Biomimetic honeycomb-patterned surface as the tunable cell adhesion scaffold†
Received
5th July 2014
, Accepted 2nd August 2014
First published on 22nd August 2014
Abstract
Inspired by the typically adhesive behaviors of fish skin and Parthenocissus tricuspidata, two different decorations of polystyrene honeycomb membrane (PSHCM) prepared by the breath figure approach were carried out with poly(N-(3-Sulfopropyl)-N-(methacryloxyethyl)-N,N-dimethylammonium betaine)(polySBMA) to explore controllable bioadhesive surfaces. Casting and dip-coating were employed to graft polySBMA onto the plasma treated PSHCM. The polySBMA casted PSHCM showed a uniform covering layer on the PSHCM similar to the mucus layer of fish skin, presenting excellent antifouling properties. On the contrary, a dip-coated one showed the polySBMA aggregating on the honeycomb pore walls forming a large number of sucking disks such as the adhesive disks of the tendrils of P. tricuspidata, which remarkably boosts cell adhesion on substrates. Thus, bioadhesion could be regulated as desired by tuning the distribution of zwitterionic polymer on the honeycomb surface. The results may provide a new approach for the design of biomaterial surfaces.
Introduction
Natural and artificial biomaterials are characterized by micro- and nanoscale morphologies to respond to various physiological environments. For example, bone tissues need an appropriate surface topography to support cell adhesion, while and some organs or organ transplantations, especially blood-contacted materials such as intravascular stents, need a certain degree of roughness to prevent biofouling.1–5 Thus, in the context of tissue repair and regeneration, an appropriate topological surface is an essential requirement for biomaterials.6,7
To investigate the adhesive behaviours between proteins/cells and surfaces, topological surfaces that mimic the natural ones have recently attracted significant interest.8–10 Different morphologies, such as grooves, pits, and pillars, have been investigated.11–15 The morphological parameters that affect cellular behaviours, including the density, shape and size, have been systematically studied.11,13,16 It has become increasingly apparent that a rough surface can stimulate cell adhesion and encourage cell differentiation.17 However, most abovementioned morphologies require elaborate and complex preparation.18,19 Hence, it makes sense to develop a more convenient method to fabricate surface topographies. Breath figure as a water-driven template method has been utilized to prepare ordered hexagonally arranged pores, the so-called honeycomb structure.20–25 Compared with traditional patterning techniques, such as microcontact printing and dip-pen lithography, breath figure avoids specialized machinery or specifically designed templates, allowing the control of the structural properties.26,27 For the porous character of honeycomb structure, various cells, such as hepatocyte, fibroblast, stem cell, have been widely investigated.28–33 It has been demonstrated that the honeycomb structure can significantly affect cell adhesion, spreading and differentiation. In microscale aspect, the micropatterning protein has been successfully fabricated on the honeycomb structures.34,35 However, to the best of our knowledge, the tunable bioadhesion based on honeycomb surface has not hitherto been reported.
Apart from the topological influence, chemical component is another vital factor that affects the bioadhesive behaviours.36 Surface modification with hydrophilic polymers, such as poly(ethylene glycol) (PEG), poly(hydroxyethyl methacrylate) (PHEMA), poly(oligo(ethylene glycol) methacrylate) (POEGMA), has proved to be an efficient approach for changing the adsorption properties of nonspecific proteins.37–39 Recently, zwitterionic polymers have received significant attention because of the stability of their surface properties and high efficiency as antifouling materials.40–42 Cellular attachment, spreading, and protein adsorption were resisted on the zwitterionic polymer surface, demonstrating that super-low fouling ability had been achieved.
