DOI:
10.1039/C5DT02974K
(Communication)
Dalton Trans., 2016,
45, 78-84
Enhanced visible and near infrared emissions via Ce3+ to Ln3+ energy transfer in Ln3+-doped CeF3 nanocrystals (Ln = Nd and Sm)†
Received
1st August 2015
, Accepted 16th November 2015
First published on 17th November 2015
Abstract
We report the enhancement of both visible and near infrared (NIR) emissions from Nd3+ ions via Ce3+ sensitization in colloidal nanocrystals for the first time. This is achieved in citrate capped Nd3+-doped CeF3 nanocrystals under ultraviolet (UV) irradiation (λex = 282 nm). The lasing transition (4F3/2 → 4I11/2) at 1064 nm from Nd3+-doped CeF3 nanocrystals has much higher emission intensity via Ce3+ ion sensitization compared to the direct excitation of Nd3+ ions. The nanocrystals were prepared using a simple microwave irradiation route. Moreover, the study has been extended to Sm3+-doped CeF3 nanocrystals which show strong characteristic emissions of Sm3+ ions via energy transfer from Ce3+ ions. The energy transfer mechanism from Ce3+ to Nd3+ and Sm3+ ions is proposed.
1. Introduction
There is a surge in research interest towards developing lanthanide (Ln3+)-doped nanomaterials as they show sharp luminescence signals with longer excited state lifetimes (in the range of μs to ms). In addition, Ln3+ ions show luminescence peaks over a wide electromagnetic spectrum. Particularly interesting are those emitting in the near-infrared (NIR) region i.e. the spectrum with the wavelength range of 700 to 2100 nm. NIR luminescence of lanthanide ions (Ln3+) finds applications in NIR LED technology, lasers, solar energy conversion, medical science and telecommunication.1–4 For example, Ln3+ ions such as Er3+, Nd3+, Tm3+ and Ho3+ have characteristic emissions in the NIR region and can be used as active materials for developing optical amplifiers.5–7 In addition, NIR emission is quite valuable for biological applications because of lower scattering from the body than visible photons, low auto-fluorescence, deep penetration and being transparent to biological tissues.8–11 However, Ln3+ ions in aqueous media show very weak emission intensities owing to their low molar absorption coefficients (2–10 M−1 cm−1), which is attributed to both spin- and parity-forbidden 4f–4f transitions of Ln3+ ions.12–14 In addition, nonradiative transitions are very efficient in aqueous medium leading to further reduction of luminescence efficiency. One way to improve the luminescence efficiency is via an antenna effect, where typically an organic fluorophore possessing a high absorption coefficient is used as a sensitizer for Ln3+ ions. The organic fluorophore transfers the absorbed energy to Ln3+ ions leading to an enhanced luminescence quantum efficiency of Ln3+ ions. However, most of the reports on the antenna effect are restricted to Ln3+ complexes.15,16 Moreover, organic molecules generally photobleach and are relatively less stable. On the other hand, Ce3+ ions can be used as a sensitizer as they possess a high absorbance co-efficient (∼10−18 cm2) due to allowed 4f–5d transitions.17–20 The optical characteristics of Ce3+ ions for efficient energy transfer are mainly attributed to two reasons: broad emission leading to better overlapping with absorption bands of other Ln3+ ions and faster luminescence decay.21–24 However, sensitization of luminescence via Ce3+ ions is mostly restricted to visible emission of Tb3+ ions and to some extent Dy3+ ions.25,26 Recently, we have used Ce3+ ions for sensitizing Tm3+ ions to obtain single band blue emission from NaYF4 nanocrystals.27
Our objective is to sensitize NIR emissions using Ce3+ ions. Among NIR emitting Ln3+ ions, Nd3+ and Sm3+ are interesting for the following reasons. For example, Nd3+ ions show an important laser transition at 1064 nm (e.g. Nd3+-doped Y3Al5O12).28–30 In fact the 1064 nm is used for achieving 532 nm via second harmonic generation. Furthermore, this emission due to the transition from 4F3/2 → 4I11/2 energy levels falls well in the second “human optical window” (1000–1350 nm).31–35 Similarly, Sm3+ ions show two emissions in this optical window (near 1020 and 1150 nm) in addition to a strong emission at 940 nm. Moreover, the emission of Nd3+ shows remarkable thermal sensitivity leading to their use as sub-tissue thermal sensors.36–38 However, both Nd3+ ions and Sm3+ ions show sharp f–f absorption which are difficult to pump.39–41 Although there are quite a few reports on Ce3+ to Nd3+ energy transfer, they are mostly restricted to a glass matrix.42–46 In fact, to our knowledge there are no reports on Ce3+ sensitized NIR emissions from Nd3+ or Sm3+ ions in colloidal nanocrystals, particularly in aqueous milieu.47,48 This is important as there is increasing demand for NIR emitting materials for biological imaging applications. Furthermore, colloidal nanocrystals can easily be coated or incorporated in sol–gel systems which is helpful for thin film device fabrication.
