, the boundary is estimated on the basis of the fact that approximately 17%24 of chemicals produced worldwide are produced in the EU at a volume of approximately 1700 tons per annum. With respect to the next order of magnitude, this value is rounded to 1000 tons per annum, which is defined as the boundary between bulk and fine chemicals for the purposes of this work. The division of the resulting reaction products is necessary, as the later defined selection criteria for the bulk and fine chemicals are different. Therefore, the evaluation of CO2 based reaction products is separated for each category. In Table 3, the 23 bulk chemicals considered with their respective gross reactions and the reference is listed.
Table 2 Possible producible substance groups with CO2 as a feedstock, depending on the nature of the reactants
Reactant |
Substance groups |
Examples in |
Organic compounds with “active hydrogen” |
Carboxylic acids and coumarins |
18 and 25
|
Organometallic compounds |
Carboxylic acids |
18 and 26
|
Alkenes |
Unsaturated carboxylic acids |
19, 20 and 27
|
Conjugated dienes |
Lactones and unsaturated ester |
19, 20 and 27
|
Allenes |
Pyrones and unsaturated ester |
19, 20 and 27
|
Methylcyclopropane |
Lactones und pyrones |
19, 20 and 27
|
Alkynes |
Lactone und pyrones |
19, 20 and 27
|
Hydrogen |
Carboxylic acids, ether, alcohols and aldehydes |
19 and 28
|
Epoxides or dioles |
Cyclic carbonates |
29 and 30
|
Alcohols |
Linear carbonates |
31–33
|
Ammonia |
Urea |
18 and 19
|
Amines |
Linear urea derivatives and isocyanates |
25 and 34
|
Diamines |
Cyclic urea derivatives |
35
|
β-Amino alcohols or aziridines |
Cyclic carbamates |
36–39
|
Amines or ammonia + alcohols |
Linear carbamates |
40
|
Table 3 Catalogue of the 23 bulk chemicals considered
Table 5 shows the 100 fine chemicals identified with their identification number (ID), gross reaction, European Union Community Production (ProdCom)41 number and the reference that reported these reactions. The individual products or product groups are registered in the database by their ProdCom number. Table 4 displays the ProdCom numbers of product groups to which the fine chemicals considered could be allocated.
Table 4 Description of the ProdCom numbers 201171
ProdCom no. |
Explanation |
20143475 |
Carboxilic acid with alcohol, phenol, aldehyde or ketone functions |
20143440 |
Aromatic polycarboxylic acids, their anhydrides, halides, peroxides, peroxyacids and their halogenated, sulphonated, nitrated or nitrosated derivatives (excluding esters of orthophthalic acid, phthalic anhydride, terephthalic acid) |
20143370 |
Aromatic monocarboxylic acids, (anhydrides), halides, peroxides, peroxyacids, derivatives excluding benzoic acid, phenylacetic acids their esters, benzoyl peroxide, benzoyl chloride |
20145210 |
Heterocyclic compounds with oxygen only hetero-atom(s) (including coumarin; methylcoumarins and ethylcoumarins) (excluding other lactones) |
21103110 |
Lactones (excluding coumarin, methylcoumarins and ethylcoumarins) |
20143310 |
Unsaturated acyclic and alicyclic monocarboxylic acids, their anhydrides, halides, peroxyacids (excluding fomic acid, acetic acid, butyric acid) |
20143383 |
Acyclic and alicyclic polycarboxylic acids |
20145225 |
Heterocyclic compounds with oxygen only hetero-atom(s) (excluding other lactones) |
20144310 |
Urea and their derivatives |
21102070 |
Cyclic amides and their derivatives-(including cyclic carbamates) |
21102060 |
Acyclic amides and their derivatives-(including acyclic carbamates) |
20144450 |
Isocyanates |
Table 5 Catalogue of the 100 fine chemicals considered
3. Definition of the selection criteria
This section outlines the selection criteria for the evaluation of the above collected CO2 utilization reactions (Tables 3 and 5) to identify the most suitable reactions relating to quantitative potential to reduce CO2 and the possible economic interests of the industry in these reactions.
3.1 Bulk chemicals
For the evaluation of CO2 utilization reactions on each other, following selection criteria for bulk chemicals were defined:
3.1.1 Specific mass of CO2 as a feedstock.
The specific mass of CO2 as a feedstock is the mass of CO2 necessary to produce, for instance, one kilogram of a product. This can be calculated using eqn (1) and the information from Table 3. | 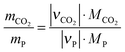 | (1) |
mCO2 = mass of carbon dioxide, kg; mP = mass of product, kg; νCO2 = stoichiometric coefficient of carbon dioxide; νP = stoichiometric coefficient of the product; MCO2 = molar mass of carbon dioxide (0.04401 kg mol−1); MP = molar mass of the product.
The specific mass of CO2 as feedstock for the synthesis of the product is of interest, as this can vary over a wide range, depending on the resulting product and byproduct. The greater the specific mass of CO2 used is, the more CO2 can be inserted as feedstock for the production of a given amount of a chemical.
3.1.2 CO2 avoidance potential.
CO2 avoidance is an important selection criterion, as it defines the maximum quantitative potential for the utilization of CO2 as a feedstock for the complete substitution of the conventional synthesis of a chemical with the CO2 based method. At this point, CO2 avoidance potential is defined as the product of the specific mass of CO2 per mass of product (eqn (1)) and the production volume of the synthesis product within the 27 states of the European Union (EU), as of 2011. In this manner, the CO2 avoidance potential expresses the mass of CO2 that can be theoretically used without regard to power emissions, heat or reactant allocation by the wholesale substitution of the conventional process within the EU. The required data on the production volumes were primary determined using the ProdCom database41 of the Statistical Office of the European Union (EUROSTAT) and, secondarily, with the database of the European Chemical Agency (ECHA).96
3.1.3 Relative added value.
The criterion “relative added value” is a measure of profit and considers the economic aspect of the evaluation. The higher the relative added value is, the more attractive the reaction is for industrial implementation. The relative added value is defined as the difference between the value of the product and price of the necessary educts (CO2 and reactants) divided by the product's value according to eqn (2). | 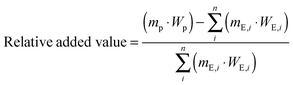 | (2) |
mP = mass of product, kg; mE,i = mass of educt i, kg; WP = specific value of product, € per kg; WE,i = specific price of educt i, € per kg.