In nature, several unique skills of animals and plants benefit from the coordination of surface components and microstructures on their bodies.43,44 It is well-known that the smooth and soft mucus layer on fish surface endows its skin with prominent antifouling ability, regardless of skin topologies.44,45 Thus, a promising strategy to decrease biocontamination is to obtain a smooth hydrophilic layer. Whereas the self-clinging P. tricuspidata can climb on house outside walls with tendrils to obtain vertical growth. At the end of the tendrils, a numerous adhesive disks exist with porous surface appearance, through which the suction force was provided. The adhesive disks secrete a heavy sticky fluid when stimulated, and the chemical anchor of the sticky fluid and the negative pressure of the adhesive disks make themselves attach quite firmly to house walls.46,47
Inspired by nature, a tunable bioadhesion surface based on honeycomb structure was explored in this work. A honeycomb structure prepared by the breath figure technique was decorated with zwitterionic polymer. Two different microdistributions of the zwitterionic polymer (polySBMA) on the honeycomb surface were obtained, namely the fish surface or tendril-like figures. The results of bioadhesion experiments showed that the two figures presented different adhesion behaviors. Antifouling surface as fish scales and highly adhesive surface as tendrils of P. tricuspidata demonstrated that the bioadhesion properties of honeycomb-patterned surfaces could be tunable by controlling the distributions of zwitterionic polymer.
Experimental
Materials
Polystyrene (PS, Mw = 250k) was purchased from Acros Reagents. N-(3-Sulfopropyl)-N-(methacryloxyethyl)-N,N-dimethylammonium betaine (SBMA, 97%) was obtained from Sigma-Aldrich. Rhodamine B (Rh-B, 95%) was purchased from J&K Chemical Ltd. Toluene and other reagents were obtained from Sinopharm Chemical Reagent Co. HeLa cells were obtained from the Cell Resource Centre of Life Sciences in Shanghai. Bovine serum albumin (BSA, 66 kD, >98%), fetal bovine serum (FBS), and Dulbecco's modified Eagle medium (DMEM) were obtained from Gibco BRL. Cellular staining dyes and all other cell culture reagents were purchased from the Beyotime Institute of Biotechnology. Water used in the experiments was purified with a Hitech system to achieve a resistivity of above 18.2 MΩ cm.
Preparation of PS honeycomb membrane
PS honeycomb membranes were prepared through a typical breath figure approach.22 First, a 10 × 10 mm glass slide was treated with Piranha solution at 120 °C for 30 min to remove impurities. PS was dissolved in toluene at a concentration of 1 mg mL−1, and 40 μL of this solution was coated onto the pretreated glass with humid air (approximately 70% R.H., 4 L min−1) flowing across the surface in a home-made chamber. All the processes were performed at room temperature. As a comparison, a flat PS membrane was prepared by solvent self-evaporation in a dry chamber.
Surface modification of the PS honeycomb membranes
The PS honeycomb membranes were exposed to O2 plasma at room temperature and a pressure of 40 Pa for 3 min using an SY-DT01 plasma apparatus (Suzhou OPS Plasma Technology, Co.), which endowed them with surface radicals. The surface modification procedure is shown schematically in Fig. 1. Two methods were then utilized for the grafting of polySBMA, namely casting and dip-coating. The casting process was as follows: 20 μL of a 10 wt% aqueous SBMA solution was directly cast onto the surface by a syringe. A UV-induced grafting technique was then applied to accelerate the reaction of SBMA with the surface radicals. A UV light generator (Intelli-ray 600, China) was set at 365 nm with energy 35 Mw cm−2, and the exposure time was limited to 5 min.
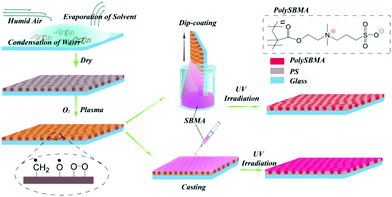 |
| Fig. 1 Schematic illustration of the fabrication process of zwitterionic polySBMA grafted onto the honeycomb PS membrane: a highly ordered honeycomb membrane was prepared by the breath figures method; plasma treatment was then applied to generate surface radicals. The SBMA solution was introduced onto the membrane by casting and dip-coating. Finally, UV irradiation was utilized to enhance the grafting reaction of polySBMA. | |
Dip-coating treatment was performed in a dip-coater (Panasonic, Japan); the dipping time and dragging speed were optimized at 5 min and 10 cm min−1, respectively. After dip-coating of SBMA, the membranes were irradiated with UV light for 1 min with energy 35 Mw cm−2. For convenience, the two modified membranes were named as casting and dip-coating membranes, respectively.
