DOI:
10.1039/C4DT03763D
(Perspective)
Dalton Trans., 2015,
44, 7092-7104
Polyoxometalate – conductive polymer composites for energy conversion, energy storage and nanostructured sensors
Received
9th December 2014
, Accepted 6th March 2015
First published on 12th March 2015
Abstract
The exchange of electric charges between a chemical reaction centre and an external electrical circuit is critical for many real-life technologies. This perspective explores the “wiring” of highly redox-active molecular metal oxide anions, so-called polyoxometalates (POMs) to conductive organic polymers (CPs). The major synthetic approaches to these organic–inorganic hybrid materials are reviewed. Typical applications are highlighted, emphasizing the current bottlenecks in materials development. Utilization of the composites in the fields of energy conversion, electrochemical energy storage, sensors and nanoparticle “wiring” into conductive materials are discussed. The outlook section presents the authors’ views on emerging fields of research where the combination of POMs and CPs can be expected to provide novel materials for groundbreaking new technologies. These include light-weight energy storage, high-sensitivity toxin sensors, artificial muscles, photoelectrochemical devices and components for fuel cells.
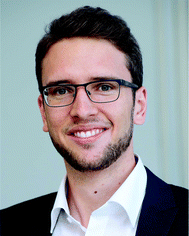 Sven Herrmann | Sven Herrmann studied chemistry at Friedrich-Alexander-University Erlangen-Nuremberg (Germany) and completed his MSc project in 2013 in the group of Carsten Streb. He subsequently followed the Streb group to Ulm University where he is working on his PhD project which is funded through a fellowship of the Fonds der Chemischen Industrie. Sven is interested in the development of polyoxometalate-based ionic liquids (POM-ILs) and their application in materials chemistry, electrochemistry and catalysis. |
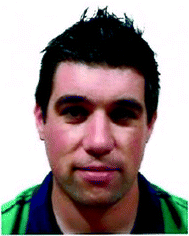 Chris Ritchie | Dr Chris Ritchie completed his undergraduate studies BSc (Hons) Chemistry with Medicinal Chemistry at the University of Glasgow, Scotland. He then commenced his PhD under the supervision of Prof. Lee Cronin in the same department (2005–2008), and subsequently moved to The University of Melbourne, Australia where he was a post-doctoral researcher with Dr Colette Boskovic (2008–2013). In 2013, Dr Ritchie was awarded an Australian Research Council Discovery Early Career Researcher Award (DECRA) and is now a group leader and lecturer at the University of Melbourne. His current research interests include the self-assembly of polyoxometalate based inorganic–organic composites. |
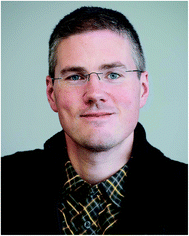 Carsten Streb | Carsten Streb studied chemistry at TU Kaiserslautern (Germany). He then undertook a PhD with Prof. Lee Cronin at the University of Glasgow (Scotland, UK) focusing on inorganic host–guest systems and porous framework materials (POMOFs). Subsequently, he did a postdoc at the University of Glasgow and James Watt Nanofabrication Centre, developing nanostructured semiconductors. In 2009, Carsten started his independent research as a Liebig-Fellow at the Friedrich-Alexander-University Erlangen-Nuremberg (Germany). In 2013 he became Professor of Inorganic Chemistry at Ulm University (Germany). His current research interests are novel synthetic routes to functional molecular metal oxides for sustainable energy conversion and storage. |
1. Introduction
Since the pioneering days of polymer development in the late 18th century, polymeric materials have conquered virtually all areas of life, due to their facile and low cost manufacturing on the megaton-scale. Over time, polymers have progressed from “simple” bulk materials such as rubber or polyethylene to cutting-edge materials where physical and chemical properties can be tuned on the molecular level. Consequently, polymers with stimuli-responsiveness, biocompatibility, antimicrobial and self-repairing properties have been accessed.1
One ground-breaking polymer class with high relevance for applications in electronics and energy systems are conducting polymers (CPs). Their importance was acknowledged when in the year 2000, the Nobel Prize in Chemistry was awarded to Alan J. Heeger, Alan G. MacDiarmid and Hideki Shirakawa “for the discovery and development of conductive polymers”.2
Conducting polymers offer the possibility to introduce organic conductors in low dimensional, flexible and mechanically resistant systems, giving access to materials with unique properties. This has led to a host of new applications such as organic light-emitting diodes (OLEDs),3 chemical- or bio-sensors,4 functional coatings5 and electronic, optoelectronic and energy storage devices6 as well as organic solar cells.7
In the context of this Perspective, we will focus our interest on so-called intrinsically conductive polymers (ICPs)1 where conductivity is based on electron-transfer through the polymers themselves, often enabled by the presence of a fully conjugated π-electron system spanning the length of the polymer backbone. Oxidative (p-type) or reductive (n-type) doping of the polymers allows the tuning of the conductivity from semiconductors to metallic conductors.1 In contrast, extrinsically conductive polymers (ECPs) are based on conductive particles (e.g. metal particles) which are dispersed in a non-conductive polymer matrix.1 These systems will not be considered here. Typical examples of ICPs are shown in Table 1.
Table 1 Overview of prototype ICPs
ICP |
Abbreviation |
Ref.a |
The references given illustrate examples of POM-containing ICPs of the types specified.
|
Polyacetylene |
PAc |
— |
Polypyrrole |
PPy |
8
|
Polyaniline |
PANI |
9
|
Polythiophene |
PT |
10
|
Poly(diphenylamine) |
PDPA |
11
|
Poly(p-phenylene) |
PPP |
12
|
Poly(3,4-ethylenedioxythiophene) |
PEDOT |
13
|
Due to their ability to bind and electronically address redox-active species on the single-molecule level, CPs have been recognized as ideal matrices to embed redox-active molecules and develop flexible, easily processible, conductive materials.14
A particularly promising approach is the introduction of nanoscale reactive inorganic species in CPs so as to harness the combined properties of electric conductivity, materials properties and unique inherent reactivity.15 One outstanding class of tuneable, redox-active inorganic materials are molecular metal oxides, so-called polyoxometalates (POMs) which have been investigated as reactive sites in a large number of CPs, see Table 1.16
Polyoxometalates (POMs) are anionic metal-oxo clusters of the general formula [MxOy]n− consisting of two or more high-valent transition metals (M) (e.g. M = V, Mo, W) which are linked through oxo-ligands. The formation of polyoxometalates follows a unique self-assembly process starting from simple building blocks such as MoO42− or VO43−. Using this approach, clusters with dimensions of ca. 1–6 nm containing up to 368 metal centres are accessible, see Fig. 1.17
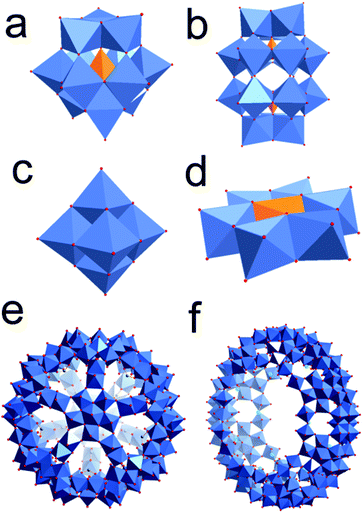 |
| Fig. 1 Polyhedral illustration of archetypal POM anions, highlighting their structural diversity. (a) Keggin anion [XM12O40]n−; (b) Dawson anion [X2M18O62]n−; (c) Lindqvist anion [Mo6O19]2−; (d) Anderson anion [XM6O24]n−. (e) + (f) Polyhedral illustration of two giant POM anions (diameter each ca. 3 nm), (e) {Mo132} ball shaped cluster.18 (f) A {Mo154} wheel structure.19 Colour scheme: Mo (blue); O (red), P (teal), C (green), template e.g. B, Si, P (orange). | |
One striking feature of POMs is their ability to be functionalized by incorporation of virtually any metal ion from the periodic table, giving one of the chemically and structurally most diverse classes of inorganic materials.20 This versatility can be used to form highly redox-active molecular materials which can undergo complex one- and multi-electron transfer, making POMs highly sought-after functional materials for a myriad of applications.17,21
This Perspective will summarize the most outstanding recent accomplishments in polyoxometalate-functionalized conducting polymers and will give an overview of the principal synthetic routes to POM/CP composites and their current applications. The outlook section focuses on the authors’ view of the future perspectives of the field, inspired by recent landmark papers or by concepts which to our mind have not been fully addressed yet.