The starting substances and products considered refers to the gross reaction equation (see Table 3). Catalysts and auxiliary reagents were not considered with the calculation of relative added value. As a reason for the multitude of reactions and the missing thermodynamic data values of most of the considered chemicals, it is assumed that the educts react to 100% of the desired product. At this point, therefore, the relative value added is only a theoretical number for an evaluation of the reactions. For the calculation of the “real” relative value added, it is necessary to consider all manufacturing costs entailed in the process. At this point, this is not possible because most CO2 based reactions for industrial processes are not yet extant and must first be designed, which is beyond the scope of this article. The specific values of the product and specific prices of the educts were at first determined by the ProdCom database and secondly with the online trading platform http://Alibaba.com.97
3.1.4 Independence from fossil reactants.
A special criterion for the bulk chemicals is the evaluation of whether it is possible to avoid fossil carbon as a feedstock for the production of the reactants. This means that the carbon in the synthesis product results exclusively from the captured CO2 molecule. This selection criterion highlights the fact that after the disposal of the product at the end of its lifetime through combustion, the amount of CO2 released is equivalent to the amount which was used as a feedstock for the synthesis. By reusing captured CO2, no additional CO2 is emitted through the use of other fossil sources. As a result of the reuse of the carbon source, the disposal of the product generates no additional CO2 emissions or fossil feedstock demand compared to a synthesis of the complete product or the reactants using fossil sources. An example for the possible independence of fossil reactants is the reaction of CO2 with hydrogen to methanol under the condition that the hydrogen is produced by water electrolysis.
3.2 Fine chemicals
In some cases, the selection criteria for fine chemicals are different than for those of bulk chemicals. For example, the independence criterion of fossil reactants will be eliminated, as the fine chemicals are generally relatively large molecules with only a small part of carbon deriving from CO2. Moreover, for the fine chemicals, some new selection criteria were defined to achieve a more sensitive relative evaluation amongst the products considered. The following selection criteria for fine chemicals were therefore defined:
3.2.1 Specific mass of CO2 as feedstock.
The criterion of specific mass of CO2 as feedstock is defined similarly to the bulk chemical (see Section 3.1.1). By using the information of Table 5 and eqn (1), its value can be calculated.
3.2.3 Production volume of the substance groups.
In terms of fine chemicals, the criterion production volume of the substance groups defined by the ProdCom database replaces the selection criterion of CO2 avoidance potential of the bulk chemicals. The reason for this is that in the context of this study, it was not possible to detect individual production volumes for all of the fine chemicals. However, the ProdCom database also contains the production volumes of the chemical substance groups (see Table 4), in which the 100 fine chemicals considered, displayed in Table 5, can be allocated. However, it must be noted that the substance groups of the ProdCom database do not only contain the fine chemicals discussed in this study. Nevertheless, the production volumes of the substance groups were used to evaluate the relative quantities of the chemicals. As a result, this selection criterion is not tailored to a special reaction product and will only be respected by the total evaluation at one quarter.
3.2.3 Technical availability.
The criterion “technical availability” expresses which of the 100 fine chemicals can be bought in industrial-scale volumes. This only considers chemical products that are produced in larger quantities and have the potential to be produced over a new technology, such as with CO2 as feedstock. For chemicals with a very small production volume only for research applications, a modification of the conventional production route is generally neither economical nor represents a significant contribution for the avoidance of CO2 emissions by using CO2 as feedstock. The technical availability of the chemicals was determined using the inventory of the online trading platform, http://Alibaba.com,97 and the online directory of manufacturers and suppliers Chemical Register.98
3.2.4 Relative added value.
The relative added value is defined in similar terms to the bulk chemicals (see Section 3.1.3). This value can be calculated using the information from Table 5 and eqn (2). Similarly to the selection criterion outlined above though, it was not possible to identify the individual market value of all educts and products of the 100 fine chemicals considered in this study. Therefore, if no individual price could be determined, the average prices from the ProdCom database for the substance groups were used for calculation. As with the selection criterion above, this selection criterion will only be observed by the total evaluation for one quarter, as the prices could not be determined for every chemical.
3.2.5 Scientific relevance.
In this study, the criterion of “scientific relevance” is defined as the number of related references of a certain chemical, which is determined by the database SCI-Finder (compare Section 3). This selection criterion should differentiate chemicals with relatively rare applications, which are a part of the basic research or are specially synthesized and substances with a multitude of publications which can be used in many applications or which are constituents of the feedstock of many other products. Only for the second kind of chemicals might a new synthesis route with CO2 as feedstock be of interest from an ecological and economic point of view. The number of references to the 100 fine chemicals considered was determined during January and April 2014.
4. Evaluation of the CO2 utilization reactions
The aim of this section is to identify the most suitable CO2 utilization reactions from the above (Tables 3 and 5) listed reactions from the literature relating to the potential of CO2 reduction and profitability. The basis for the evaluation is the selection criteria defined in Section 3. Using a point system, for certain value ranges, points between 1 and 5 will be assigned increasing numbers of which indicate that the significance or potential of CO2 utilization reactions is higher. The value ranges for the points are distributed in such a way, that there is a homogeneous distribution over all of the determined values. Finally, all points from the different selection criteria will be added and a ranking list for bulk and fine chemicals created separately.
4.1 Evaluation of the bulk chemicals syntheses
4.1.1 The specific mass of CO2 as a feedstock.
Fig. 1 illustrates the specific mass of CO2 as a feedstock, as well as the value ranges of the points for evaluation. The specific consumed mass of CO2 varies between 0.31 for the synthesis of salicylic acid and 1.91 for the synthesis of dimethyl ether. The low value for salicylic acid is a result of the relatively low content of CO2 in the molecule, as 68 wt% of the acid is provided by the reactant phenol (see Table 3). In contrast, two CO2 molecules and six light hydrogen molecules lead to dimethyl ether. Therefore, dimethyl ether consists of 87 wt% of CO2, while at the same time three oxygen molecules of the two CO2 molecules react to water. In all four cases, the conversion to water led to the high specifically used mass of CO2, but with the disadvantage that a part of the reactive hydrogen reacts to unreactive water and is not bounded in the product.