Characterizations
The chemical properties of the membranes were characterized by Fourier-transform infrared spectroscopy (FTIR, Perkin-Elmer, Spectrum 100, USA), attenuated total reflectance Fourier-transform infrared spectroscopy (ATR-FTIR) and X-ray photoelectron spectroscopy (XPS, PHI-5000 Versaprobe II, Japan). To avoid the interference of the glass slide, all the membranes were peeled from the glass slides and attached onto a KBr tablet, and then submitted to FTIR measurement. The scanning area of XPS was 200 × 200 μm. Scanning electron microscopy (SEM) images were acquired using an FEI Nova NanoSEM (FEI, USA). The swollen morphologies of the membranes under water were imaged using a Leica DM 4000 optical microscope (OM) equipped with a CCD camera (Leica, Germany). In addition, Rh-B labelling was applied to track the distribution of polySBMA. The membranes were first immersed in 10 μg mL−1 of Rh-B for 1 h at room temperature, then observed with an inverted fluorescence microscope (IX 71, Olympus) equipped with a CCD camera. The wettability measurement was performed on an OCA 20 apparatus (Dataphysics, Germany).
Protein adsorption
For the adsorption of BSA, membranes were first rinsed five times with phosphate-buffered saline (PBS, pH 7.4) and then immersed in PBS for 12 h to achieve swelling equilibrium. The membranes were then transferred to a 24-well plate and incubated with 1 mL of a 5 mg mL−1 solution of BSA in PBS for 2 h at 37 °C. Subsequently, the membranes were transferred to a new plate filled with 1 mL of PBS containing 1 wt% Triton X-100. The adsorbed proteins were desorbed by sonication for 20 min. The protein concentration was quantitatively measured by a bicinchoninic acid protein assay (BCA). For this, 20 μL of desorbed protein solution was mixed with 200 μL of BCA reagent in a 96-well plate and incubated for 30 min at 37 °C. Finally, light absorbance at a wavelength of 570 nm was determined using a microplate reader. The absorbance of each sample was measured six times. A standard curve was then plotted. In addition, the surface morphology of the membranes after the adsorption of the protein was detected by atom force microscopy (AFM, BioScope, VEECO).
Cell culture
The membranes were sterilized with medicinal alcohol and exposed to UV light for 24 h, and then placed in a 24-well plate and rinsed with PBS (2 × 1 mL). HeLa cells were cultured in DMEM with 10% FBS and 1% penicillin-streptomycin, seeded at a density of 4 × 104 cells per well, and incubated at 37 °C in atmosphere containing 5% CO2 for 24 h. The membranes with adhered cells were then rinsed twice with PBS to remove unattached cells. The cell viability was assessed by dead/live (AO/EB) double staining. An inverted fluorescence microscope (IX 71, Olympus) equipped with a CCD camera was used for cell imaging. Cell counting and image overlay were carried out with Image J software. SEM was also used for cellular morphology analysis. Cells were fixed with 2.5% glutaraldehyde solution for 20 min and then dehydrated with graded ethanol solutions. Samples were sputtered with Pt and examined with an FEI Nova NanoSEM 450 (FEI, USA).
Results and discussion
Chemistry and wettability of membranes
FTIR was applied to characterize the zwitterionic polymer decorated honeycomb membranes, as shown in Fig. 2. The bands at ν = 1180 and 1020 cm−1 can be assigned to the asymmetric and symmetric vibrations of the sulfonyl group (–SO3), and the peak at 1720 cm−1 is the characteristic peak of carbonyl group (C
O); both the decorated membranes (c and d) exhibited the characteristic bands of polySBMA, confirming the successful grafting of the zwitterionic polymer. Moreover, an obvious difference in the intensity of the two characteristic peaks, where the stretching vibration peak of C
C in the benzene ring (ν = 1490 cm−1) is chosen as a reference, could be observed in the spectra of the two decorated membranes: the peak intensity in the spectrum of the casting membrane was stronger than that in the spectrum of the dip-coating membrane, implying a difference in SBMA grafting density. The same results were also observed in the ATR-FTIR spectrum (Fig. S1†).