1.1. POMs as redox-active molecules for electron transfer, catalysis and substrate sensing
Polyoxometalates are known to exhibit unique redox properties and the reversible uptake of up to 24 electrons per cluster unit (here: [PMo12O40]3−) has been reported in the solid state,22 thus demonstrating their potential for multi-electron transfer. Further, the exact electrochemical properties such as redox potentials and number of electrons stored can be fine-tuned by chemical modification of the POM structure, e.g. by incorporation of redox-active metal centres. Metal functionalization can also be used to generate coordinative substrate binding sites so that electrochemical and amperometric sensors can be accessed.17,23
The most common route to this end is the hydrolytic removal of one or several metal sites from (typically tungsten-based) Keggin- or Dawson-anions, leading to so-called lacunary species (e.g. [SiW12O40]4− → [SiW11O39]8− + “WO4+”) where vacant metal binding sites are generated which are available for further metal functionalization.17 As the functionalized clusters are stable at acidic to neutral pH levels, sensors for bio-medicinal applications at physiological conditions can be envisaged (Fig. 2).
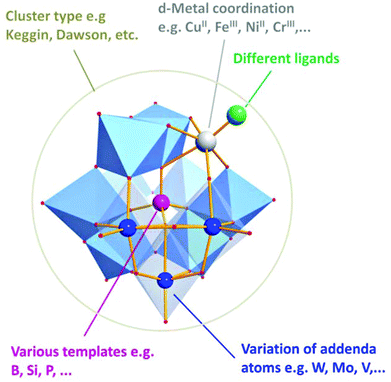 |
| Fig. 2 Components of a POM which are amenable to chemical modification. The cluster anion can be modified by variation of the template, addenda (i.e. framework) metal, heterometal and ligand. The counter-cations (not shown) can be tuned from simple inorganic (e.g. metal) cations to complex organic cations. | |
1.2. Interfacing POMs with electrically conductive substrates, current state-of-the art and challenges
Typically, POM-salts are insulators and only few examples are known where (semi-)conducting properties are observed. This is often achieved by incorporation of organic radical cations which significantly enhance electrical conductivity within the salt.24 Further, in addition to high intrinsic electronic conductivity, ionic conductivity, particularly protonic conductivity is often a desired feature, e.g. for applications in fuel cells and water splitting devices.25 To enable the technological use of POMs in electrical devices, it is critical to design reliable electrical addressability between the POM as the reaction centre and other parts of the device. With the recent advent of nanostructured conductive carbons such as carbon nanotubes (CNTs) and graphene, a host of materials have been developed where POMs were attached to these conductive materials through covalent or non-covalent means.26 The attractiveness of this route lies in the intrinsic 1D or 2D nanostructure of the CNTs and graphene, respectively, which gives access to high surface-area materials with applications in energy storage,22b pollutant sensing27 and electrocatalysis.28
POM/graphene composites have also been recently explored for charge storage as they are easily accessible, e.g. by simply mixing an acetonitrile solution of the tetrabutylammonium salt of the [PMo12O40]3− anion with a toluene solution containing a sample of RGO (reduced graphene oxide) followed by filtration. The cooperative enhancement of the capacitance properties of this material is proposed to be induced by the adsorption of the POMs to its surface. On reduction, each POM accepts up to 24 electrons to yield [PMo12O40]27− with the resulting increase in negative charge being spread onto the RGO, significantly enhancing the electrical double layer capacitance of the RGO. Overall power density of the material at 4 mA is reported as 700 W kg−1 with an improvement in both capacity and charge/discharge rates being realised through synergetic interactions between the POM chemical component due to valence change and the capacitance of the RGO.
An alternative approach is the surface modification of metal oxide semiconductors, e.g. TiO2 with POMs which has been used to access heterogeneous photooxidation catalysts30 and photoanodes in photoelectrochemical cells.29 In one notable example, the authors assembled a photoanode via layer-by-layer assembly of POMs and TiO2 nanoparticles see Fig. 3. The resulting composite showed that the photoelectrochemical performance can be controlled by altering the number of assembled layers. Further, it was found that the type of POM significantly affects the performance characteristics: photoanodes based on the Keggin anion [PW12O40]3− showed significantly higher photocurrents and conversion efficiency compared with photoanodes based on the Dawson anion [P2Mo18O62]6−. The authors tentatively assigned this behaviour to the more facile re-oxidation of the tungstate-based Keggin anion compared with the molybdate-based Dawson, which is suggested to enable more efficient electron shuttling from the TiO2 to the electrode, see Fig. 3.29
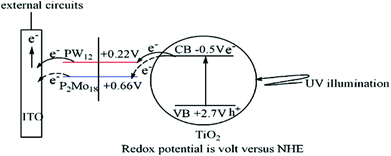 |
| Fig. 3 Illustration of electronic features of the POM/TiO2 photoanode. Note that the different redox-properties of the cluster used (here: [PW12O40]3− and [P2Mo18O62]6− modify the photoelectrochemical properties of the system. Reproduced from ref. 29 with permission of ACS Publishing. | |
1.3. Polyoxometalate-non-conductive polymer composites
This section provides a brief introduction to composite materials where POMs are interfaced with non-conductive polymers to highlight recent developments in this closely related field which has also been reviewed recently.31 Recent reports demonstrate that embedding POMs into polymers can be used to control the nanoscale aggregation behaviour of the materials;32 as well as their thermoresponsive,33 photocatalytic,34 mechanical and electrical properties.35 Thus, a large number of fields of application are accessible.
2. Synthetic access to POM-conductive polymer composites
2.1. Non-covalent functionalization by chemical or electrochemical polymerization
The most widely used approach to POM/CP composites uses non-covalent attachment of the POM anion to CPs, see Table 2. One common route is the use of cationic CPs to electrostatically immobilize the anionic POM clusters. Prime example for this approach is cationic PPy which has been electrostatically functionalized with various POM anions such as Anderson–Evans, Lindqvist, Keggin and Wells–Dawson type clusters (Fig. 1).36 Often, electropolymerization routes are employed where the monomer pyrrole and the POM anion of choice are dissolved in an inert solvent (e.g. acetonitrile) and the pyrrole is oxidatively electropolymerized. The POM/CP film formed on the electrode can be mechanically removed and dried. Electrochemical analysis show that the films combine the redox-properties of the polypyrrole and of the respective cluster anion, making the POM anions electrochemically addressable in a solid polymer matrix.37 When comparing chemical polymerization and electropolymerization, it is found that chemical polymerization often leads to bulk materials, whereas electrochemical polymerization gives facile access to CP films on electrode surfaces.31b
Table 2 Overview of prototype POM/CP composites synthesized chemically (a) or electrochemically (b). Possible applications are cited according to the original work (see references)
POM |
CP |
Support |
Application |
Ref. |
Chemical polymerization.
Electrochemical polymerization.
PPE = (poly phenylene-ethynylene).
|
[PMo12O40]3− (= PW12)a |
Ppy |
Carbon paper |
Capacitors, electrocatalysis |
13
|
[PW12O40]3− (= PW12)b |
PEDOT |
Carbon paper |
Capacitors, electrocatalysis |
13
|
[SiCr(H2O)W11O39]5− b |
PPy |
Pt |
Heterogeneous catalysis, electrodes, sensors |
45
|
[PW12O40]3− b |
PPy |
Pt |
— |
46
|
[SiW12O40]4−; [P2W18O62]6− b |
PPy |
Pt |
Charge storage |
47
|
V2O5 a |
PANI |
— |
Sensing, LEDs |
48
|
[SiW12O40]4−; [PMo12O40]3−; [PW12O40]3− b |
PT |
— |
— |
10
|
[Mo6O18NR]2− a |
PPEc |
— |
Vesicle formation |
43
|
[P2W17O61M]n− b M = CuII, CoII, FeIII |
PPy |
GC |
H2O2-sensing |
49
|
POM incorporation into the CP matrix can be achieved by two general approaches: under pre-polymerization conditions, the POM is combined with the monomer before polymerization occurs.13 In contrast, under post-polymerization conditions,16 the polymer is formed first and only afterwards functionalized by the POM cluster. The exact choice of approach depends on considerations regarding synthesis, stability and reactivity.
In order to immobilize the POM anion within the CP matrix so as to prevent leaching, ideally, cationic polymers such as PPy (see also Table 1) are used and have shown high POM loadings with negligible leaching into solution.16 Interestingly, Sung et al. demonstrated that POM/CP composites remain stable upon redox switching, even if the cationic CP (PPy) was significantly reduced, and no leaching of the incorporated POMs ([SiW12O40]4− and [P2W18O62]6−, respectively) was observed.38
The effects of bulk vs. electro-polymerization on the morphology and properties of POM/CP composites were recently demonstrated by Freund et al. The authors used an evaporation-driven chemical polymerization to deposit polypyrrole-POM composites (here: H3PMo12O40, abbreviated as PMo12) on carbon paper, giving even thin films where the composite fully penetrated the carbon matrix.39 In contrast, when anodic electro-polymerization of 3,4-ethylene–dioxythiophene (EDOT)–phosphotungstic acid (H3PW12O40, abbreviated as PW12) mixtures in acetonitrile was investigated, films based on nodular structures of PEDOT/PW12 were grown on the surface of the carbon paper only and no penetration of the carbon paper matrix was observed (Fig. 4). Coulometric control was used to ensure equal deposition quantities of both the PPy/PMo12 and the PEDOT/PW12 composite.