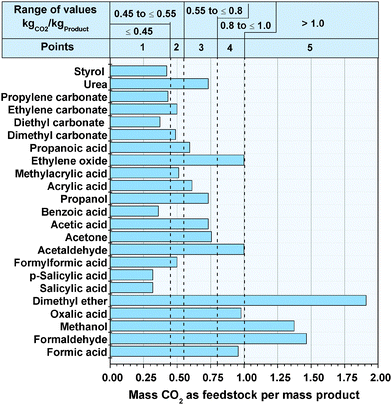 |
| Fig. 1 Specific mass of CO2 as feedstock for the synthesis of the bulk chemicals, as well as the value ranges of the points. | |
4.1.2 CO2 avoidance potential.
The CO2 avoidance potential of the bulk chemicals and value ranges for the points for the evaluation are illustrated in Fig. 2. The CO2 avoidance potential for the entire substitution of the conventional production processes by the CO2 based route within the 27 states of the EU is for the most considered bulk chemicals below one million tons per year. A significantly higher CO2 avoidance potential achieves a synthesis of urea with ∼40 million tons CO2 per year. However the synthesis of urea via CO2 as feedstock is already the only conventional process route.2 Therefore, no substitution potential exists.
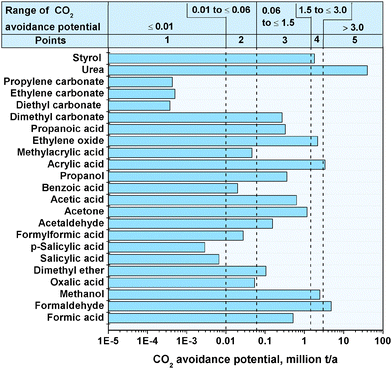 |
| Fig. 2 CO2 avoidance potential for bulk chemicals within the EU for 2011. | |
4.1.3 Relative added value.
At this point, it is necessary to determine the relative added value of the 23 bulk chemicals. For the calculation of the relative added value (eqn (2)) for the reactions with hydrogen, the hydrogen price was defined at 5.22 € per kgH2. This value corresponds to the average manufacturing costs of hydrogen produced by alkaline water electrolysis operated with wind power.99 Although the average cost of conventional hydrogen from natural gas reforming is much lower (∼1.22 € per kgH299), the specific reaction-dependent CO2 emissions for hydrogen production from natural gas are ∼5.5 kgCO2 kgH2−1. Consequently, the process-dependent CO2 emissions of conventional hydrogen production are in the range of the CO2 used as feedstock in the reactions, despite the relatively low specifically used masses of hydrogen. As a reason for this, the utilization of CO2 under these conditions will not lead to the aim of a reduction in CO2 emissions. Hydrogen derived from alkaline water electrolysis operated by renewable energy technologies is an opportunity to produce hydrogen with very low CO2 emissions. For the feedstock price of CO2, different cases (see Fig. 3) are considered, like the assumption that CO2 is free of charge or that the market price of 8.78 €-cent per kg41 has to be paid. In another case it is assumed that a CO2 emitter pays the average CO2 certificate price (8.0 €-cent per kg, May 2015100) for the consumption or sale of CO2 for this price. As Fig. 3 illustrates, the different prices of CO2 considered have no significant influence on the relative added value. The reason for this is that even for the highest considered price of 8.78 €-cent per kg, the prices for the other reactants and the resulting product are generally ten times higher. The relative added value for the synthesis of propionic acid, dimethyl ether, methanol and formaldehyde is negative because the prices of the reactants are higher than the value of the products. All of these reactions have in common the fact that only hydrogen is a reactant of CO2, the price for which was set to 5.22 € per kgH2 for the afore-mentioned reason. Therefore, the synthesis of these products is only profitable if the manufacturing costs of hydrogen, produced by water electrolysis operated with renewable energies, decreases in the future. An exception is the synthesis of formic acid, although hydrogen is used as a reactant. The necessary amount of hydrogen is very low (0.04 kgH2 kgHCOOH−1), because no hydrogen is consumed for the formation of water.
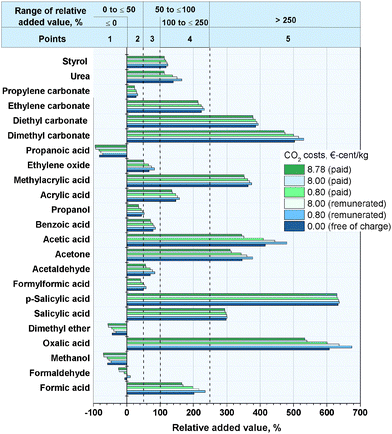 |
| Fig. 3 Relative added value by the synthesis of the bulk chemicals for different CO2 prices. | |
4.1.4 Independence from fossil reactants.
In the condition where hydrogen is produced by water electrolysis operated with renewable energy, for the synthesis of propionic acid, dimethyl ether, methanol, formaldehyde and formic acid, there is an opportunity for complete independence on fossil sources as feedstock, as the only carbon feedstock is CO2. For the CO2 based synthesis of dimethyl carbonate, diethyl carbonate and urea, an indirect independence of fossil sources as feedstock is possible (see Table 6). For the synthesis of dimethyl carbonate, the necessary reactant methanol (see Table 3) can be produced over the CO2 based route with renewable hydrogen and for the synthesis of diethyl carbonate, bio-ethanol can serve as the reactant. The conventional synthesis of urea occurs with ammonia and CO2, at which point the ammonia is produced via the reforming of natural gas with ambient air.2,101 At this point, it is possible to produce urea directly using renewable hydrogen and CO2 without the previous steps utilizing fossil sources. Table 6 illustrates the points allocated for the evaluation of the criterion, “independence from fossil reactant,” for the 23 bulk chemicals considered.
Table 6 Evaluation of the independence of fossil sources of the reactants
Name |
Independent of fossils |
Points |
Formic acid |
Yes |
5 |
Formaldehyde |
Yes |
5 |
Methanol |
Yes |
5 |
Styrol |
No |
1 |
Oxalic acid |
Yes |
5 |
Dimethyl ether |
Yes |
5 |
Salicylic acid |
No |
1 |
p-Salicylic acid |
No |
1 |
Formylformic acid |
No |
1 |
Acetaldehyde |
No |
1 |
Acetone |
No |
1 |
Acetic acid |
No |
1 |
Benzoic acid |
No |
1 |
Propanol |
No |
1 |
Acrylic acid |
No |
1 |
Methacrylic acid |
No |
1 |
Ethylene oxide |
No |
1 |
Propanoic acid |
No |
1 |
Dimethyl carbonate |
Indirect |
2.5 |
Diethyl carbonate |
Indirect |
2.5 |
Ethylen carbonate |
No |
1 |
Propylen carbonate |
No |
1 |
Urea |
Indirect |
2.5 |
4.1.5 Ranking of bulk chemical synthesis.
With the addition of all points of the selection criteria from Sections 4.1.1–4.1.4, it is possible to create a ranking list. The synthesis with the most points is favored CO2 utilization reactions regarding the potential to reduce CO2 emissions and their economic feasibility. Formic acid, oxalic acid and formaldehyde achieve 16 of the maximum achievable 20 points.