In this article, we report enhanced visible and NIR luminescence from Nd3+-doped CeF3 nanocrystals via Ce3+ sensitization. The lasing transition (4F3/2 → 4I11/2) at 1064 nm from Nd3+-doped CeF3 nanocrystals has shown emission strength about 3 times higher compared to that of the direct excitation of Nd3+ ions. Similarly, strong emissions in the NIR region are observed for Sm3+-doped CeF3 nanocrystals. We emphasize that all these are achieved from water and DMSO dispersible colloidal nanocrystals which can be useful for bioimaging applications.
2. Experimental section
2.1. Materials
Cerium nitrate [Ce(NO3)3, 99.98%], neodymium oxide [Nd2O3, 99.9%], samarium nitrate [Sm(NO3)3, 99.9%], trisodium citrate (>98%), sodium tetrafluoroborate (98%) and absolute ethanol were purchased from Sigma Aldrich. All chemicals were of analytical grade and used without further purification. Double distilled water was used throughout the synthesis and characterization.
2.2. Synthesis
Citrate functionalized Ln3+-doped CeF3 nanocrystals were prepared by a simple microwave assisted method. Briefly, 0.95 mmol of Ce(NO3)3 and 0.05 mmol of Nd(NO3)3 were taken in a 100 ml round bottom flask and completely dissolved in 15 ml of double distilled water. To this clear aqueous solution 1.5 mmol NaBF4 and 4 mmol trisodium citrate (TSC) were added and stirred until complete dissolution. The mixture was magnetically stirred for 15 minutes at room temperature. Finally the mixture was transferred to a 30 ml vial, used for microwave synthesis. The synthesis was carried out using the Anton Parr 300 microwave reactor. The vial was tightly sealed with a Teflon cap and the reaction was carried out at 180 °C for 10 minutes and then cooled to room temperature. The product was collected by centrifugation and washed thrice with absolute ethanol. It should be noted that the microwave experiments were carried out in temperature control mode. Simultaneous gas jet cooling (3–5 bar) during microwave irradiation was performed by using compressed air (6 bar). All microwave experiments were carried out using magnetic stirring at a rate of 600 rpm. The same protocol was used for the synthesis of citrate capped Sm3+-doped CeF3 nanocrystals. [0.97 mmol of Ce(NO3)3 and 0.03 mmol of Sm(NO3)3 in 15 mL double distilled water.]
2.3. Characterization
Powder X-ray diffraction (PXRD) measurements were performed on a Rigaku-smartlab diffractometer with Cu Kα operating at 70 kV and 35 mA at a scanning rate of 1° min−1 in the 2θ range from 20° to 80°. The samples were completely powdered and spread evenly on a quartz slide. TEM measurement was carried out using a high resolution FEG transmission electron microscope (JEOL, JEM 2100F) with a 200 keV electron source. Briefly, a drop of the CeF3 nanocrystals in water was taken on a strong carbon coated 300 mesh Cu grid and dried in air. The FT-IR spectra were obtained with a Perkin Elmer Spectrum RX1 spectrophotometer with the KBr disk technique in the range of 400–4000 cm−1. Thermogravimetric analysis was performed using the Mettler Toledo TGA 851 instrument under a N2 atmosphere at a heating rate of 10° min−1. The photoluminescence measurements were performed with the Horiba Jobin Yvon Fluorolog. All the emission spectra were recorded using the steady state 450 W Xe lamp as the excitation source. The luminescence lifetime measurements were performed using the Horiba Jobin Yvon Fluorolog machine with a pulsed Xe source of 150 W.
3. Results and discussion
3.1. Phase and structure
The phase analysis of the Ln3+-doped CeF3 (Ln = Nd and Sm) nanocrystals was performed using powder X-ray diffraction (XRD) measurements. Fig. 1 shows the XRD pattern of the Nd3+-doped CeF3 nanocrystals along with that of a standard pattern for bulk CeF3. All the peaks are matched well with that of the standard CeF3 (ICSD PDF Card no. 00-038-0452) suggesting the formation of a pure hexagonal phase. The miller indices for each peak are shown above the corresponding peaks.