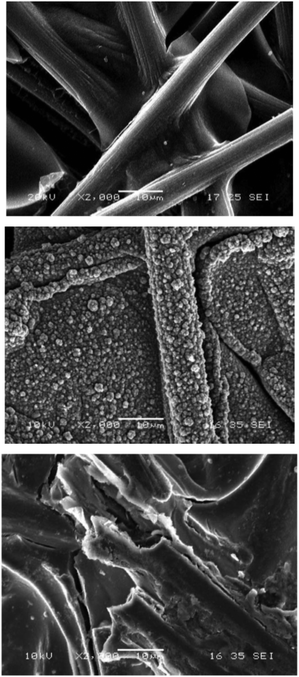 |
| Fig. 4 Scanning electron micrographs of deposited POM/CPs. Top: untreated Spectracarb 2050-A carbon paper. Centre: PEDOT/PW12 grown electrochemically on carbon paper, showing nodular structures. Bottom: PPy/PMo12 grown chemically on carbon paper, showing sheet-like structures. Reproduced from ref. 39 with permission of The Electrochemical Society. | |
The authors expanded their studies and illustrated that evaporation-driven polymerization40 is a facile means to deposit thin films where the film thickness can be tuned between 40–100 nm and high electric conductivities of 15–30 S cm−1 were found. The results suggest that evaporation-driven polymerization could be developed into a general process to coat substrates with thin films of conductive POM/CP composites, thus significantly widening their application range.
McCormac et al. provided further insight into electropolymerization and demonstrated the need for a detailed understanding of the underlying electrochemistry of the compounds: the group investigated the electrochemical immobilization of a series of Dawson-type POMs into various CPs41 and it was noted that the potential cycling range has a dramatic effect on the stability of the composites: stable composites were only obtained when the first and second tungsten-based redox waves were cycled, while other cycling modes led to unsatisfactory materials.
2.2. Covalent functionalization routes
The covalent attachment of POMs to CPs can be achieved by (1) side-chain incorporation, where the POM anions are chemically linked to functionalized polymer side-chains; (2) backbone incorporation where the POM is directly embedded into the main polymer chain. To-date, side-chain incorporation is the more frequently used approach, while backbone incorporation is extremely rare, mainly due to the synthetic challenges of the latter technique. One prerequisite for both approaches is access to organically functionalized POMs which allow the covalent attachment to the polymer. Typical examples of POM organo-functionalization routes have recently been reviewed and often involve the use of organically modified silane, phosphonate, alkoxide, organotin and imine moieties as anchoring sites on the cluster, see Fig. 5.42
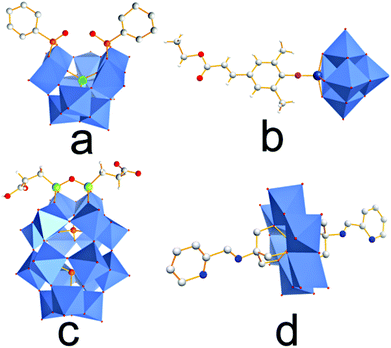 |
| Fig. 5 Organo-functionalization of POMs: (a) dilacunary Keggin POM organically functionalized with two phosphonates (P in orange); (b) Lindqvist anion functionalized with an organic imine (N in pink); (c) Dawson cluster functionalized via two silane anchoring sites (Si in green); (d) Mn-templated Anderson cluster with two trisalkoxide linkers (N in blue). Colour scheme: Cluster addenda atoms Mo/W (blue polyhedra), O (red), C (grey). | |
2.2.1. Side-chain incorporation.
As described above, side-chain modification is currently the method of choice when covalent anchoring of POMs to CPs is desired. A series of POM/CP composites have recently been reported by Liu et al. The group developed organoimido-derivatized Lindqvist anions, [Mo6O18NR]2− (R = organic linker to the polymer backbone) which were covalently attached to a phenylene-ethynylene polymer.43 The POM/CP materials obtained showed solvent-dependent behaviour: in nonpolar solvents they assembled into reverse vesicles where the polymer backbone is exposed to the solvent and the POM-functionalized side-chains form the solvophobic interior. In contrast, in polar solvents, the composite behaves like a typical anionic polyelectrolyte and assembles into micro-tubular structures. Thereby facile access to structurally switchable, electrically conductive nanostructures is possible.
In an expansion of their studies, the same authors found that modification of the organic linker between POM and CP backbone has significant effects on the photochemical properties of the material: when conjugated linkers were used, high fluorescence quenching was observed, whereas flexible, non-conductive alkyl linkages resulted in significantly lower quenching rates, suggesting that photoinduced intra-chain electron-transfer might be the dominating quenching mechanism.44
2.2.2. Backbone incorporation.
One rare example of POM incorporation in the polymer backbone employs difunctionalized Lindqvist-type molybdates50 [Mo6O17(NR)2]2− (R = iodo- or ethynyl-functionalized linkages) as monomeric building units for incorporation into the backbone of poly-(phenylene acetylene). By introduction of iodo- or ethynyl-functionalized side groups on the POM, Pd-catalyzed coupling reactions could be used for controlled polymerization, giving access to a POM/CP composite. The material was used for charge-separation in a photovoltaic cell and significantly higher conversion rates were observed compared with non-POM-functionalized CP systems, demonstrating that the POMs form stable building units suitable for composite synthesis, processing and application.51
3. Current fields of application
In the following chapter we discuss current applications of POM/CP composites which highlight the synergetic effects of combining both compound classes in one material.
3.1. Electrochemical energy storage
Electrochemical capacitors fill an important performance gap between conventional capacitors and batteries by supplying high-power electric pulses over short time scales (up to tens of seconds).39 In so-called supercapacitors, high capacitance is achieved by combining electrochemical double-layer capacitance with pseudocapacitance arising from reversible faradaic redox reactions.6c Therefore, redox active metal oxides (e.g. RuO2)52 are promising materials for supercapacitors. However, often, their low stability, low electrical conductivity and high cost limit their technological use on a large scale.53 By employing molecular metal oxides embedded in CPs enhanced stability, electrical conductivity and facile fabrication can be combined in one material with the additional benefit of the facile tunability of the chemical properties of the POMs.54
In particular electrodes based on protonated polyoxometalates (so-called heteropolyacids, HPAs) combine acidic stability, high proton mobility and high redox chemistry, making them ideal components for supercapacitors.
Yamada55 reported the assembly of an inexpensive electrochemical capacitor system where HPAs ([H3PMo12O40]) were immobilized in a Nafion proton-exchange membrane and assembled into a capacitor cell. High reversible specific capacitance (112 F g−1) and energy density (36 J g−1) with an operating voltage of 0.8 V were observed. It should be noted that the Nafion does not act as an electrically conducting polymer but instead is used as a proton-conductive membrane.
Asymmetric supercapacitors based on two POM/CP composites were recently fabricated by Freund et al.:39 to this end, PPy/PMo12 was used as the positive electrode and PEDOT/PW12 was employed as the negative electrode. Using sulphuric acid (0.5 M) as electrolyte, an operating voltage of ∼1 V, a power density of 103 W kg−1, energy density of 14 J g−1 and a capacitance of 31 F g−1 were achieved. Long-term degradation of the PPy/PMo12 electrode due to over-oxidation of the polypyrrole was observed, resulting in a severe loss of capacitance after 200 cycles. In contrast, the PEDOT/PW12 electrode remained intact.