For formaldehyde as well as for methanol, urea and dimethyl ether (see Table 7) point deduction occurs as a result of the negative relative added value (see Fig. 3) because of the high price of renewable hydrogen. Formic acid and oxalic acid do not achieve the maximal score due to the relatively low CO2 avoidance potential.
Table 7 Ranking list of the 23 bulk chemicals after the accumulation of points of the selection criteria from Sections 4.1.1–4.1.4
Name |
Points |
Name |
Points |
Formic acid |
16 |
Methylacrylic acid |
10 |
Oxalic acid |
16 |
Styrol |
10 |
Formaldehyde |
16 |
Diethyl carbonate |
9.5 |
Methanol |
15 |
Propanol |
9 |
Urea |
14.5 |
Ethylene carbonate |
8 |
Dimethyl ether |
14 |
Salicylic acid |
8 |
Acrylic acid |
13 |
p-Salicylic acid |
8 |
Dimethyl carbonate |
12.5 |
Propionic acid |
8 |
Acetone |
12 |
Formylformic acid |
7 |
Acetic acid |
12 |
Benzoic acid |
7 |
Ethylene oxide |
12 |
Propylene carbonate |
5 |
Acetaldehyde |
11 |
|
|
4.2 Evaluation of the fine chemicals synthesis
4.2.1 Specific mass of CO2 as feedstock.
Analogous to the bulk chemicals, Fig. 5 (see Appendix) illustrates the specific mass of CO2 as feedstock, as well as the value ranges for the points for the evaluation of the fine chemicals. The specific consumed mass of CO2 varies between 0.11 for N,N′-bis(benzhydryl)urea (ID 58) and 0.77 for methyl isocyanate (ID 99). All in all, 46% of the fine chemicals achieve a specific CO2 consumption higher than 0.3 and 4% higher than 0.5. The reason for this is that the reactants of CO2 are generally large molecules and CO2 only contributes to a small portion of the final products.
4.2.2 Production volume of the substance groups.
Fig. 4 illustrates the production volumes of the substance groups (see Table 4) referred to in the ProdCom database, into which the 100 fine chemicals considered were assigned. The production volumes of the substance groups vary between 0.004 and 1.8 million tons per year. It should be remembered though that Fig. 4 not only shows the production volume of the 100 fine chemicals, but also includes the production volume of all chemicals within the EU which belong to these substance groups. Nevertheless, the production volumes of the substance groups are used to evaluate the relative quantities of the chemicals amongst each other. Because of the inexact availability of the database, this selection criterion is only included in one quarter of the total evaluation.
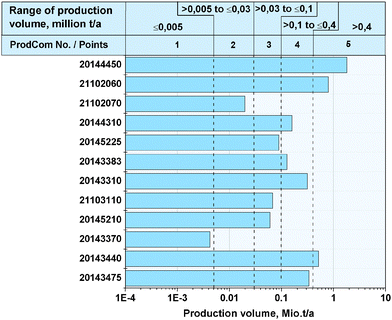 |
| Fig. 4 Production volume of the substance groups referred to in the database, in which the 100 considered fine chemicals are assigned. | |
4.2.3 Technical availability.
In Table 8, the IDs (compare Table 5) of the CO2 utilization reaction, which leads to products that are not technically available, are summarized. These 25 reactions get only one point for the criterion. All of the other 75 reactions receive 5 points for the evaluation.
Table 8 IDs of technically unavailable products
2; 4–6; 8–9; 11–15; 20; 22; 28; 33; 37; 38; 42; 43; 58; 59; 66; 67; 74 |
4.2.4 Relative added value.
The relative added value for the fine chemicals according eqn (2) and illustrated in Fig. 6 (see Appendix) was calculated under the assumption that CO2 as a feedstock is free of charge. This assumption is justified because the low CO2 price does not significantly influence the relative added value to the bulk chemicals in Section 4.1.3. The relative added value for 41 of the CO2 utilization reactions is over 500% and, for 27, over 1000%. The fine chemicals which belong to the substance group of lactones (ProdCom No. 2110311) achieve almost all of the highest values of the 100 fine chemicals.
4.2.5 Scientific relevance.
The selection criterion of “scientific relevance” was determined by the number of references relating to the product in the SCI-Finder database. Fig. 7 shows that of the 100 fine chemicals considered, the number of references vary between one (e.g., 5-methyl-2-(1-methylethylidene)-4,6-heptadienoic acid) and 12
293 (e.g., isocyanatobenzene). In general, the 10 considered isocyanate have a high number of references, with more than one quarter of the fine chemicals having over 500 references. Similarly to the other selection criteria, the value range for the award of points is shown in the figure.
4.2.6 Ranking of fine chemical synthesis.
The addition of the points from Sections 4.2.1–4.2.6 lead to the ranking list shown in Table 9. Only the synthesis to methylurethane achieves the maximum number of 17.5 points and completely fulfils all of the selection criteria. The CO2 utilization reactions to 3-oxo-pentanedioic acid, 2-imidazolidinone and ethylurthane almost achieve the maximal score, with 17.25 points.