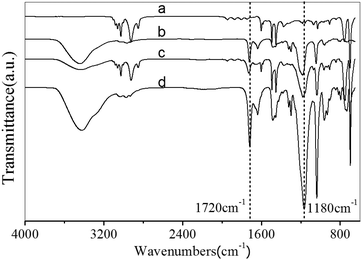 |
| Fig. 2 FTIR spectra of the membranes: (a) spectrum of the PS honeycomb membrane without modification, (b) spectrum of the SBMA monomer, and zwitterionic polymer-modified membranes obtained by (c) dip-coating and (d) direct casting. | |
In addition, N, S, and O atoms could be observed in the XPS spectra of both the dip-coating and casting PSHCM, demonstrating the successful grafting of polySBMA on the membranes (Fig. 3). The peak information is summarized in Table S1.†
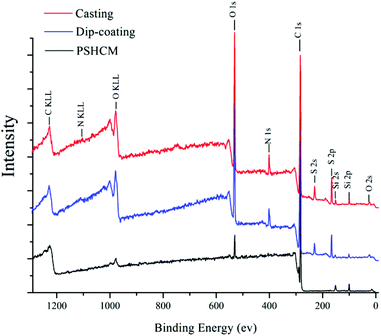 |
| Fig. 3 XPS spectra of the membranes. Red: the casting membrane; blue: the dip-coating membrane; black: PSHCM. | |
The wettability was evaluated by static contact-angle measurement. As shown in Fig. 3a, the water contact angles on the undecorated flat surface and honeycomb surface were 97.2° and 106.2°, respectively. According to the Wenzel theory, a rough surface can increase the hydrophobicity of a hydrophobic surface (water contact angle greater than 90°) and the hydrophilicity of a hydrophilic surface (water contact angle less than 90°), honeycomb structure endowed PS surface with a surface roughness. Thus, honeycomb holes enhanced the hydrophobicity of the membrane.48 After decoration with polySBMA, the contact angles of the casting and dip-coating membranes were 30.5° and 25°, respectively. The slight difference in the wettability of the casting and the dip-coating membranes was caused by different surface structures, as shown in Fig. 5b, c. The surface of the dip-coating membrane still retained a porous structure, whereas that of the casting membrane was fully covered with polySBMA.
The surface energy of the membranes was calculated via the OWRK method, based on the contact angle results of water and diiodomethane (ESI†). The results are shown in Fig. 4b. The undecorated PS membrane exhibited low surface energy; after the grafting of polySBMA, the surface energy increased from 40 mN m−1 to approximately 70 mN m−1. Moreover, there was a 17% increase in the surface energy of the dip-coating membrane compared with the casting membrane. The difference in surface energy between casting and dip-coating membranes plays an important role in the process of protein adsorption and cell adhesion (vide infra).
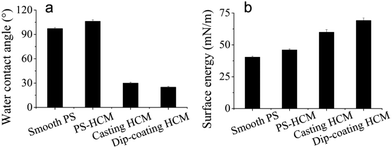 |
| Fig. 4 Static water contact angles and surface energies of the membranes. Smooth PS and PSHCM represent the PS flat and PS honeycomb membranes, casting HCM denotes the polySBMA-modified honeycomb PS membrane obtained by casting, and dip-coating HCM denotes the polySBMA-modified honeycomb PS membrane obtained by dip-coating. | |
Morphologies of the membranes
The morphologies of the membranes were first characterized by SEM, as shown in Fig. 5a–c. Fig. 5a shows an ordered honeycomb membrane. When SBMA was casted onto the membrane (Fig. 5b), the entire pores of PSHCM were filled with polySBMA because of a sufficient amount of reactive monomers, and the membrane became smoother than that before casting treatment, indicative of total coverage of the honeycomb structure with a uniform polySBMA layer. The cross section SEM of this membrane further confirmed this result. By contrast, polySBMA aggregatively grafted around the wall of a honeycomb hole by dip-coating (Fig. 5c) attributed to the capillary force (Fig. S2†). The complete figures of cross-section SEM photographs are provided in Fig. S3.† The phenomena of honeycomb structure being filled with polySBMA by casting and would exist after dip-coating can be further demonstrated by AFM (Fig. S4†).