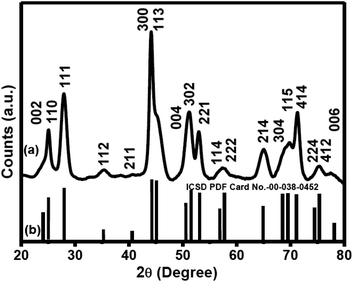 |
| Fig. 1 Powder XRD patterns of (a) Nd3+-doped CeF3 nanocrystals and (b) standard hexagonal CeF3 crystals (ICSD PDF Card No-00-038-0452). | |
The average crystallite size is found to be 8.5 nm as calculated using the Debye–Scherrer equation, t = (0.9λ/β
cos
θ), where t stands for average crystallite size, λ denotes the wavelength (λ = 1.5418 Å) of incident X-ray, β denotes the corrected full width at half maximum (FWHM) and θ denotes the diffraction angle. Lattice parameters of Nd3+-doped CeF3 nanocrystals from XRD are found to be a = b = 7.1024, c = 7.2612 and α = β = 90°, γ = 120°. The hexagonal phase CeF3 nanocrystals adopt the same structure as LaF3, and the space group of these two crystals is P3c1. The highest point group of this structure is D3d. In this structure, the anion ligand number is 12, i.e. each cation is surrounded by 12 anions. The cation sits at the center of an icosahedron. Twelve fluorides are close: four at the bottom corners of the icosahedron, four in the faces of the icosahedron, and four at the top corners of the icosahedron. The cation can be considered as 12 coordinate. The schematic of the unit cell crystal structure of hexagonal CeF3 obtained using the visualization for electronic and structural analysis (VESTA) program is shown in Fig. 2. The atomic coordinates (x, y and z) used for the calculations are used from the reported literature49 and shown in Table S1 (see the ESI†).
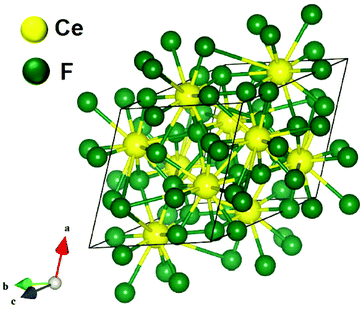 |
| Fig. 2 Schematic presentation of the unit cell structure of CeF3 nanocrystals. | |
3.2. Morphology analysis
The morphology of the citrate-functionalized Nd3+-doped CeF3 nanocrystals is obtained by transmission electron microscopy (TEM) as shown in Fig. 3. From the TEM image the formation of oval shaped nanocrystals is clear. The average aspect ratio of the Nd3+-doped CeF3 nanocrystals is found to be approximately 0.8 (length = 20 nm and breadth = 16 nm). For the semi-quantitative analysis of the elements present in the Nd3+-doped CeF3 nanocrystals energy-dispersive X-ray (EDX) analysis was performed. The EDX analysis spectrum of Nd3+-doped CeF3 nanocrystals are shown in Fig. S1 (see the ESI†). It confirms the presence of Ce, F, and Nd in the sample.
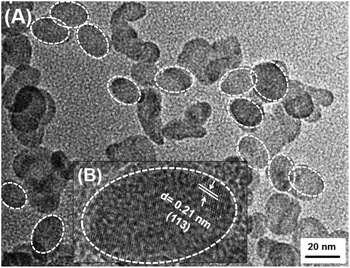 |
| Fig. 3 TEM image of Nd3+-doped CeF3 nanocrystals and the inset shows the HR image of a nanocrystal. White color circles indicate the boundary of the nanocrystals. | |
3.3. Surface functionalization
The high dispersibility of the citrate functionalized CeF3 nanocrystals in water suggests the binding of citrate ions to the surface of the nanocrystals which is supported by the FTIR analysis. The FTIR spectra of citrate capped CeF3 nanocrystals along with pure trisodium citrate molecules are shown in Fig. 4. For the free TSC, major peaks are observed near 3453, 2985, 1600 and 1399 cm−1. The strong and broad stretching vibration band centered at 3448 cm−1 is assigned to O–H in TSC and the peak at 2952 cm−1 is attributed to the methylene (CH2) stretching vibrations of the alkyl chains of TSC. The band at 1600 cm−1 is assigned to the C
O asymmetric stretching vibration of the –COO− group, and the band at 1399 cm−1 is due to symmetric stretching vibrations of C–O in the –COO− group of the TSC.50 In the case of citrate capped Nd3+ doped CeF3 nanocrystals, all the characteristic peaks for TSC molecules are observed, however the C
O stretching frequency is shifted towards a lower wavenumber (at 1590 cm−1) which clearly indicates the binding of the TSC ligand onto the surface of the Nd3+ doped CeF3 nanocrystals.