Table 9 Ranking list of the 100 fine chemicals after accumulation of the points of the selection criteria from Sections 4.2.1–4.2.3
ID |
Points |
ID |
Points |
ID |
Points |
86 |
17.5 |
87 |
14.75 |
12 |
11 |
10 |
17.25 |
92 |
14.75 |
27 |
11 |
70 |
17.25 |
44 |
14.25 |
40 |
11 |
85 |
17.25 |
1 |
14 |
41 |
11 |
80/84 |
16.75 |
50 |
14 |
25 |
10 |
94 |
16.75 |
52 |
14 |
26 |
10 |
95 |
16.75 |
56 |
14 |
82 |
9.5 |
99 |
16.75 |
68 |
14 |
90 |
9.5 |
55 |
16.5 |
81 |
14 |
69 |
9.25 |
96 |
16.5 |
88 |
14 |
11 |
9 |
47 |
16.25 |
77 |
13.75 |
15 |
9 |
30 |
16 |
60 |
13.5 |
59 |
8.25 |
39 |
16 |
64 |
13.5 |
9 |
8 |
48 |
16 |
31 |
13 |
14 |
8 |
51 |
16 |
35 |
13 |
20 |
8 |
53 |
16 |
36 |
13 |
37 |
8 |
72 |
15.75 |
89 |
13 |
65 |
7.5 |
79 |
15.75 |
24 |
12.75 |
66 |
7.5 |
93 |
15.75 |
76 |
12.75 |
8 |
7.25 |
97 |
15.75 |
78 |
12.75 |
74 |
7.25 |
17 |
15.5 |
16 |
12.5 |
2 |
7 |
98 |
15.5 |
73 |
12.25 |
5 |
7 |
100 |
15.5 |
19 |
12 |
6 |
7 |
18 |
15 |
21 |
12 |
22 |
7 |
29 |
15 |
23 |
12 |
38 |
7 |
34 |
15 |
32 |
12 |
67 |
6.25 |
49 |
15 |
57 |
12 |
42 |
6 |
54 |
15 |
75 |
12 |
43 |
6 |
91 |
15 |
61 |
11.5 |
58 |
6 |
62 |
14.75 |
45 |
11.25 |
28 |
5 |
63 |
14.75 |
46 |
11.25 |
|
|
71 |
14.75 |
3 |
11 |
|
|
83 |
14.75 |
7 |
11 |
|
|
5. Conclusions
The utilization of captured CO2 as a feedstock in the chemical industry for the synthesis of organic products offers an opportunity to mitigate CO2 emissions and increase independence from fossil fuels. In the scientific literature, numerous reactions are described that use CO2 as a feedstock to synthesize various chemical products, but most of these publications have focused on the feasibility of the syntheses without considering the CO2 reduction potential or potential profitability of these reactions. The present study pre-evaluated 23 reactions to bulk and 100 reactions to fine chemicals that use CO2 as feedstock, relating to the CO2 reduction and economic potential with specially developed selection criteria. The aim was to identify the most suitable products for further, detailed analysis within a reasonable period. Of the examined bulk chemicals, formic acid, oxalic acid, formaldehyde, methanol, urea and dimethyl ether and, of the fine chemicals, methylurethane, 3-oxo-pentanedioic acid, 2-imidazolidinone, ethylurethane, 2-oxazolidone and isopropyl isocyanate, mostly fulfil the selection criteria in each category. Therefore, these reactions represent useful CO2 utilization options with high potential for future technical, ecological and economic analysis that compares their viability to conventional processes.
In addition to the pre-evaluation of the CO2 utilization reactions, some conclusions can be drawn: Amongst the top six bulk chemicals, there are five for which hydrogen is the only reactant of CO2. The advantage of these hydrogenation reactions is that a large amount of CO2 is consumed and the resulting products are simple molecules with a high production volume. For these products, a complete synthesis without fossil feedstock is possible, which would increase their manufacturers’ degree of independence from fossil sources. Through the synthesis of oxalic acid, formaldehyde, methanol, urea and dimethyl ether, some of the oxygen atoms of the CO2 react with water, increasing the consumption of CO2 in the reactions. As a result of the conversion of oxygen into water, these reactions are highly exothermic and in most cases exergonic. At the same time though, the generation of water is the main disadvantage undermining the economic feasibility of the syntheses, as it leads to negative relative added value. The prior highly energy- and cost-intensively-produced hydrogen by electrolysis – preferably operated with renewable power – further reacts to the starting material, water. Future developments and cost reductions in the production of renewable hydrogen could lead to decreasing hydrogen prices and therefore to the economical utilization of CO2 as a feedstock for these products. In the event that hydrogen is produced by steam reforming, the manufacturing costs are significantly lower, but then there is no potential to effectively reduce CO2 emissions through the utilization of CO2 as a feedstock. If CO2 is used as feedstock, the bulk chemicals considered, other than urea, which is already exclusively produced using CO2, have the potential to avoid the emission of ∼20 million tons of CO2 per year if all conventional processes in the EU were to be substituted. This corresponds to ∼0.43% of the EU's greenhouse gas emissions. Therefore, the synthesis of bulk chemicals could be a suitable additional route for the avoidance of CO2 emissions alongside other, already existing options, even if the possible quantities are, at this time, limited. To avoid the emission of larger quantities of CO2 through CO2 re-utilization, the synthesis of fuels or power-to-gas technologies, for example, must also be taken into account.
Of the fine chemicals, four syntheses fulfil or nearly fulfil the maximum of all of the selection criteria. In general, the advantage of these reactions is that in most cases, there is high added value. However, the potential of CO2 consumption is more limited than for the bulk chemicals and distributed over many individual reactions. The CO2 avoidance potential is, for all substances, significantly lower than 1.3 million tons per year, which corresponds to ∼0.029% of EU greenhouse gas emissions. Therefore, the utilization of CO2 as a feedstock for fine chemicals is not a CO2 avoidance option for large amounts of CO2. Nonetheless, as a basis for the highly achievable relative added value, the synthesis of fine chemicals can be an economical option for reducing small amounts of CO2, for instance if a CO2 source is under the control of a manufacturer that already produces the necessary reactants for one of the syntheses.