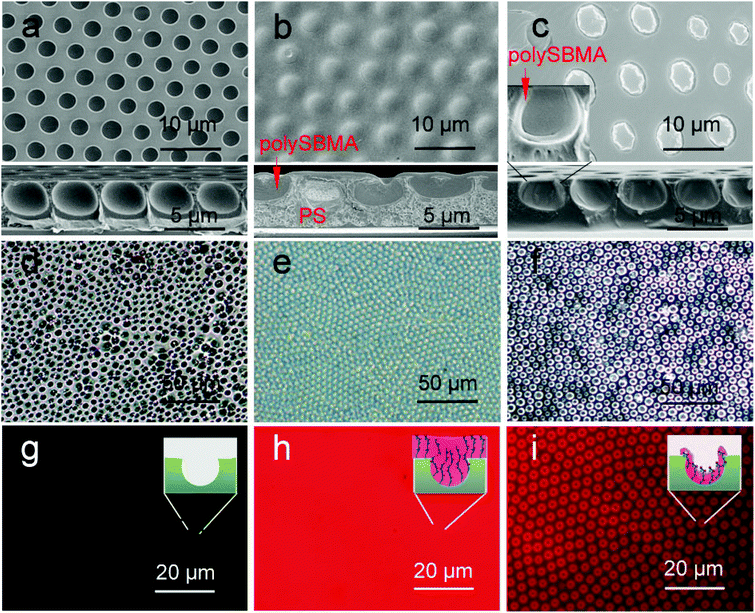 |
| Fig. 5 SEM, OM, and fluorescence photographs of honeycomb membranes: (a–c) SEM images; the inserted photographs are the correspondent cross-section SEM, the whole figure shown in Fig. S3.† (d–f) OM photographs in water; (g–i) fluorescence images of membranes after labelling with Rhodamine; (a), (d), and (g) honeycomb membrane without modification; (b), (e), and (h) casting membrane; (c), (f), and (i) dip-coating membrane. | |
In general, the process of membranes interacting with proteins or cells is performed in aqueous media, and the swollen morphologies of the membranes directly influence cellular behavior.49 To characterize the swollen morphologies of decorated membranes in water, we utilized an optical microscope (OM) to observe the morphologies of the membranes. As shown in Fig. 5d, the honeycomb PS membrane was hydrophobic and could not be significantly infiltrated. Water could not enter its pores and formed several liquid film caps over the membrane. The casting membrane on which PSHCM was fully covered with polySBMA displayed distinct swelling behaviour, namely forming a hydration layer. The dip-coating membrane still contained the honeycomb porous structure. However, the polySBMA aggregated around the pore walls of the honeycomb, and only these domains can swell in water. An ordered array of zwitterionic polymer rings was formed. Because the hydrated layer is transparent, it is difficult to distinguish the difference between the two samples just using OM.
For this, the fluorescent labelling method was applied to characterize the distribution of zwitterionic polymer on the surface. In this work, a water-soluble Rh-B with an excitation wavelength of 552 nm was selected to label the polySBMA on the surface. The zwitterionic polymer contained a large number of ammonium (–NH2+–) and sulfonic (–SO3−) groups, which could combine with the fluorescent dye. In contrast, the PS does not interact with Rh-B. Thus the Rh-B labelling can be applied to exhibit the distribution of polySBMA. The results are shown in Fig. 5g–i. The undecorated honeycomb membrane could not interact with Rh-B and thus could not emit fluorescence showing a dark image (Fig. 5g). However, the casting membrane exhibited a uniform fluorescence emission. This phenomenon suggested that the honeycomb structure had been fully covered with polySBMA (Fig. 5h). The dip-coating membrane emitted fluorescence only around the pores, indicating the enrichment of polySBMA in the pore (Fig. 5i). Namely, the polySBMA aggregated around the honeycomb structure. In this case, the different decoration procedures resulted in different distributions of the zwitterionic polymer. These composite architectures could then be utilized to investigate the interactions between cells and these surfaces.
Protein adsorption
The adhesion of cells essentially corresponded to the adsorption of proteins because the extracellular matrix (ECM) is made up of all kinds of proteins.50 To study cellular behaviours on the membranes, the adsorption of BSA was utilized to investigate the interactions between proteins and surfaces.