3.2. Photoelectrochemical devices
POMs have recently attracted wide attention as promising materials for charge separation and charge transport in photovoltaic and photoelectrochemical systems. In a proof of concept, Wang et al. used electropolymerization to deposit a composite of the lacunary Keggin anion [SiW11O39]8− and PEDOT on an indium tin oxide (ITO) electrode. The electrode was used as counter-electrode in a classical dye-sensitized solar cell (DSSC). Performance characteristics comparable with the standard Platinum electrodes typically employed in DSSCs were observed together with high electrochemical stability.56
A different approach was recently reported by Proust, Tortech et al. where a layered organic solar cell configuration was assembled using the Dawson anion [P2W18O62]6− as an electroactive interface between the indium tin oxide electrode and a conductive polymer bulk heterojunction. The bulk heterojunction was based on poly(3-hexylthiophene) (= P3HT) and [6,6]-phenyl-C61-butyric acid methyl ester (= PCBM).57 This cell configuration showed better conversion efficiency than a POM-free reference system, and in addition, high current density and optimized open-circuit voltage were observed. The authors assign this improved performance to the presence of discrete in-gap energy states in the POM layer, which may facilitate charge transport and make the POM-layer conductive, see Fig. 6.57
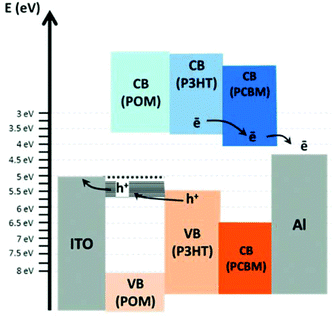 |
| Fig. 6 Energy scheme of the organic solar cell based on a layered assembly of POM/P3HT/PCBM sandwiched between a transparent ITO electrode and an Al counterelectrode. Reproduced from ref. 57 with permission of the Royal Society of Chemistry. | |
In an extension of this work, Palilis, Vasilopoulou et al. used a similar device setup featuring the Keggin anion PW12 as an electron extraction layer. The study showed that a higher efficiency was observed together with improved device stability. The authors ascribe the enhanced performance to the improved electron extraction at the cathode interface due to an energy level matching between the POM layer and the aluminium cathode.58 The authors also showed that the same concept can be employed to improve the performance of hybrid light emitting diodes (HyLEDs).59
In a related study, Xu, Du et al. recently demonstrated that POM anions can also be employed as electron trapping sites in photovoltaic cells.60 The authors assembled a composite based on cobalt phthalocyanine as photosensitizer, Dawson anions [P2W18O62]6− as electron acceptors/transfer sites embedded in a poly(styrene sulfonate) (PSS)/poly(allylamine hydrochloride) (PAH) conductive copolymer. An increased photovoltaic effect compared with the non-POM containing reference was observed. Photophysical studies suggested that upon irradiation, an electron transfer from the cobalt phthalocyanine to the POM is observed which resulted in an improved electron–hole separation. The initial studies illustrate that by matching the electronic properties of photosensitizer, electron traps and CPs, the overall performance of photovoltaic devices can be optimized on the molecular level.
3.3. Substrate sensing (biomolecules, pollutants, toxins)
POM/CPs are ideal composites for substrate sensing as the molecularly dispersed POMs act as redox-active substrate binding sites to signal the presence of a substrate while the CP converts the signal into an electrical information for further (quantitative) detection.20 McCormac et al. recently reported a composite film of Dawson anions immobilized in electropolymerized PPy.49 The materials were used as amperometric sensors for hydrogen peroxide (detection limit: 0.3 mM). Redox processes associated with all components were accessible for voltammetric studies and stable redox responses in the pH range of 2–7 were reported. Notably, H2O2 detection was only achieved when transition-metal functionalized Dawson anions ([P2W17O61M]n−) (M = Fe3+, Cu2+, Co2+) were used. In contrast, the non-functionalized parent anion ([P2W18O62]6−) did not result in H2O2 detection, illustrating the need for chemically tuneable substrate binding and sensing sites.
Further, in comparison with related H2O2 sensors based on identical Dawson anions in solution61 or assembled on electrodes by layer-by-layer (LbL) techniques,62 the POM/CP composites revealed improved H2O2 detection characteristics and high long-term redox-stability, highlighting the technological viability of the systems.
The use of POM/CP composites for gas sensing was reported by Ammam et al. who showed that nanostructured POM/PPy composites can be used for NOx detection. To this end, pyrrole was polymerized in situ using the Dawson anion P2Mo18 as oxidant. The resulting semiconducting composite showed high selectivity for detection of gaseous NOx and wide NOx-concentration dependent linear response was observed.20
3.4. Nanoparticle wiring in conductive matrices
The combination of metallic nanoparticles (NPs) as electrocatalytically active sites together with polyoxometalates as electron relays or structural/chemical stabilizers in a CP matrix holds great promise for the design of highly active materials for sensing and catalysis. The concept has been illustrated by Kulesza et al. who have recently grown multilayer networks of POM (here: PMo12)-stabilized platinum nanoparticles (size: 5–10 nm) embedded in ultrathin PANI films.63 The resulting composites showed activity as oxygen reduction catalysts and might be useful in future fuel cell systems, see Fig. 7. In addition, these systems can be considered molecular model systems to investigate and understand the activity-enhancing effects of metal oxide (nano-) particles on noble metal NP catalysts.64
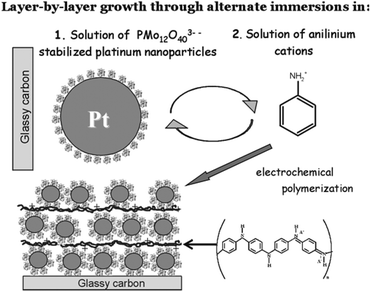 |
| Fig. 7 Illustration of the assembly process of POM-stabilized Pt nanoparticles sandwiched between ultrathin layers of PANI for electrochemical sensors. Reproduced from ref. 63 with permission of the American Chemical Society. | |
An intriguing route to POM/NP/CP composites was recently reported by Viswanathan et al. who used redox-active Keggin anions [PMo12O40]3− to initiate the oxidative polymerization of aniline to PANI. The reduced Keggin anion [PMoVMoVI11O404−] subsequently acts as reductant for the formation of metal NPs. Thus, the authors gained access to POM-containing Ag and Au NP-functionalized PANI composites (Fig. 8).65
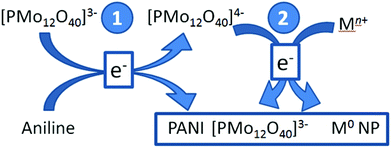 |
| Fig. 8 Illustration of the two-step formation of metal nanoparticle/POM/PANI composites. Step 1: reduction of POM and oxidative polymerization of aniline. Step 2: the reduced POM reduces metal salts, giving metal nanoparticles (here: Au, Ag). All three components are simultaneously assembled into a nanocomposite. Adapted from ref. 65 with permission of Springer Publishers. | |
3.5. Electrochemical catalysts
Linkage of redox-active POMs to electrode surfaces is an elegant route to highly reactive electrocatalysts20 and has been exploited to improve their electrochemical stability and catalytic performance.66 The approach is attractive as it gives access to heterogeneous catalysts with activities approaching those of homogeneous systems. A recent landmark paper by McCormac et al. highlights the potential of POM/CP composites in electrocatalysis. Using layer-by-layer (LbL) assembly, the prototype water oxidation catalyst (WOC) Na10[{Ru4O4(OH)2(H2O)4}(γ-SiW10O36)2] (= Ru4POM)67 was embedded in a PPy matrix assembled onto glassy carbon electrodes or indium tin oxide (ITO). In the composite, the ruthenium-based redox-waves were still observed while the tungsten-based redox-waves were not detected. The composite films show pH dependent redox activity (pH-range 2–5) and feature excellent redox cycling stability. Electron microscopy showed that the films are porous, giving a large substrate-accessible surface area. Initial tests showed that the Ru4POM-based LbL assemblies exhibit promising electrocatalytic water oxidation activity at neutral pH where the onset of water oxidation was observed at ca. 1.1 V (vs. Ag/AgCl). Compared with a Ru4POM paste electrode, the multilayer assembly showed higher oxidation currents, and therefore better performance for the electrooxidation of water.68
4. Future perspectives for applications
4.1. Lightweight, high-capacity battery materials
All composites discussed earlier contained W- or Mo-based POMs as inorganic redox-active components. As the clusters feature rather high molecular weights, important technological factors such as charge density and specific capacitance are limited. One future goal therefore is the development of 3d-metal-based POM/CP composites for high-performance, low-weight capacitors and batteries. Thus, vanadium-based POMs are ideal candidates. The analogous solid-state metal oxide, vanadium pentoxide (V2O5) has already been explored in this context and lamellar mesostructured nanocomposites of V2O5 intercalated in polyaniline (PANI) were fabricated.69 Oxidative polymerization of aniline is observed when V2O5 is brought in contact with it and strong hydrogen bonding between the two components was observed spectroscopically. As such, this initial study illustrates that vanadium-based materials, and in particular metal-functionalized vanadate clusters might be ideal candidates to develop electrochemically tuneable composites for energy storage.70
While the bulk electronic conductivity of POMs is typically negligible, the preparation of POM containing conducting materials9 using methods discussed previously highlights the scope for the design of novel composites and development of this research area. An area of POM chemistry with great potential in this respect is that of the organo-imido functionalised POMs.71 As eluded to previously, polyanions of this type can be relatively easily synthesised and processed while their electronic properties can also be finely tuned due to conjugation between the polyanion and the organic component through d–π electronic transitions.72 A recent report illustrates this with the 2,6-dimethyl-4-thiocyano aniline functionalised Lindqvist polyanion (Mo6-SCN). The anion shows excellent properties as the anode in a lithium ion battery.73 The presence of the electron withdrawing thiocyano group attached to the polyanion results in a dramatic lowering of the LUMO+n (n = 1–3) frontier orbital energies compared to derivatives such as 2,6 dimethylaniline and 2,4,5-trimethylaniline. This reduction of the HOMO–LUMO band gap consequently results in more facile redox cycling, with the relative lowering of the LUMO+2 and +3 in particular resulting in increased capacity. Pristine Mo6-SCN boasts an 85% capacitance retention after 100 cycles of 876 mA h g−1 with approximately 100% coulombic efficiency at a testing current density of 50 mA g−1.