Appendix
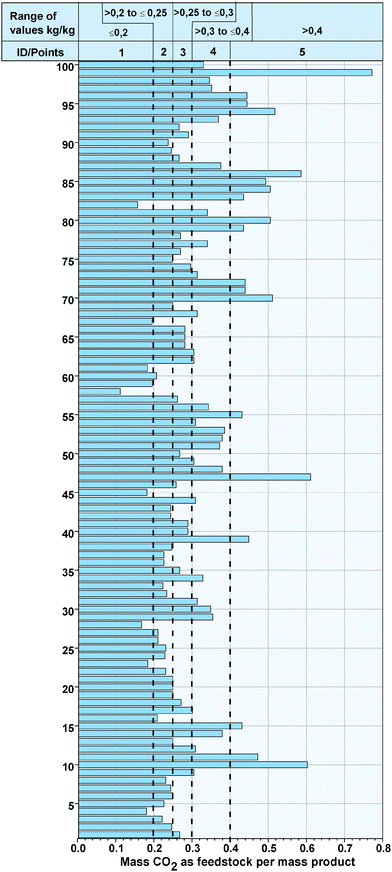 |
| Fig. 5 Specific mass of CO2 as a feedstock for the syntheses of the fine chemicals and value range of the points. | |
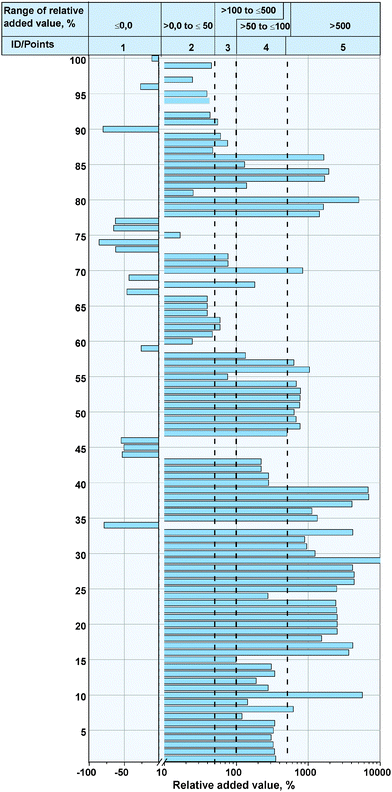 |
| Fig. 6 Relative added value by the synthesis of the fine chemicals. | |
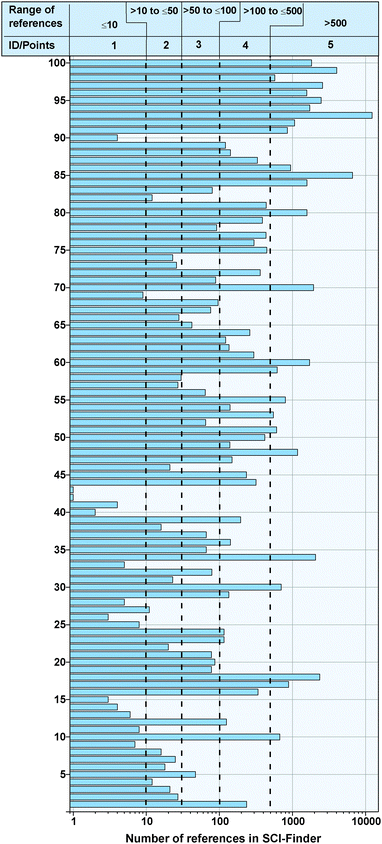 |
| Fig. 7 Number of references of the fine chemicals in the online database SCI-Finder. | |
References
-
A. Otto, Chemische, verfahrenstechnische und ökonomische Bewertung von Kohlendioxid als Rohstoff in der chemischen Industrie, Forschungszentrum Jülich GmbH, Energie & Umwelt, 2015, vol. 268 Search PubMed
.
-
J. H. Meessen, Ullmann's Encyclopedia of Industrial Chemistry, Wiley-VCH Verlag GmbH & Co. KGaA, 2000 DOI:10.1002/14356007.a27_333.pub2
.
- M. Aresta and A. Dibenedetto, Dalton Trans., 2007, 2975–2992 RSC
.
- E. Quadrelli, G. Centi and J.-L. Dulpan, ChemSusChem, 2011, 4, 1194–1215 CrossRef CAS PubMed
.
- M. R. Kember, A. Buchard and C. K. Williams, Chem. Commun., 2011, 47, 141–163 RSC
.
- AsahiKasai Chemicals Corporation, http://www.asahi-kasei.co.jp/chemicals/en/license/page01.html, 2013.
- C. Federsel, R. Jackstell and M. Beller, Angew. Chem., Int. Ed., 2010, 49, 6254–6257 CrossRef CAS PubMed
.
- International Energy Agency, http://www.iea.org/newsroomandevents/news/2015/march/global-energy-related-emissions-of-carbon-dioxide-stalled-in-2014.html, 2015.
- World Energy Outlook 2012, International Energy Agency, Paris, 2012.
- P. Markewitz, W. Kuckshinrichs, W. Leitner, J. Linssen, P. Zapp, R. Bongartz, A. Schreiber and T. E. Muller, Energy Environ. Sci., 2012, 5, 7281–7305 CAS
.
- A. Goeppert, M. Czaun, G. K. Surya Prakash and G. A. Olah, Energy Environ. Sci., 2012, 5, 7833–7853 CAS
.
- M. E. Boot-Handford, J. C. Abanades, E. J. Anthony, M. J. Blunt, S. Brandani, N. Mac Dowell, J. R. Fernandez, M.-C. Ferrari, R. Gross, J. P. Hallett, R. S. Haszeldine, P. Heptonstall, A. Lyngfelt, Z. Makuch, E. Mangano, R. T. J. Porter, M. Pourkashanian, G. T. Rochelle, N. Shah, J. G. Yao and P. S. Fennell, Energy Environ. Sci., 2014, 7, 130–189 CAS
.
-
Efficient Carbon Capture for Coal Power Plants, ed. D. Stolten and V. Scherer, Wiley-VCH, Weinheim, 2011 Search PubMed
.
-
B. Metz, O. Davidson, H. D. Coninck, M. Loos and L. Meyer, IPCC Special Report on Carbon Dioxide Capture and Storage, Intergovernmental Panel on Climate Change, 2007.
-
F. Ausfelder and A. Bazzanella, Diskussionspapier Verwertung und Speicherung von CO2, DECHEMA e.V., 2008.
- S. Schiebahn, T. Grube, M. Robinius, V. Tietze, B. Kumar and D. Stolten, Int. J. Hydrogen Energy, 2015, 40, 4285–4294 CrossRef CAS PubMed
.
- I. Dimitriou, P. Garcia-Gutierrez, R. H. Elder, R. M. Cuellar-Franca, A. Azapagic and R. W. K. Allen, Energy Environ. Sci., 2015, 8, 1775–1789 CAS
.
-
M. Aresta, Carbon Dioxide as Chemical Feedstock, Wiley-VCH, Weinheim, 2010 Search PubMed
.
- X. Xiadoing and J. A. Moulijn, Energy Fuels, 1996, 10, 305–325 CrossRef
.