It is well-known that hydrophobic surfaces benefit protein adsorption.51 An effective method for reducing the nonspecific adsorption of proteins is to improve the wettability of the surfaces. In this work, the original PSHCM surface was hydrophobic but was rendered hydrophilic by decoration with the zwitterionic polymer. Fig. 6a–e shows the AFM images of the membranes after the adsorption of BSA. Both the smooth and honeycomb PS membranes showed obvious protein adsorption, which can be attributed to their hydrophobicity. When the PS surfaces with or without honeycomb structures were decorated by grafting the zwitterionic polymer by casting, they exhibited repelling protein fouling. Two basic mechanisms of antifouling, namely steric repulsion and hydration theory, have been proposed.41,52 The former considers that hydrophilic polymers bind with water through hydrogen bonds and swell, and the swollen polymer acts as a barrier that prevents proteins from attaching to the substrates; the hydration theory regards the hydration layer as an excellent antifouling barrier because the displacement of bound water (icelike water) molecules constitutes a major barrier in the adsorption and adhesion phenomena to highly hydrophilic surfaces.53 In our works, the zwitterionic polymer layer by casting can strongly bond with water through hydrogen bonds and electrostatic interactions and form a thick hydration layer. The thick zwitterionic polymer-water layer looks like fish scales efficiently reducing the adsorption of proteins.
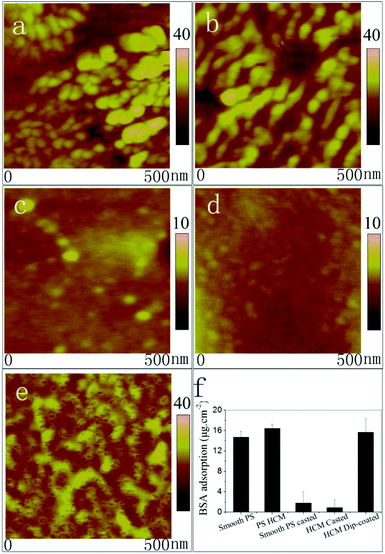 |
| Fig. 6 AFM photographs of membranes after the adsorption of BSA: (a) smooth flat PS; (b) PS honeycomb membrane; (c) polySBMA-modified flat PS obtained by casting; (d) polySBMA-modified membrane obtained by casting, and (e) polySBMA-modified membrane obtained by dip-coating. (f) The results of BSA protein adsorption experiments measured by the BCA method: smooth PS is the PS flat surface, PSHCM, smooth PS casted the polySBMA grafted PS flat, HCM casted is the casting membrane, and HCM dip-coated is the dip-coating membrane. | |
A significant increase in protein adsorption was observed on the dip-coating membrane, as shown in Fig. 6e. To quantitatively compare protein adsorptions on the different membranes, the BCA assay was used to detect the quantity of BSA adsorption (Fig. 6f). Protein adsorption on the hydrophobic PSHCM surface was as high as 15 g cm−2 but decreased when the surface was covered with the zwitterionic polymer by casting. However, the BSA adsorption on the dip-coating membrane maintained a high level. As shown in Fig. 5c and f, we can see that polySBMA aggregated mostly around the pore walls, forming sucker disks such as the adhesive disks of the tendril of self-clinging P. tricuspidata. The distribution of the zwitterionic polymer on the honeycomb surface prevented the formation of a uniform hydration layer. Moreover, the surface of the honeycomb structure partially retained the properties of uncovered PS, which imparted high surface energy because the PS surface was treated with O2 plasma before grafting polySBMA. The sucker disk structure with high surface energy caused the proteins to undergo conformational changes and entropy gained upon adsorption on solid surfaces,54 and the protein affinity on the dip-coating surface was clearly higher than that on the casting membrane. Moreover, the protein adsorption on the PSHCM was different from that on the dip-coating membrane. Because of hydrophobic interaction, the protein on PSHCM was physically adsorbed, as shown in Fig. 6b and e.
Cell adhesion
The HeLa cells adhered on the membranes were counted based on the fluorescence images. As shown in Fig. 7, the cell density on the PS honeycomb was 53 cells mm−2. Few cells were found on the polySBMA-covered surface. Compared with the casting membrane, the dip-coating showed excellent cell adhesion (443 cells mm−2). Thus, a remarkable phenomenon was described that the PSHCM could be modulated into a cell-phobic or cell-philic state by regulating the surface distribution of polySBMA on the honeycomb membrane.