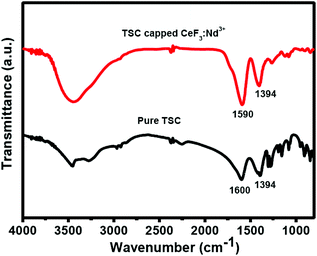 |
| Fig. 4 FTIR spectra of citrate capped Nd3+-doped CeF3 nanocrystals and pure TSC molecules. | |
To further confirm the citrate functionalization onto the surface of CeF3 nanocrystals, TGA analysis was conducted. The TGA curves for both citrate capped CeF3 nanocrystals and pure TSC are shown in Fig. S2, (see the ESI†). For the free TSC the major weight loss is noted from 250 °C due to the decomposition of TSC molecules. For the citrate functionalized CeF3 nanocrystal, the onset of decomposition is shifted to the higher temperature (∼275 °C) which further confirms that the citrate molecules are strongly attached to the surface of the CeF3 nanocrystals. The wt% of citrate molecules attached to the CeF3 is close to 3.12% as calculated from the weight loss from the TGA analysis. Further confirmation for the attachment of the citrate ligands to the nanocrystals comes from the high stability of the nanocrystals in water. The dispersion of the nanocrystals was relatively stable in water for more than 10 hours. The photoluminescence study suggests that the luminescence intensity of Nd3+ ions reduced by only ∼30% after 10 hours. The digital images of the colloidal dispersion along with the corresponding emission spectra are shown in Fig. S3.†
3.4. Optical properties
Photoluminescence (PL) studies have been carried out for the colloidal 0.1 wt(%) Nd3+-doped CeF3 nanocrystals in water. Upon excitation at 282 nm, the nanocrystal dispersion shows visible and NIR emissions as shown in Fig. 5. The prominent band in the visible region is at 693 nm, which is assigned to the 2I15/2 → 4F7/2 transition. In addition, the nanocrystal dispersion shows characteristic NIR bands of Nd3+ ions at 1062 and 1339 nm in water, which are assigned to the 4F3/2 → 4I11/2 and 4F3/2 → 4I13/2 transitions, respectively. The most intense band at 1062 nm is potentially suitable for application in laser emission and telecommunication. The inset of Fig. 5 displays the excitation spectrum which shows an intense broad peak at 282 nm by monitoring the emission at 1062 nm. This peak is due to the 4f5d → 5f electronic transition of Ce3+ ions. This confirms the energy transfer from Ce3+ to Nd3+ ions. To further verify the occurrence of energy transfer, we prepared Nd3+-doped (5 mol%) LaF3 nanocrystals using the same synthesis protocol. Upon excitation at 280 nm, no characteristic emission from Nd3+ ions is observed confirming the energy transfer from Ce3+ to Nd3+ in Nd3+-doped CeF3 nanocrystals (see Fig. S4†). To understand the energy transfer efficiency, PL spectra of Nd3+-doped CeF3 nanocrystals were collected at direct excitation (514 nm) and 282 nm excitation. From Fig. 6, it is clear that upon 282 nm excitation the visible emission and NIR emission intensity increases by two and three times, respectively, compared to that of direct excitation. These results strongly suggest that efficient energy transfer occurs from Ce3+ to Nd3+ ions.
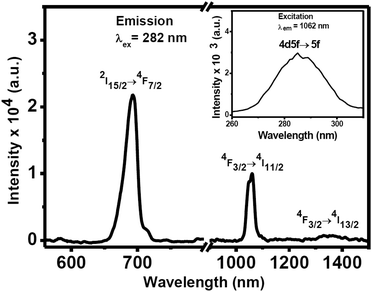 |
| Fig. 5 PL spectrum of 5 mol(%) Nd3+-doped CeF3 nanocrystals in water covering the visible to NIR region (λexi = 282 nm). The inset shows the excitation spectrum for the same nanocrystals (λemi = 1062 nm). | |
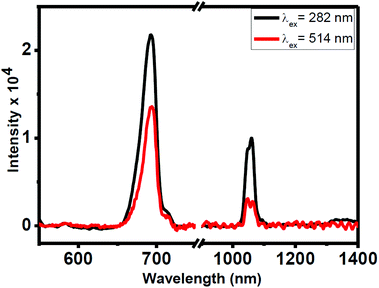 |
| Fig. 6 PL spectra in the visible and NIR regions from Nd3+-doped CeF3 nanocrystals via Ce3+ ion excitation (282 nm) and direct excitation (512 nm). | |
We have extended our study to check whether energy transfer from Ce3+ ions to Sm3+ ions is possible. The 3 mol(%) Sm3+-doped CeF3 nanocrystals were prepared using identical reaction conditions and characterized by XRD analysis. The XRD pattern suggests the formation of pure hexagonal phase CeF3 nanocrystals as shown in Fig. S5.† The PL and excitation spectra of Sm3+ (3%)-doped CeF3 nanocrystals are shown in Fig. 7. Upon 280 nm excitation the dispersion of Sm3+-doped CeF3 nanocrystals in DMSO shows intense peaks at 559, 593, 639 and 702 nm which are assigned to 4G5/2 → 6H5/2, 4G5/2 → 6H7/2, 4G5/2 → 6H9/2 and 4G5/2 → 6H11/2 transitions, respectively. The 4G5/2→6H5/2 transition at 560 nm has a magnetic dipole character.51 The intensity ratio of I(4G5/2 → 6H9/2)/I(4G5/2 → 6H5/2) is 1.05 which indicates lower polarizability of the chemical environment around the Sm3+ ions.52 In addition, the nanocrystals show strong NIR emission peaks at 939 nm, 1017 nm and 1152 nm which are ascribed to the 4G5/2 → 6F5/2, 4G5/2 → 6F7/2, and 4G5/2 → 6F9/2 transitions, respectively. These transitions are characteristics of Sm3+ ions. Among these peaks, 939 peaks are quite intense and they fall in the first “human optical window”. It is quite clear from Fig. S6,† that upon 280 nm excitation, both visible and NIR emission intensities increase by two times compared to that of direct excitation (400 nm). We have performed some control experiments to test the effect of the concentrations of Nd3+ and Ce3+ ions on the emission intensity of the nanocrystals. There is an increase in the emission intensity up to 5 mol% doping for the Nd3+ ions and there upon a decrease is noted. Similarly, the optimum doping concentration for Sm3+-doped CeF3 is found to be 3 mol% (see Fig. S7†).