- P. Braunstein and D. Matt, Chem. Rev., 1988, 88, 747–764 CrossRef CAS
.
- M. Mikkelsen, M. Jorgensen and F. C. Krebs, Energy Environ. Sci., 2010, 3, 43–81 CAS
.
- Chemical Abstracts Service: SciFinder, http://www.cas.org/products/scifinder, 2013.
- BIONITY.COM, http://www.bionity.com/de/news/136646/wichtige-grund-oder-bulkchemikalien-kuenftig-durch-fermentation-von-zucker-und-synthesegas.html, 2012.
- Competitiveness of the European Chemical Industry, http://www.cefic.org/Documents/PolicyCentre/Competitiveness/Competitiveness-of-the-European-chemical-industry-2014.pdf, 2015.
-
S. Inoue and N. Yamazaki, Organic and Bio-organic Chemistry of Carbon Dioxide, Kodansha Scientific Books, Japan, 1982 Search PubMed
.
- A. Correa and R. Martín, Angew. Chem., Int. Ed., 2009, 48, 6201–6204 CrossRef CAS PubMed
.
- S. M. Pillai and R. Ohnishi, React. Kinet. Catal. Lett., 1992, 48, 201–208 CrossRef CAS
.
- S. K. Hoekman, A. Broch, C. Robbins and R. Purcell, Int. J. Greenhouse Gas Control, 2010, 4, 44–50 CrossRef CAS PubMed
.
- M. North, R. Pasquale and C. Young, Green Chem., 2010, 12, 1514–1539 RSC
.
- K. Tomishige, T. Sakaihori, Y. Ikeda and K. Fujimoto, Catal. Lett., 1999, 58, 225–229 CrossRef CAS
.
- X. C. Guo, Z. F. Qin, G. F. Wang and J. G. Wang, Chin. Chem. Lett., 2008, 19, 249–252 CrossRef CAS PubMed
.
- M. Aresta, A. Dibenedetto and E. Fracchiolla, J. Org. Chem., 2005, 70, 6177–6186 CrossRef CAS PubMed
.
- Y. Yamazaki and K. Kakuma, Tetrahedron Lett., 2010, 66, 9675–9680 CrossRef CAS PubMed
.
- N. Yamazaki and F. Higashi, Tetrahedron Lett., 1974, 13, 1191–1194 CrossRef
.
- C. Wu, H. Cheng, R. Liu and The Royal Society of Chemistry, Green Chem., 2010, 12, 1811–1816 RSC
.
- C. J. Dinsmore and S. P. Mercer, Org. Lett., 2004, 6, 2885–2888 CrossRef CAS PubMed
.
- M. Kodaka and T. Tomohiro, J. Chem. Soc., Chem. Commun., 1993, 1, 81–82 RSC
.
- R. Juarez, P. Concepcion and A. Corma, Chem. Commun., 2010, 46, 4181–4183 RSC
.
- C. Phung, R. M. Ulrich and M. Ibrahim, Green Chem., 2011, 13, 3224–3229 RSC
.
- M. Honda, S. Sonehara, H. Yasuda and Y. Nakagawaa, Green Chem., 2011, 13, 3406–3413 RSC
.
- Europäische Kommission: Eurostat – Produktionsstatistiken (Prodcom) Prodcom – Produktionsstatistiken. http://epp.eurostat.ec.europa.eu/portal/page/portal/prodcom/introduction, 2011.
- W. Leitner, Angew. Chem., Int. Ed. Engl., 1995, 34, 2207–2221 CrossRef CAS PubMed
.
- M. W. Farlow and H. Adkins, J. Am. Chem. Soc., 1935, 57, 2222–2223 CrossRef CAS
.
-
A. Behr and K. Nowakowski, in Advances in Inorganic Chemistry, ed. A. Michele and E. Rudi van, Academic Press, 2014, vol. 66, pp. 223–258 Search PubMed.
-
J. J. Anderson, D. J. Dury, J. E. Hamlin and A. G. Kent, England Pat., WO86/02066, 1989 Search PubMed
.
- A. Behr, P. Ebbinghaus and F. Naendrup, Chem. Ing. Tech., 2003, 75, 877–883 CrossRef CAS PubMed
.
- T. Schaub and R. A. Paciello, Angew. Chem., Int. Ed., 2011, 50, 7278–7282 CrossRef CAS PubMed
.
-
T. Schaub, R. Paciello, K. D. Mohl, D. Schneider, M. Schäfer and S. Rittinger, Germany Pat., WO2010/149507 A2, 2010 Search PubMed
.
- D.-K. Lee, D.-S. Kim and S.-W. Kim, Appl. Organomet. Chem., 2001, 15, 148–150 CrossRef CAS
.
- F. Pontzen, W. Liebner, V. Gronemann, M. Rothaemel and B. Ahlers, Catal. Today, 2011, 171, 242–250 CrossRef CAS PubMed
.
- M. Saito, M. Takeuchi, T. Watanabe, J. Toyir, S. Luo and J. Wu, Energy Convers. Manage., 1997, 38, S403–S408 CrossRef CAS
.
- J. Toyir, R. Miloua, N. E. Elkadri, M. Nawdali, H. Toufik, F. Miloua and M. Saito, Phys. Procedia, 2009, 2, 1075–1079 CrossRef CAS PubMed
.
- B. Doss, C. Ramos and S. Atkins, Energy Fuels, 2009, 23, 4647–4650 CrossRef CAS
.
- D. L. Chiavassa, J. Barrandeguy, A. L. Bonivardi and M. A. Baltanás, Catal. Today, 2008, 133–135, 780–786 CrossRef CAS PubMed
.
- M. Sahibzada, Trans. IChemE, 2000, 78, 943–946 CrossRef CAS
.
- A. Goeppert, M. Czaun, J.-P. Jones, G. K. Surya Prakash and G. A. Olah, Chem. Soc. Rev., 2014, 43, 7995–8048 RSC
.
- N. Mimura, I. Takahara, M. Saito, T. Hattori, K. Ohkuma and M. Ando, Catal. Today, 1998, 45, 61–64 CrossRef CAS
.
- J. Fischer, T. Lehmann and E. Heitz, J. Appl. Electrochem., 1981, 11, 743–750 CrossRef CAS
.
- K. Sun, W. Lu, M. Wang and X. Xu, Catal. Commun., 2004, 5, 367–370 CrossRef CAS PubMed
.