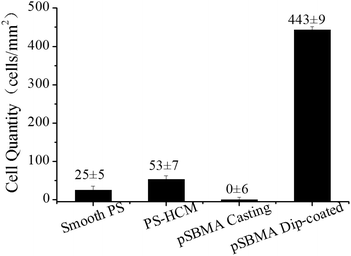 |
| Fig. 7 Adhesion densities of HeLa cells on the surfaces after culturing for 24 h. | |
To acquire more detailed information about the HeLa cells on the polySBMA decorated PS honeycomb, the cells were fixed with glutaraldehyde and observed by SEM. For comparison, the SEM images of the cells and the corresponding fluorescence images are shown in Fig. 8. The cells adhered on PSHCM were mostly tower shaped and formed visible lamellipodia. Because the PSHCM was hydrophobic, a further spreading of cells is seldom observed. Note that no cell can be observed on the casting membrane due to the hydration layer.
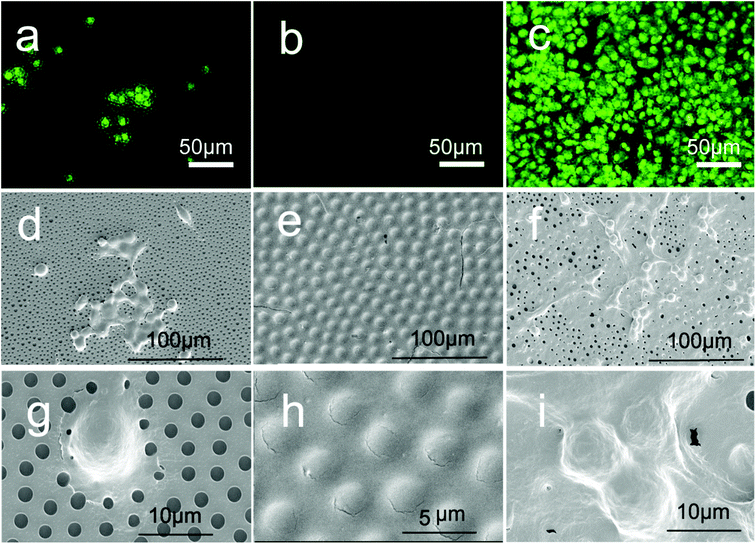 |
| Fig. 8 AO/EB double-staining fluorescence images and SEM photographs of HeLa cells on the membranes. (a–c) Fluorescence images; (d–i) SEM photographs of HeLa cell adhesion on the membranes, in which (a), (d), and (g) show the honeycomb PS membrane, (b), (e), and (h) show the polySBMA cast membranes, and (c), (f), and (i) show the polySBMA dip-coated membranes. | |
However, on the dip-coating membrane, the cells clearly spread and the protrusions of different cells interconnected. The honeycomb pores were almost covered with the cell protrusions, and the cells were firmly immobilized on the dip-coated PSHCM. In contrast to the casting membrane, the polySBMA patterned surface was cell-philic because of the lack of a hydration layer.
To understand the cellular behaviour on the surfaces, we postulated that there were two possible ways of attaching cells onto the zwitterionic polymer decorated surfaces, as shown in Fig. 9. As mentioned above, polySBMA has already covered the honeycomb structure on the casting membrane and uniformly formed a hydration layer.55 In addition, the hydration layer is just like the mucus layer on fish scales, which prevented proteins and cells from attaching to the surface.