As described above (section 1.2), POM/nanocarbon composites are currently investigated as cathode materials, with the poor intrinsic electrical conductivity of the POMs being overcome by their adsorption onto well-established conducting substrates. Recently the use of carbon black74 and single walled carbon nanotubes (SWNTs)75 to generate conducting POM composites has proven a useful strategy; however, the development of a POM/graphene (reduced graphene oxide, RGO) hybrid yields the highest capacity.76 Similar performance characteristics might be accessible with POM/CP composites, which benefit from potentially more synthetic control regarding the degree of interaction between the POM cluster as a charge storage site and the CP wiring.
Taking into consideration these two different approaches, it is apparent that close collaborative efforts between chemists and electrical engineers is required to continue the growth of this research area. Chemically, much scope exists for the design and synthesis of molecular clusters containing mixed metal compositions that will facilitate both considerable redox cycling and attachment of relevant organic components. The development of these complementary materials will assist to maximise interactions with conducting polymers and also the molecules interface with capacitive materials such as SWNTs and RGO. Recent work by the groups of Wang and Song provide a solid foundation upon which to be built in this research direction.77 Furthermore the development of intrinsically conducting POM based polymers/architectures is an ambitious yet plausible set of synthetic targets that should be revisited.24b
4.2. Fuel-cell proton-conducting membranes
The development of a proton exchange membrane (PEM), with excellent proton conductivity at high temperature and low humidity is of great importance for improved proton-exchange membrane fuel cells (PEMFCs). Operating fuel cells at increased temperature results in minimising anode catalyst poisoning by CO, avoids fuel cell flooding and gives increased efficiency and faster kinetics.78 Despite much progress over the last decade, it should however be noted that the exact membrane function is still not fully understood79 and further work is urgently required to identify the key mechanisms which determine membrane performance under operating conditions.
One typical synthetic route uses the basic polymer polybenzimidazole (PBI) which can be complexed by strong acids like phosphoric acid, making it a viable proton conducting material. The polymer composite features promising properties for the use as a polymer electrolyte in high temperature (ca. 150 to 200 °C) PEM fuel cells.80 Phosphomolybdic acid (PMo12) is an excellent proton conductor and has been used to replace phosphoric acid in several PEM fuel cells.81 Li et al. synthesized PMo12/PBI composites and demonstrated the promising properties of the water insoluble proton exchange membrane and significantly higher proton conductivity (>0.15 S cm−1) was observed compared with a phosphoric acid-only doped PBI membrane. High long-term stability in 200 h runs at 150 °C was also observed. The authors suggest the possibility of a chemical bond between the POM and PBI in the composite membrane based on 31P-NMR data.
4.3. Redox-switchable nanostructures towards “artificial muscles”
The development of “artificial muscles”82 based on actuators, i.e. materials which convert energy (e.g. electricity) into motion holds great promise for robotics, microfluidics and micromechanics as well as other fields of technology.83 One strategy to induce physical motion in polymeric materials is to use conducting polymers where changes in volume are observed upon applying an electric potential. This concept has been used to fabricate PPy-based actuators where length changes of up to 12% were observed at potentials <1 V.84 This concept can in principle be expanded to POM-modified CPs where the POM acts as an electron-storage site to accumulate negative charge and thus increase inter-cluster electrostatic repulsion upon reduction; it can be envisaged that this leads to a significant volume increase. The concept is particularly appealing when considering that CP-based actuators can displace significant loading weights (up to 100× the typical loads observed for mammalian muscles) and also feature a so-called catch-state where a given displacement can be maintained without further energy input, making them interesting for low-energy-consumption electromechanical devices.85
Furthermore, the development of POM/CPs based on p-type organic semiconductors will require an increase in charge-balancing anions on “hole” accumulation within the polymer. The use of large POMs bearing low anionic charge may be a strategy to induce actuation as a result of the polymers volume expansion on incorporation of the POM. Once again, hybrid inorganic–organic POM hybrids may be particularly useful in this role due to our ability to control the nature of their functionalization as well as their effective charge distribution.86 Important interactions to consider with such an approach include the involvement of the redox activity of the selected POM as well as factors that may influence their mobility into and out of a given polymer matrix. Another strategy could be the development of an electrolyte storage type actuator, where two different POM/CP electrodes are prepared, one of which contains an immobilised polyoxoanion and the other an immobilised polyoxocation such as [E-PMo12O36(OH)4[La(H2O)4]]5+.87 The electrolyte layer sandwiched between the electrodes then acts as a reservoir of mobile ions that can be reversibly doped into and removed from the electrodes on redox cycling. Ultimately this process will result in actuation.
4.4. High-sensitivity sensors for aqueous toxins
As discussed earlier, numerous methods exist for the development of excellent POM based sensors. However, in order to increase sensitivity and robustness, the POM anions need to be embedded and electrically wired to a conductive, high surface-area substrate to enable efficient read-out of the detection event. A notable report by Kurth et al. discusses the design and function of a smart POM-based nitrogen monoxide (NO) sensor with ppb sensitivity and tuneable sensitivity through control of the device assembly procedure.88 Using the cobalt containing Dawson based POM [CoII4(H2O)2(P2W15O56)2]16− the authors took advantage of the two well-placed reversible two electron reduction processes of the POM for the selective electrocatalytic reduction of NO. The electrode was constructed using layer-by-layer deposition where alternating layers of the polyelectrolytes poly(styrenesulfonate) (PSS) and poly(allylamine hydrochloride) (PAH) were adsorbed onto the (3-aminopropyl)triethoxysilane functionalised ITO. Subsequently, multiple layers of the POM and PAH were grown on the electrode. The systems showed selective reduction of NO over nitrite and nitrate, despite their similar reduction potentials. Reasons for this include electrostatic repulsion between the polyanions and the negatively charged nitrite and nitrate, as well as the clever completion of the electrode with a negatively charged PSS/PAH/PSS multilayer which prevents diffusion of the anions through the electrode to the incorporated POMs. Clearly the use of polyelectrolytes (PE) is essential to control the permeability of the analyte to the redox active POMs incorporated in the electrode, however a multi-layered design is required due the lower electrochemical response of the POM when fewer layers are used.
To maximise the POM-based electrochemical response to analytes and to improve selectivity by controlling permeability of the electrode, POM-polyelectrolyte (POM-PE) electrodes linked to CPs should be targeted. One strategy to achieving this may involve the design and synthesis of organically derivatised POMs capable of acting as POM-PE monomers. Use of traditional electropolymerisation techniques to grow conducting polymers in the presence of these monomers may then yield both highly sensitive and selective composites POM-PEs@CPs. Also the use of water soluble conducting polyelectrolytes such as sulfonated polyanilines may hold promise as matrixes capable of improved performance, particularly for aqueous toxin detection and possibly also simultaneous remediation.89
In order to utilize POMs in the development of bio-recognition-sensors, the interactions between POMs and the biological molecules of interest must first be assessed. It is well-known that POMs have notable interactions with proteins; however the quantification of these interactions in terms of sensing applications is in its infancy. Understandably this is largely due to the lack of sufficient knowledge regarding the specific interactions of POMs with proteins compared to the relative ease of identifying suitable redox couples for POM based electrochemical sensors. The following literature reports summarise three different approaches to the development of POM based bio-sensors, followed by our perspective on a future strategy.
Recently, the interactions between various polyoxotungstates including plenary, lacunary, lanthanoid, and transition metal containing analogues with human and bovine serum albumin (HSA and BSA) were investigated.90 This work showed that the POM–protein interactions are highly dependent on size, shape, charge and any embedded metal ions incorporated within the POM.