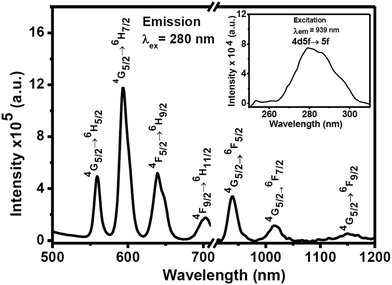 |
| Fig. 7 PL spectrum of 3 mol(%) Sm3+-doped CeF3 nanocrystals in DMSO covering the visible to NIR region (λex = 280 nm). The inset shows the excitation spectrum for the same nanocrystals (λem = 939 nm). | |
Enhancement of visible and NIR emissions for both Nd3+ and Sm3+ ions is achieved via energy transfer from Ce3+ ions. This is supported by the overlap between the emission spectrum of Ce3+ ions with the absorbance of Sm3+ and Nd3+ ions. The results are shown in Fig. S8.† The energy transfer efficiency between the donor (Ce3+) and acceptor (Nd3+/Sm3+) is evaluated using the equation η = 1 − τd/τd0. The average terms τd and τd0 are denoted for the excited state lifetimes of the sensitizer in the presence and absence of the activator, respectively. The lifetimes of the sensitizer Ce3+ ions in the presence and absence of an emitter (i.e. Nd3+/Sm3+ ions) are calculated using Time Correlated Single Photon Counting (TCSPC). The lifetime values of Ce3+ ions in CeF3, CeF3:Nd3+ (5%) and CeF3:Sm3+ (3%) nanocrystals are found to be 23 ns, 9.7 ns and 12 ns, respectively.53 Lifetime decay curves are shown in Fig. S9 (see the ESI†). The calculated energy transfer efficiencies from Ce3+ to Nd3+ and Sm3+ are close to 57% and 48%, respectively. To understand the effect of Nd3+ concentration in CeF3 on the energy transfer efficiency between Ce3+ and Nd3+, we have calculated the corresponding lifetimes of Ce3+ emissions. It is interesting to note that the energy transfer efficiency increases with the increase in the Nd concentration. The values are 53%, 55%, 57% and ∼80% respectively, for 1, 3, 5 and 8 mol% Nd3+ doping in CeF3 nanocrystals. Please note that the optimum luminescence efficiency of Nd3+ is 5 mol%. This suggests that though there is a higher energy transfer probability between Ce3+ to Nd3+ at a higher doping concentration, the cross relaxation between Nd3+–Nd3+ is higher leading to self-quenching of the luminescence. The lifetime decay curves are shown in Fig. S10.† Furthermore, we have measured the lifetimes of Sm3+ and Nd3+ ions in Sm3+ and Nd3+ doped CeF3 nanocrystals, respectively. The lifetime of Sm3+ ions is 741 μs when excited at 282 nm and emission was monitored at 599 nm. The lifetime of Nd3+ ions is 8 μs when excited at 282 nm and emission was monitored at 693 nm as shown in Fig. S11.†
53
3.5. Energy transfer mechanism
The proposed energy transfer mechanism between Ce3+ to Nd3+ ions and Sm3+ ions is schematically shown in Fig. 8. Upon 282 nm excitation, Ce3+ ions get excited from 4f1 to 4f05d1 levels. A radiative energy transfer occurs from the relaxed lowest 4f5d energy level of Ce3+ ions to the 2I15/2 energy level of Nd3+ ions followed by radiative transfer to the low lying 4F7/2 level of Nd3+ ions. Subsequently, energy transfer from 4F7/2 to 4F3/2 occurs nonradiatively. Radiative relaxation from 4F3/2 levels to the 4I11/2 and 4I13/2 energy levels of Nd3+ ions, lead to the 1064 nm and 1339 nm NIR emissions, respectively. Similarly, for Sm3+-doped CeF3 nanocrystals, upon 280 nm excitation, Ce3+ ions are excited from the 4f05d1 → 4f1 level. A radiative energy transfer occurs from the lowest 4f5d energy band of Ce3+ ions to the 4D1/2 energy level of Sm3+ ions followed by nonradiative transfer to the low lying 4G5/2 level of Sm3+ ions. Subsequent decays from 4G5/2 levels and 6F9/2 levels to the 6H5/2, 6H7/2, 6H9/2, 6H11/2, 6F5/2, 6F7/2 and 6F9/2 Sm3+ ions, result in emissions in the visible and NIR regions.