- S. Wang, D. Mao, X. Guo, G. Wu and G. Lu, Catal. Commun., 2009, 10, 1367–1370 CrossRef CAS PubMed
.
- F. Zha, J. Ding, Y. Chang, J. Ding, J. Wang and J. Ma, Ind. Eng. Chem. Res., 2011, 51, 345–352 CrossRef
.
- S. Naik, T. Ryu, V. Bui, J. Miller, N. Drinnan and W. Zmierczak, Chem. Eng. J., 2011, 167, 362–368 CrossRef CAS PubMed
.
- M.-H. Zhang, Z.-M. Liu, G.-D. Lin and H.-B. Zhang, Appl. Catal., A, 2013, 451, 28–35 CrossRef CAS PubMed
.
-
W. Kuckshinrichs, P. Markewitz, M. Peters and B. Köhler, Weltweite Innovationen bei der Entwicklung von CCS-Technologien und Möglichkeiten der Nutzung und des Recyclings von CO2, Schriften des Forschungszentrums Jülich Reihe Energie & Umwelt, 2010, vol. 60
.
- S. Huang, B. Yan, S. Wang and X. Ma, Chem. Soc. Rev., 2015, 44, 3079–3116 RSC
.
- O. Arbelaez, A. Orrego, F. Bustamante and A. L. Villa, Top. Catal., 2012, 55, 668–672 CrossRef CAS
.
-
G. Bottaccio, S. Campolmi, and M. G. Felicioli, Italy Pat., US 4132732 A, 1978 Search PubMed
.
- M. North, P. Villuendas and C. Young, Tetrahedron Lett., 2012, 53, 2736–2740 CrossRef CAS PubMed
.
- Y.-M. Shen, W.-L. Duan and M. Shi, Eur. J. Org. Chem., 2004, 3080–3089 CrossRef CAS PubMed
.
- R. L. Paddock, Y. Hiyama and J. M. McKay, Tetrahedron Lett., 2004, 45, 2023–2026 CrossRef CAS PubMed
.
- PRODCOM Liste 2013, Statistische Amt der Europäischen Union, 2013.
- P. D. Re and E. Sandri, Chem. Ber., 1960, 93, 1085–1088 CrossRef PubMed
.
- Y. Inoue, Y. Itoh and H. Hashimoto, Chem. Lett., 1977, 855–856 CrossRef CAS
.
- Y. Inoue, Y. Itoh and H. Hashimoto, Chem. Lett., 1978, 633–634 CrossRef CAS
.
- J. P. Hanrahan and K. J. Ziegler, Langmuir, 2004, 20, 4386–4390 CrossRef CAS
.
- Y. Kishimoto and I. Mitani, Synlett, 2005, 2141–2146 CAS
.
- M. Zhou and Y. Yoshida, Synth. Commun., 1999, 29, 2241–2247 CrossRef CAS PubMed
.
- J. Cervell and J. Narquet, Synth. Commun., 1990, 20, 1931–1941 CrossRef PubMed
.
- C. R. Hauser and T. M. Harris, J. Am. Chem. Soc., 1958, 80, 6360–6363 CrossRef CAS
.
- B. T. Tsuda, R. Sumiya and T. Saegusa, Synth. Commun., 1987, 17, 147–154 CrossRef PubMed
.
- K. Orito and M. Miyazawa, Tetrahedron Lett., 1995, 51, 2489–2496 CrossRef CAS
.
- P. Binger and H.-J. Weintz, Chem. Ber., 1984, 117, 654–665 CrossRef CAS PubMed
.
- H. Hoberg, S. Gross and A. Milchereit, Angew. Chem., Int. Ed. Engl., 1987, 26, 571–572 CrossRef PubMed
.
- H. Hoberg and M. Minato, J. Organomet. Chem., 1991, 406, C25–C28 CrossRef CAS
.
- C. J. Whiteoak and E. Martin, Adv. Synth. Catal., 2012, 354, 469–476 CrossRef CAS PubMed
.
- S. Pulla, P. Ramidi, B. Jarvis, P. Munshi, W. Griffin, J. Darsey, J. Dallas, V. Pokala and A. Ghosh, Greenhouse Gases: Sci. Technol., 2012, 2, 66–74 CrossRef CAS PubMed
.
- Y. Kayaki, M. Yamamoto and T. Ikariya, Angew. Chem., Int. Ed., 2009, 48, 4194–4197 CrossRef CAS PubMed
.
- T. Kimura, K. Kamata and N. Mizuno, Angew. Chem., Int. Ed., 2012, 51, 6700–6703 CrossRef CAS PubMed
.
- H. Ogura and K. Takeda, Communications, 1978, 394–396 CAS
.
- N. Yamazaki, T. Iguchi and F. Higashi, Tetrahedron, 1975, 31, 3031–3034 CrossRef CAS
.
- J. Paz, C. Perez-Balado and B. Iglesias, J. Org. Chem., 2010, 75, 3037–3046 CrossRef CAS PubMed
.
- S. Zheng, F. Li, J. Liua and C. Xiaa, Tetrahedron Lett., 2007, 48, 5883–5886 CrossRef CAS PubMed
.
- J. Li, X. Qi, L. Wang, Y. He and Y. Deng, Catal. Commun., 2011, 12, 1224–1227 CrossRef CAS PubMed
.
- D. Saylik, M. J. Horvath and P. S. Elmes, J. Org. Chem., 1999, 64, 3940–3946 CrossRef CAS
.
- P. Molina and M. Alajarin, Communications, 1882, 596–597 Search PubMed
.
- ECHEA-European Chemicals Agency: Registrierte Stoffe, http://echa.europa.eu/de/, 2012.
- Alibaba Group, http://german.alibaba.com/, 2013.
- Chemical Register, http://www.chemicalregister.com/, 2013.
-
Wasserstoff und Brennstoffzelle-Technologien und Marktperspektiven, ed. J. Töpler and J. Lehmann, Sringer Vieweg, Berlin, Heidelberg, 2014 Search PubMed
.
- boerse.de, http://www.boerse.de/chart/Co2-Emissionsrechte/XC000A0C4KJ2, 2014.
-
M. Appl, Ullmann's Encyclopedia of Industrial Chemistry, Wiley-VCH Verlag GmbH & Co. KGaA, 2000 DOI:10.1002/14356007.a02_143.pub2
.
|
This journal is © The Royal Society of Chemistry 2015 |