A recent report illustrated how organo-functionalized derivatives of [PW11O39]7− can be used as scaffolds for constructing bio-sensors which combine the advantages of the POM and the organic component. Two prototypes are [PW11O39{(SiC6H4NH2)2O}]3− (Amine-PW11) and [PW11O39{(SiC21H16NO2)2O}]4− (Py-PW11) bearing pendant amine and pyrene residues respectively. Amine-PW11 was elegantly grafted to a gold surface through only one of the pendant amines, leaving the remaining amine free for further linkage to a secondary antirabbit immunoglobin (anti-rIgG). The composite biosensor showed specific recognition of rabbit immunoglobin and non-specific binding of BSA was also observed.91
The ability of the pyrene bearing Py-PW11 to form strong π–π interactions was exemplified by binding the cluster to the surface of single-walled carbon nanotubes (SWNTs) and energy transfer from the pyrene to the SWNTs was observed by quenching of the typical pyrene centred emission. The (Py-PW11)@SWNT composites were found to interact strongly with HSA and the properties of this and related materials are under investigation regarding their potential biosensor applications.92
These studies show that POM based biosensors are achievable and well-designed POM/CP composites can make a valuable contribution to the current material design efforts. One non-POM based strategy that we can learn from has been developed for the sensing of aqueous pollutants such as ricin and E. Coli. This approach utilises the less toxic model protein Concanavalin A to probe sugar–protein interactions where a conjugated polymer is decorated with pendant sugars.93 On binding of the protein to the sugars, the change in the polymer structure is reflected by modulation of its fluorescence properties. This occurs due to the presence of fluorene donor and benzothiadiazole acceptor units within the backbone, resulting in Förster resonance energy transfer (FRET) with sensitivity in the ppb range. Considering the intrinsic properties of POMs, the development of water soluble conjugated polymers containing POM pendant groups may be an interesting prospect for the sensing of water soluble toxins with well-defined positive surface charge density. One important consideration is the desired output of the sensor, as the use of conjugated linkers between POMs and CPs have shown significant quenching of fluorescence properties due to intra-chain electron-transfer. Consequently for a sensor using fluorescence as the signal, the use of appropriate linkers between the POM and CP will be crucial to the materials viability.
In summary, the development of POM/CP bio-sensors that show selectivity and sensitivity towards a given toxin will be driven by the POM charge, size and shape and redox potential as well as the covalent attachment method (stability), linker (length and hydrophobicity), and polymer backbone structure (signal output).
4.5. Supramolecular POM/CP composite assembly
The regioselective deposition of POM anions at specific sites of conductive polymers would allow the tuning of materials properties such as electron transfer, cooperative cluster interactions and synergistic redox-switching. As discussed earlier, this can in principle be achieved by covalent functionalization routes, however, often, these are limited by complex syntheses. Switching to supramolecular assembly routes, it can be envisaged that POMs attach to specific, pre-designed binding sites within the CP through intermolecular interactions such as electrostatics, hydrogen-bonding, or coordinative interactions (or a combination thereof). As a consequence, using well-known supramolecular assembly principles, functional materials with controllable properties could become accessible.26a These concepts have already been realized for composites assembled from POMs and non-conductive polymers. In one example, POM-PS nanoparticles were formed by first assembling supramolecular POM-surfactant aggregates in the presence of styrene, resulting in the formation of a microemulsion. Polymerization of the microemulsion droplets gave access to POM/PS nanoparticles in the 50 nm size range.94 Future work is therefore required to transfer these results into the POM/CP domain.
5. Conclusions
In conclusion, we have presented the design principles which are currently employed to access polyoxometalate/conductive polymer composites; their advantages and associated challenges were discussed and potential future developments in the field were described. The applications of POM/CP composites in technologically relevant areas such as electrochemical and photoelectrochemical energy storage, sensors with ppb-sensitivity and electrochemical catalysis have been described and the current state of the art together with future target applications were discussed.
In the outlook section, a number of potential future applications were critically discussed based on developments which in the authors’ mind have significant potential or which have recently been addressed in pioneering studies.
In summary, the field of POM/CP composites is one of the fastest-moving fields that targets the incorporation of electroactive POMs in conductive matrices and offers facile materials design by control of the properties of both the inorganic POMs and the organic CPs.
Acknowledgements
S.H. acknowledges funding by the Fonds der Chemischen Industrie; C.S. gratefully acknowledges Ulm University and the Deutsche Forschungsgemeinschaft DFG (STR1164/4-1) for financial support. C.R. acknowledges the Australian Research Council ARC (DE130100615) for financial support. C.R. gratefully acknowledges financial support by the University of Melbourne.
Notes and references
-
H. F. Mark, Encyclopedia of Polymer Science and Technology, Wiley, New York, 4 edn, 2014 Search PubMed.
-
(a) A. J. Heeger, Angew. Chem., Int. Ed., 2001, 40, 2591–2611 CrossRef CAS;
(b) A. G. MacDiarmid, Angew. Chem., Int. Ed., 2001, 40, 2581–2590 CrossRef CAS;
(c) H. Shirakawa, Angew. Chem., Int. Ed., 2001, 40, 2574–2580 CrossRef.
- P. L. Burn, A. W. Grice, A. Tajbakhsh, D. D. C. Bradley and A. C. Thomas, Adv. Mater., 1997, 9, 1171–1174 CrossRef CAS.
- U. Lange, N. V. Roznyatovskaya and V. M. Mirsky, Anal. Chim. Acta, 2008, 614, 1–26 CrossRef CAS PubMed.
- F. Jonas, W. Krafft and B. Muys, Macromol. Symp., 1995, 100, 169–173 CrossRef.
-
(a) K. Gurunathan, A. V. Murugan, R. Marimuthu, U. P. Mulik and D. P. Amalnerkar, Mater. Chem. Phys., 1999, 61, 173–191 CrossRef CAS;
(b) A. Rudge, J. Davey, I. Raistrick, S. Gottesfeld and J. P. Ferraris, J. Power Sources, 1994, 47, 89–107 CrossRef CAS;
(c) G. A. Snook, P. Kao and A. S. Best, J. Power Sources, 2011, 196, 1–12 CrossRef CAS PubMed.
- S. Günes, H. Neugebauer and N. S. Sariciftci, Chem. Rev., 2007, 107, 1324–1338 CrossRef PubMed.
- M. J. L. a. S. J. Dong, Electrochim. Acta, 1995, 40, 197–200 CrossRef.
- H. Yang, T. Song, L. Liu, A. Devadoss, F. Xia, H. Han, H. Park, W. Sigmund, K. Kwon and U. Paik, J. Phys. Chem. C, 2013, 117, 17376–17381 CAS.
- E. M. G. G. Bidan and M. Lapkowski, Synth. Met., 1989, 31, 327–334 CrossRef.
- M. Baibarac, I. Baltog, I. Smaranda and A. Magrez, Carbon, 2015, 81, 426–438 CrossRef CAS PubMed.
- S. Liu and Z. Tang, Nano Today, 2010, 5, 267–281 CrossRef CAS PubMed.
- G. M. Suppes, C. G. Cameron and M. S. Freund, J. Electrochem. Soc., 2010, 157, A1030–A1034 CrossRef CAS PubMed.
- H. Nishide and K. Oyaizu, Science, 2008, 319, 737 CrossRef CAS PubMed.
- G. Kickelbick, Prog. Polym. Chem., 2003, 28, 83–114 CrossRef CAS.
- D. E. Katsoulis, Chem. Rev., 1998, 98, 359–388 CrossRef CAS PubMed.
-
(a) POM-themed issue: L. Cronin and A. Müller (guest eds.), Chem. Soc. Rev., 2012, 41, 7325–7648;
(b) POM-themed issue: C. L. Hill (guest ed.), Chem. Rev., 1998, 98, 1–387;
(c)
M. T. Pope, Heteropoly and isopoly oxometalates, Springer-Verlag, Heidelberg, 1983 Search PubMed.
- A. Müller, V. P. Fedin, C. Kuhlmann, H. Bogge and M. Schmidtmann, Chem. Commun., 1999, 927–929 RSC.
- A. Müller, S. K. Das, H. Bögge, C. Beugholt and M. Schmidtmann, Chem. Commun., 1999, 1035–1036 RSC.
- M. Ammam, J. Mater. Chem. A, 2013, 1, 6291–6312 CAS.
- A. Giusti, G. Charron, S. Mazerat, J.-D. Compain, P. Mialane, A. Dolbecq, E. Rivière, W. Wernsdorfer, R. Ngo Biboum, B. Keita, L. Nadjo, A. Filoramo, J.-P. Bourgoin and T. Mallah, Angew. Chem., Int. Ed., 2009, 48, 4949–4952 CrossRef CAS PubMed.
-
(a) H. Wang, S. Hamanaka, Y. Nishimoto, S. Irle, T. Yokoyama, H. Yoshikawa and K. Awaga, J. Am. Chem. Soc., 2012, 134, 4918–4924 CrossRef CAS PubMed;
(b) N. Kawasaki, H. Wang, R. Nakanishi, S. Hamanaka, R. Kitaura, H. Shinohara, T. Yokoyama, H. Yoshikawa and K. Awaga, Angew. Chem., Int. Ed., 2011, 123, 3533–3536 CrossRef;
(c) X. Lopez, J. A. Fernandez and J. M. Poblet, Dalton Trans., 2006, 1162–1167 RSC;
(d) H. Wang, N. Kawasaki, T. Yokoyama, H. Yoshikawa and K. Awaga, Dalton Trans., 2012, 41, 9863–9866 RSC;
(e) X. López, C. Bo and J. M. Poblet, J. Am. Chem. Soc., 2002, 124, 12574–12582 CrossRef PubMed.