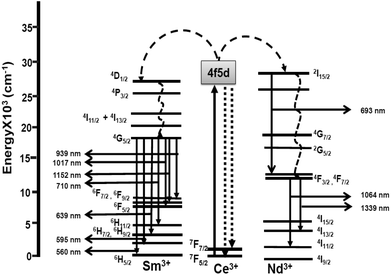 |
| Fig. 8 Schematic of the proposed energy transfer mechanism from Ce3+ ions to Nd3+ and Sm3+ ions. | |
4. Conclusions
In conclusion, we have successfully synthesized hexagonal phase Ln3+ doped CeF3 nanocrystals via a microwave assisted method. The morphology of the nanocrystals was oval shaped with an average aspect ratio of ∼0.8. The nanocrystals are functionalized with citrate molecules which render them highly water dispersible. In Nd3+ and Sm3+-doped CeF3 nanocrystals, enhanced visible and near infrared (NIR) emissions were observed via Ce3+ ion sensitization upon ultraviolet (UV) irradiation (λexi = 280 nm). The energy transfer mechanism from Ce3+ to Nd3+ and Sm3+ ions is proposed. The strong NIR emissions from water dispersible nanocrystals are advantageous for bioimaging applications. In addition, they can easily be incorporated into polymers and other sol–gel matrices for the fabrication of devices for telecommunications.
Acknowledgements
VM thanks the Council of Scientific and Industrial Research (CSIR), Department of Science and Technology (DST), India and IISER-Kolkata for funding. TS, SS and VNKBA thank University Grand Commission (UGC), India for the scholarship.
Notes and references
- S. Mishra, E. Jeanneau, A.-L. Bulin, G. Ledoux, B. Jouguet, D. Amans, A. Belsky, S. Danieleb and C. Dujardin, Dalton Trans., 2013, 42, 12633 RSC.
- S. K. Singh, RSC Adv., 2014, 4, 58674 RSC.
- M. Balestrieri, S. Colis, M. Gallart, G. Ferblantier, D. Muller, P. Gilliot, P. Bazylewski, G. S. Chang, A. Slaouib and A. Diniaa, J. Mater. Chem. C, 2014, 2, 9182 RSC.
-
(a) P. Liu, J. Liu, X. Zheng, H. Luo, X. Li, Z. Yao, X. Yu, X. Shi, B. Hou and Y. Xia, J. Mater. Chem. C, 2014, 2, 5769 RSC;
(b) C. Hazra, T. Samanta, A. V. Asaithambi and V. Mahalingam, Dalton Trans., 2014, 43, 6623 RSC.
- G. Gao, M. Peng and L. Wondraczek, J. Mater. Chem. C, 2014, 2, 8083 RSC.
- Z.-P. Zheng, X.-X. Zhang, T. Li, J. Yang, L.-M. Wei, L.-G. Zhang, X.-M. Lina and Y.-P. Cai, Dalton Trans., 2014, 43, 14009 RSC.
- J. Zhang and S. Petoud, Chem. – Eur. J., 2008, 14, 1264 CrossRef CAS PubMed.
- D. Wang, B. Xue, X. Kong, L. Tu, X. Liu, Y. Zhang, Y. Chang, Y. Luo, H. Zhaoc and H. Zhang, Nanoscale, 2015, 7, 190 RSC.
- D. Tu, L. Liu, Q. Ju, Y. Liu, H. Zhu, R. Li and X. Chen, Angew. Chem., Int. Ed., 2011, 50, 6306 CrossRef CAS PubMed.
- E. Hemmer, N. Venkatachalam, H. Hyodo, A. Hattori, Y. Ebina, H. Kishimoto and K. Soga, Nanoscale, 2013, 5, 11339 RSC.