- Y. F. Song and R. Tsunashima, Chem. Soc. Rev., 2012, 41, 7384–7402 RSC.
-
(a) E. Coronado and C. J. Gómez-García, Chem. Rev., 1998, 98, 273–296 CrossRef CAS PubMed;
(b) R. Tsunashima, Y. Iwamoto, Y. Baba, C. Kato, K. Ichihashi, S. Nishihara, K. Inoue, K. Ishiguro, Y.-F. Song and T. Akutagawa, Angew. Chem., Int. Ed., 2014, 53, 11228–11231 CrossRef CAS PubMed.
- P. J. Kulesza and L. R. Faulkner, J. Am. Chem. Soc., 1993, 115, 11878–11884 CrossRef CAS.
-
(a) Y.-F. Song and R. Tsunashima, Chem. Soc. Rev., 2012, 41, 7384–7402 RSC;
(b) C. Bosch-Navarro, B. Matt, G. Izzet, C. Romero-Nieto, K. Dirian, A. Raya, S. I. Molina, A. Proust, D. M. Guldi, C. Marti-Gastaldo and E. Coronado, Chem. Sci., 2014, 5, 4346–4354 RSC;
(c) D. Ma, L. Liang, W. Chen, H. Liu and Y.-F. Song, Adv. Funct. Mater., 2013, 23, 6100–6105 CrossRef CAS;
(d) Y. Ji, L. Huang, J. Hu, C. Streb and Y.-F. Song, Energy Environ. Sci., 2015, 8, 776–789 RSC.
- S. Fan, F. Xiao, L. Liu, F. Zhao and B. Zeng, Sens. Actuators, B, 2008, 132, 34–39 CrossRef CAS PubMed.
- F. M. Toma, A. Sartorel, M. Iurlo, M. Carraro, P. Parisse, C. Maccato, S. Rapino, B. R. Gonzalez, H. Amenitsch, T. Da Ros, L. Casalis, A. Goldoni, M. Marcaccio, G. Scorrano, G. Scoles, F. Paolucci, M. Prato and M. Bonchio, Nat. Chem., 2010, 2, 826–831 CrossRef CAS PubMed.
- Z. Sun, L. Xu, W. Guo, B. Xu, S. Liu and F. Li, J. Phys. Chem. C, 2010, 114, 5211–5216 CAS.
- R. R. Ozer and J. L. Ferry, Environ. Sci. Technol., 2001, 35, 3242–3246 CrossRef CAS.
-
(a) M. Carraro and S. Gross, Materials, 2014, 7, 3956–3989 CrossRef CAS PubMed;
(b) W. Qi and L. Wu, Polym. Int., 2009, 58, 1217–1225 CrossRef CAS.
-
(a) Y. Han, Y. Xiao, Z. Zhang, B. Liu, P. Zheng, S. He and W. Wang, Macromolecules, 2009, 42, 6543–6548 CrossRef CAS;
(b) Y.-K. Han, Z.-J. Zhang, Y.-L. Wang, N. Xia, B. Liu, Y. Xiao, L.-X. Jin, P. Zheng and W. Wang, Macromol. Chem. Phys., 2011, 212, 81–87 CrossRef CAS.
- J. Rieger, T. Antoun, S.-H. Lee, M. Chenal, G. Pembouong, J. Lesage de la Haye, I. Azcarate, B. Hasenknopf and E. Lacôte, Chem. – Eur. J., 2012, 18, 3355–3361 CrossRef CAS PubMed.
-
(a) D. Schaming, C. Allain, R. Farha, M. Goldmann, S. Lobstein, A. Giraudeau, B. Hasenknopf and L. Ruhlmann, Langmuir, 2009, 26, 5101–5109 CrossRef PubMed;
(b) C. Costa-Coquelard, S. Sorgues and L. Ruhlmann, J. Phys. Chem. A, 2010, 114, 6394–6400 CrossRef CAS PubMed;
(c) J. L. de la Haye, P. Beaunier, L. Ruhlmann, B. Hasenknopf, E. Lacote and J. Rieger, ChemPlusChem, 2014, 79, 250–256 CrossRef.
-
(a) H. Chen, L. Xie, H. Lu and Y. Yang, J. Mater. Chem., 2007, 17, 1258–1261 RSC;
(b) P. Xiao, C. Simonnet-Jegat, F. Dumur, G. Schrodj, M.-A. Tehfe, J. P. Fouassier, D. Gigmes and J. Lalevee, Polym. Chem., 2013, 4, 4526–4530 RSC.
- T. F. Otero, S. A. Cheng, E. Coronado, E. M. Ferrero and C. J. Gómez-García, ChemPhysChem, 2002, 3, 808–811 CrossRef CAS.
- P. Gómez-Romero, K. Cuentas-Gallegos, M. Lira-Cantú and N. Casañ-Pastor, J. Mater. Sci., 2005, 40, 1423–1428 CrossRef.
- H. S. S. Sung and W. K. Paik, Electrochim. Acta, 1994, 39, 645–650 CrossRef CAS.
- G. M. Suppes, C. G. Cameron and M. S. Freund, J. Electrochem. Soc., 2010, 157, A1030–A1034 CrossRef CAS PubMed.
-
(a) M. S. Freund and N. S. Lewis, Proc. Natl. Acad. Sci. U.S.A., 1995, 92, 2652–2656 CrossRef CAS;
(b) M. S. Freund, C. Karp and N. S. Lewis, Inorg. Chim. Acta, 1995, 240, 447–451 CrossRef CAS.
- T. McCormac, D. Farrell, D. Drennan and G. Bidan, Electroanalysis, 2001, 13, 836–842 CrossRef CAS.
-
(a) A. Proust, B. Matt, R. Villanneau, G. Guillemot, P. Gouzerh and G. Izzet, Chem. Soc. Rev., 2012, 41, 7605–7622 RSC;
(b) A. Proust, R. Thouvenot and P. Gouzerh, Chem. Commun., 2008, 1837–1852 RSC.
- P. Yin, L. Jin, D. Li, P. Cheng, D. V. Vezenov, E. Bitterlich, X. Wu, Z. Peng and T. Liu, Chem. – Eur. J., 2012, 18, 6754–6758 CrossRef CAS PubMed.
- B. Xu, M. Lu, J. Kang, D. Wang, J. Brown and Z. Peng, Chem. Mater., 2005, 17, 2841–2851 CrossRef CAS.
- S. Cheng, T. Fernández-Otero, E. Coronado, C. J. Gómez-García, E. Martínez-Ferrero and C. Giménez-Saiz, J. Phys. Chem. B, 2002, 106, 7585–7591 CrossRef CAS.
- T. F. Otero, S. A. Cheng, D. Alonso and F. Huerta, J. Phys. Chem. B, 2000, 104, 10528–10533 CrossRef CAS.
- H. Sung, H. So and W.-k. Paik, Electrochim. Acta, 1994, 39, 645–650 CrossRef CAS.
- Y. Z. L. Li, J. Energy Chem., 2005, 14, 35–39 Search PubMed.
- N. Anwar, M. Vagin, F. Laffir, G. Armstrong, C. Dickinson and T. McCormac, Analyst, 2012, 137, 624–630 RSC.
- Z. Peng, Angew. Chem., Int. Ed., 2004, 43, 930–935 CrossRef CAS PubMed.
- M. Lu, B. Xie, J. Kang, F.-C. Chen, Yang and Z. Peng, Chem. Mater., 2004, 17, 402–408 CrossRef.
- B. E. Conway, J. Electrochem. Soc., 1991, 138, 1539–1548 CrossRef CAS PubMed.
-
(a) R. N. Reddy and R. G. Reddy, J. Power Sources, 2003, 124, 330–337 CrossRef CAS;
(b) T. Brousse and D. Belanger, Electrochem. Solid-State Lett., 2003, 6, A244–A248 CrossRef CAS PubMed.
- P. Gómez-Romero, M. Chojak, K. Cuentas-Gallegos, J. A. Asensio, P. J. Kulesza, N. Casañ-Pastor and M. Lira-Cantú, Electrochem. Commun., 2003, 5, 149–153 CrossRef.
- J. B. Atsuo Yamada, J. Electrochem. Soc., 1998, 145, 737–743 CrossRef PubMed.
- C. Yuan, S. Guo, S. Wang, L. Liu, W. Chen and E. Wang, Ind. Eng. Chem. Res., 2013, 52, 6694–6703 CrossRef CAS.
- M. Alaaeddine, Q. Zhu, D. Fichou, G. Izzet, J. E. Rault, N. Barrett, A. Proust and L. Tortech, Inorg. Chem. Front., 2014, 1, 682–688 RSC.
- L. C. Palilis, M. Vasilopoulou, A. M. Douvas, D. G. Georgiadou, S. Kennou, N. A. Stathopoulos, V. Constantoudis and P. Argitis, Sol. Energy Mater. Sol. Cells, 2013, 114, 205–213 CrossRef CAS PubMed.
- L. C. Palilis, M. Vasilopoulou, D. G. Georgiadou and P. Argitis, Org. Electron., 2010, 11, 887–894 CrossRef CAS PubMed.
- Y. Yang, L. Xu, F. Li, X. Du and Z. Sun, J. Mater. Chem., 2010, 20, 10835–10840 RSC.
- R. C. I. M. Mbomekalle, K. I. Hardcastle, C. L. Hill, M. Ammam, B. Keita, L. Nadjo and T. M. Anderson, C. R. Chim., 2005, 8, 1077–1086 CrossRef PubMed.
- S. Y. G. S. Y. Zhai, J. U. Jiang, S. J. Dong and J. H. Li, Anal. Chim. Acta, 2003, 486, 85–92 CrossRef CAS.
- P. J. Kulesza, M. Chojak, K. Karnicka, K. Miecznikowski, B. Palys, A. Lewera and A. Wieckowski, Chem. Mater., 2004, 16, 4128–4134 CrossRef CAS.
-
(a) P. J. Kulesza, I. S. Pieta, I. A. Rutkowska, A. Wadas, D. Marks, K. Klak, L. Stobinski and J. A. Cox, Electrochim. Acta, 2013, 110, 474–483 CrossRef CAS PubMed;
(b) Y. Luo, A. Habrioux, L. Calvillo, G. Granozzi and N. Alonso-Vante, ChemPhysChem, 2014, 15, 2136–2144 CrossRef CAS PubMed.
- P. Kishore, B. Viswanathan and T. Varadarajan, Nanoscale Res. Lett., 2008, 3, 14–20 CrossRef CAS.
- R. Esfand and D. A. Tomalia, Drug Discovery Today, 2001, 6, 427–436 CrossRef CAS.
-
(a) C. Besson, Z. Huang, Y. V. Geletii, S. Lense, K. I. Hardcastle, D. G. Musaev, T. Lian, A. Proust and C. L. Hill, Chem. Commun., 2010, 46, 2784–2786 RSC;
(b) A. Sartorel, M. Carraro, G. Scorrano, R. D. Zorzi, S. Geremia, N. D. McDaniel, S. Bernhard and M. Bonchio, J. Am. Chem. Soc., 2008, 130, 5006–5007 CrossRef CAS PubMed.
- N. Anwar, A. Sartorel, M. Yaqub, K. Wearen, F. Laffir, G. Armstrong, C. Dickinson, M. Bonchio and T. McCormac, ACS Appl. Mater. Interfaces, 2014, 6, 8022–8031 CAS.
- M. Lira-Cantú and P. Gómez-Romero, J. Electrochem. Soc., 1999, 146, 2029–2033 CrossRef PubMed.
- K. Kastner, J. T. Margraf, T. Clark and C. Streb, Chem. – Eur. J., 2014, 20, 12269–12273 CrossRef CAS PubMed.
-
(a) A. Proust, R. Thouvenot, M. Chaussade, F. Robert and P. Gouzerh, Inorg. Chim. Acta, 1994, 224, 81–95 CrossRef CAS;
(b) Y. Du, A. L. Rheingold and E. A. Maata, J. Am. Chem. Soc., 1992, 114, 345–346 CrossRef CAS;
(c) W. Clegg, R. J. Errington, K. A. Fraser, S. A. Holmes and A. Schafer, J. Chem. Soc., Chem. Commun., 1995, 455–456 RSC;
(d) J. Kang, B. Xu, Z. Peng, X. Zhu, Y. Wei and D. R. Powell, Angew. Chem., Int. Ed., 2005, 44, 6902–6905 CrossRef CAS PubMed.
- Y. Zhu, L. Wang, J. Hao, Z. Xiao, Y. Wei and Y. Wang, Cryst. Growth Des., 2009, 9, 3509–3518 CAS.
- R. N. Nasim Khan, N. Mahmood, C. Lv, G. Sima, J. Zhang, J. Hao, Y. Hou and Y. Wei, RSC Adv., 2014, 4, 7374 RSC.
- H. Wang, S. Hamanaka, Y. Nishimoto, S. Irle, T. Yokoyama, H. Yoshikawa and K. Awaga, J. Am. Chem. Soc., 2012, 134, 4918–4924 CrossRef CAS PubMed.
- N. Kawasaki, H. Wang, R. Nakanishi, S. Hamanaka, R. Kitaura, H. Shinohara, T. Yokoyama, H. Yoshikawa and K. Awaga, Angew. Chem., Int. Ed., 2011, 50, 3471–3474 CrossRef CAS PubMed.
- K. Kume, N. Kawasaki, H. Wang, T. Yamada, H. Yoshikawa and K. Awaga, J. Mater. Chem. A, 2014, 2, 3801–3807 CAS.
-
(a) W. Chen, L. Huang, J. Hu, T. Li, F. Jia and Y.-F. Song, Phys. Chem. Chem. Phys., 2014, 16, 19668–19673 RSC;
(b) X.-J. Sang, J.-S. Li, L.-C. Zhang, Z.-M. Zhu, W.-L. Chen, Y.-G. Li, Z.-M. Su and E.-B. Wang, Chem. Commun., 2014, 50, 14678–14681 RSC.
- M.-Q. Li, Z.-G. Shao and K. Scott, J. Power Sources, 2008, 183, 69–75 CrossRef CAS PubMed.
- M. Góral-Kurbiel, A. Drelinkiewicz, R. Kosydar, J. Gurgul, B. Dembińska and P. Kulesza, J. Solid State Electrochem., 2014, 18, 639–653 CrossRef.
- S. W. S. R. Samms and R. F. Savinell, J. Electrochem. Soc., 1996, 143, 1225–1232 CrossRef CAS PubMed.
-
(a) J. A. Asensio, S. Borrós and P. Gómez-Romero, Electrochem. Commun., 2003, 5, 967–972 CrossRef CAS PubMed;
(b) J. A. Asensio, S. Borrós and P. Gómez-Romero, Electrochim. Acta, 2005, 50, 4715–4720 CrossRef PubMed.
- T. Mirfakhrai, J. D. W. Madden and R. H. Baughman, Mater. Today, 2007, 10, 30–38 CrossRef CAS.
- Y. Bahramzadeh and M. Shahinpoor, Soft Rob., 2013, 1, 38–52 CrossRef.
- L. Bay, K. West, P. Sommer-Larsen, S. Skaarup and M. Benslimane, Adv. Mater., 2003, 15, 310–313 CrossRef CAS.
- J. D. Madden, P. G. Madden and I. W. Hunter, Proc. SPIE., 2002, 4695, 176–190 CrossRef CAS PubMed.
- A. Proust, B. Matt, R. Villanneau, G. Guillemot, P. Gouzerh and G. Izzet, Chem. Soc. Rev., 2012, 41, 7605–7622 RSC.
- P. Mialane, A. Dolbecq, L. Lisnard, A. Mallard, J. Marrot and F. Sécheresse, Angew. Chem., Int. Ed., 2002, 114, 2504–2507 CrossRef.
- S. Liu, D. Volkmer and D. G. Kurth, Anal. Chem., 2004, 76, 4579–4582 CrossRef CAS PubMed.
- J. Xu, P. Yao, X. Li and F. He, Mater. Sci. Eng., B, 2008, 151, 210–219 CrossRef CAS PubMed.
- V. Goovaerts, K. Stroobants, G. Absillis and T. N. Parac-Vogt, Phys. Chem. Chem. Phys., 2013, 15, 18378–18387 RSC.
- D. Mercier, S. Boujday, C. Annabi, R. Villanneau, C.-M. Pradier and A. Proust, J. Phys. Chem. C, 2012, 116, 13217–13224 CAS.
- Y. Ji, T. Li and Y.-F. Song, Ind. Eng. Chem. Res., 2014, 53, 11566–11570 CrossRef CAS.
- Q. Chen, Y. Cui, J. Cao and B.-H. Han, Polymer, 2011, 52, 383–390 CrossRef CAS PubMed.
- H. Li, P. Li, Y. Yang, W. Qi, H. Sun and L. Wu, Macromol. Rapid Commun., 2008, 29, 431–436 CrossRef CAS.
|
This journal is © The Royal Society of Chemistry 2015 |