-
(a) A. F. Collet, K. A. Gogick, K. A. White, S. Villette, A. Pallier, G. Collet, C. Kieda, T. Lib, S. J. Geib, N. L. Rosi and S. Petoud, Proc. Natl. Acad. Sci. U. S. A., 2013, 113, 17199 CrossRef PubMed;
(b) M. Pedroni, F. Piccinelli, T. Passuello, M. Giarola, G. Mariotto, S. Polizzi, M. Bettinellia and A. Speghini, Nanoscale, 2011, 3, 1456 RSC.
- Y. Liu, D. Tu, H. Zhu, R. Li, W. Luo and X. Chen, Adv. Mater., 2010, 22, 3266 CrossRef CAS PubMed.
- L. Li, Y. Su and G. Li, J. Mater. Chem., 2010, 20, 459 RSC.
- Z. Zhang, H. Feng, L. Liu, C. Yu, X. Lü, X. Zhu, W.-K. Wong, R. A. Jones, M. Pand and C. Su, Dalton Trans., 2015, 44, 6229 RSC.
- J.-C. G. Bünzli and C. Piguet, Chem. Soc. Rev., 2005, 34, 1048 RSC.
-
(a) O. A. Blackburn, M. Tropiano, T. J. Sørensen, J. Thom, A. Beeby, L. M. Bushby, D. Parker, L. S. Natrajan and S. Faulkner, Phys. Chem. Chem. Phys., 2012, 14, 13378 RSC;
(b) T. Samanta, C. Hazra and V. Mahalingam, New J. Chem., 2015, 39, 106 RSC.
- S. Wu, C. Li, W. Wei, H. Wang, Y. Song, Y. Zhu and L. Lu, J. Rare Earths, 2010, 28, 171 CrossRef.
- Z. Fang, R. Cao, F. Zhang, Z. Ma, G. Donga and J. Qiu, J. Mater. Chem. C, 2014, 2, 2204 RSC.
- B. Gao, Q. Yan, Y. Tong, X. Zhang, H. Ma b, J.-l. Adam, J. Ren and G. Chen, J. Lumin., 2013, 143, 181 CrossRef CAS.
- D. Chen, Y. Wang, Y. Yu, P. Huang and F. Weng, J. Appl. Phys., 2008, 104, 116105 CrossRef.
- M. C. Tan, G. A. Kumar and R. E. Riman, Opt. Express, 2009, 17, 15904 CrossRef CAS PubMed.
- Y. V. Orlovskii, T. T. Basiev, E. O. Orlovskaya, Y. S. Privisa, V. V. Fedorov and S. B. Mirov, J. Lumin., 2003, 101, 211 CrossRef CAS.
- J. Mares, B. Jacquier, C. Pédrini and G. Boulon, Rev. Phys. Appl., 1987, 22, 145 CrossRef CAS.
- J. Ueda, P. Dorenbos, A. J. J. Bos, K. Kuroishia and S. Tanabea, J. Mater. Chem. C, 2015, 3, 5642 RSC.
- K. Li, M. Shang, D. Geng, H. Lian, Y. Zhang, J. Fan and J. Lin, Inorg. Chem., 2014, 53, 6743 CrossRef CAS PubMed.
- G. V. L. Reddy, L. R. Moorthy, T. Chengaiah and B. C. Jamalaiah, Adv. Mater. Lett., 2013, 4, 841 Search PubMed.
- V. N. K. B. Adusumalli, S. Sarkar and V. Mahalingam, ChemPhysChem, 2015, 16, 2313 CrossRef PubMed.
- L. Tong, J. Lou, Y. Xu, Q. Luo, N. Shen and E. Mazur, Appl. Opt., 2002, 41, 4008 CrossRef CAS PubMed.
- S. Satapathy, A. Ahlawat, A. Paliwal, R. Singh, M. K. Singh and P. K. Gupta, CrystEngComm, 2014, 16, 2723 RSC.
- P. Samuel, G. A. Kumar, T. Yanagitani, H. Yagi, K. I. Ueda and S. M. Babu, Opt. Mater., 2011, 34, 303 CrossRef CAS.
- A. M. Smith, M. C. Mancini and S. Nie, Nat. Nanotechnol., 2009, 4, 710 CrossRef CAS PubMed.
-
(a) R. Wang and F. Zhang, J. Mater. Chem. B, 2014, 2, 2422 RSC;
(b) N. Bogdan, F. Vetrone, G. A. Ozin and J. A. Capobianco, Nano Lett., 2011, 11, 835 CrossRef CAS PubMed.
- N. Won, S. Jeong, K. Kim, J. Kwag, J. Park, S. G. Kim and S. Kim, Mol. Imaging, 2012, 11, 338 CAS.
- G. Hong, Y. Zou, A. L. Antaris, S. Diao, D. Wu, K. Cheng, X. Zhang, C. Chen, B. Liu, Y. He, J. Z. Wu, J. Yuan, B. Zhang, Z. Tao, C. Fukunaga and H. Dai, Nat. Commun., 2014, 5, 4206 CAS.
- U. Rocha, K. U. Kumar, C. Jacinto, I. Villa, F. S. Rodríguez, M. d. l. C. I. d. l. Cruz, A. Juarranz, E. Carrasco, F. C. J. M. van Veggel, E. Bovero, J. G. Solé and D. Jaque, Small, 2014, 10, 1141 CrossRef CAS PubMed.
- U. Rocha, C. Jacinto, W. F. Silva, I. Guedes, A. Benayas, L. M. Maestro, M. A. Elias, E. Bovero, F. C. J. M. van Veggel, J. G. Solé and D. Jaque, ACS Nano, 2013, 7, 1188 CrossRef CAS PubMed.
- K. Binnemans, Chem. Rev., 2009, 109, 4283 CrossRef CAS PubMed.
-
(a) A. S. Chauvin, F. Gumy, D. Imbert and J.-C. G. Bünzli, Spectrosc. Lett., 2004, 37, 517 CrossRef CAS;
(b) A. J. Steckl, M. Garter, D. S. Lee, J. Heikenfeld and R. Birkhahn, Appl. Phys. Lett., 1999, 75, 2184 CrossRef CAS.
- J. H. Kim and P. H. Holloway, Adv. Mater., 2005, 17, 91 CrossRef CAS.
- A. Bednarkiewicz, D. Wawrzynczyk, M. Nyk and W. Strek, Opt. Mater., 2011, 33, 1481 CrossRef CAS.
- K. Lunstroot, L. Baeten, P. Nockemann, J. Martens, P. Verlooy, X. Ye, C. G. Walrand, K. Binnemans and K. Driesen, J. Phys. Chem. C, 2009, 113, 13532 CAS.
- S. Moeller, A. Hoffmann, D. Knaut, J. Flottmann and T. Juestel, J. Lumin., 2015, 158, 365 CrossRef CAS.
- B. M. Brewer and M. Nicol, J. Lumin., 1981, 23, 269 CrossRef.
- H. Dong, L.-D. Sun and C.-H. Yan, Chem. Soc. Rev., 2015, 44, 1608 RSC.
- P. Samuel, T. Yanagitani, H. Yagi, H. Nakao, K. I. Ueda and S. M. Babu, J. Alloys Compd., 2010, 507, 475 CrossRef CAS.
- F. N. Sayed, V. Grover, S. V. Godbole and A. K. Tyagi, RSC Adv., 2012, 2, 1161 RSC.
- Q. Xiao, Q. Zhou and M. Li, J. Lumin., 2010, 130, 1092 CrossRef CAS.
- J. L. Ferrari, K. de O. Lima, E. Pecoraro, R. A. S. Ferreira, L. D. Carlos and R. R. Goncalves, J. Mater. Chem., 2012, 22, 9901 RSC.
- Pierre Villars, Material Phases Data System (MPDS), CH-6354 Vitznau, Switzerland; SpringerMaterials; sd_1622044 (Springer-Verlag GmbH, Heidelberg, 2014), http://materials.springer.com/isp/crystallographic/docs/sd_1622044; accessed: 13-06-2015.
- M. Pedroni, F. Piccinelli, T. Passuello, S. Polizzi, J. Ueda, P. Haro-González, L. Martinez Maestro, D. Jaque, J. García-Solé, M. Bettinelli and A. Speghini, Cryst. Growth Des., 2013, 13, 4906 CAS.
-
(a) K. Lunstroot, P. Nockemann, K. Van Hecke, L. Van Meervelt, C. Görller-Walrand, K. Binnemans and K. Driesen, Inorg. Chem., 2009, 48, 3018 CrossRef CAS PubMed;
(b) H. F. Brito, O. L. Malta, M. C. F. C. Felinto, E. E. S. Teotonio, J. F. S. Menezes, C. F. B. Silva, C. S. Tomiyama and C. A. A. Carvalho, J. Alloys Compd., 2002, 344, 293 CrossRef CAS.
- L. Sun, Y. Qiu, T. Liu, H. Peng, W. Deng, Z. Wang and L. Shi, RSC Adv., 2013, 3, 26367 RSC.
- R. L. Nigro, G. Malandrino, I. L. Fragala, M. Bettinelli and A. Speghini, J. Mater. Chem., 2002, 12, 2816 RSC.
Footnote |
† Electronic supplementary information (ESI) available: SEM, EDAX, TGA, PL spectra and digital images of water dispersion of Nd3+ doped CeF3 nanocrystals. Lifetimes, XRD and energy transfer mechanism of Ce3+ to Sm3+ of Sm3+ doped CeF3 nanocrystals. See DOI: 10.1039/c5dt02974k |
|
This journal is © The Royal Society of Chemistry 2016 |