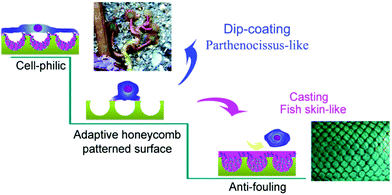 |
| Fig. 9 Schematic illustration of cell behaviors on the different membranes. Cells on the undecorated HCM surface were spherical in which spreading was restricted; then two different decorations of polySBMA were processed on it, namely dip-coating and casting. The dip-coating PSHCM endowed PSHCM with a Parthenocissus sucker-like topological surface, resulting in a cell-philic state, whereas a confluent polySBMA layer formed on the casting one, which can form a hydration layer to prevent the cells from adhering, which was inspired by the fish surfaces. | |
The polySBMA only aggregated around the pore walls and formed sucker disks similar to the adhesive disks on the tendrils of P. tricuspidata. The structured membranes not only hindered the formation of a uniform hydration layer but also provided favourable conditions for both protein adsorption and cell adhesion. In addition, the cavities of the honeycomb structure can provide negative pressure.56 It is hard for a cell to escape from the dip-coating membranes after the cells cover the honeycomb surface. Thus, the cells were stabilized on the dip-coating surface. In contrast, the honeycomb structure without zwitterionic polymer decoration was hydrophobic and showed a higher level of protein adsorption. Moreover, the cytomembrane is hydrophilic; an appropriately hydrophilic surface is required for the permeation of culture medium and the growth of the cell.57 The hydrophobicity of the PSHCM restricted the spreading of the cells (Fig. 9).
Based on the abovementioned results, a simple pattern on the surface was created to prove the controllable cellular adhesion on the modified membrane: the honeycomb membrane with cast polySBMA was selected as the substrate and then a channel was prepared with a needle. The cells were seeded onto the membrane. The results are shown in Fig. 10 and provide clear evidence that the area of the PS honeycomb membranes completely covered with polySBMA had antifouling ability. The HeLa cells adhered selectively in the channel, where substrate was not covered with polySBMA.
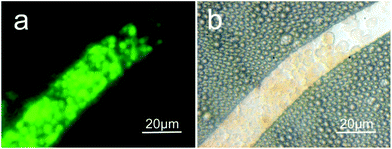 |
| Fig. 10 AO/EB double-staining fluorescence images of cells on a designed PSHCM model. The PSHCM was coated with polySBMA by casting, and then a channel was cut with a needle. | |
Conclusions
Inspired by nature, tunable bioadhesive membranes, including highly adhesive and antifouling surface, were prepared based on a PS honeycomb structure. Two methods have been applied to graft polySBMA onto the surface, namely direct casting and dip-coating, which resulted in different bioadhesive behaviors. The casting membrane showed super-low protein- and cell-binding properties. In contrast, on the dip-coating membrane, most of the polySBMA aggregated around the pore walls. In this case, the surface of the PSHCM membrane was protein- and cell-philic. Because of the array of pores, the breath figure method offers a facile route to mimic biosurfaces. Here, we have decorated the honeycomb surface to render it either cell-phobic or cell-philic by regulating the grafting of polySBMA, and our results may be applicable to the design of several biomedical surfaces.
Acknowledgements
This work was supported by Major Program of Chinese National Programs for Fundamental Research and Development (973 project: 2012CB933803, 2014CB643605); National Science Fund for distinguished Young Scholars (50925310), The National Science Foundation of China (51173103, 21374060), and the Excellent Academic Leaders of Shanghai (11XD1403000).
Notes and references
- L. E. Niklason and R. S. Langer, Transpl. Immunol., 1997, 5, 303–306 CrossRef CAS.
- K. J. L. Burg, S. Porter and J. F. Kellam, Biomaterials, 2000, 21, 2347–2359 CrossRef CAS.
- J. Nikolovski and D. J. Mooney, Biomaterials, 2000, 21, 2025–2032 CrossRef CAS.
- M. P. Lutolf and J. A. Hubbell, Nat. Biotechnol., 2005, 23, 47–55 CrossRef CAS PubMed.
- G. D. Prestwich, S. Bhatia, C. K. Breuer, S. L. M. Dahl, C. Mason, R. McFarland, D. J. McQuillan, J. Sackner-Bernstein, J. Schox, W. E. Tente, A. Trounson, C. K. Breuer and A. Trounson, Sci. Transl. Med., 2012, 4, 6 Search PubMed.
- D. G. Anderson, J. A. Burdick and R. Langer, Science, 2004, 305, 1923–1924 CrossRef CAS PubMed.
- M. E. Furth, A. Atala and M. E. Van Dyke, Biomaterials, 2007, 28, 5068–5073 CrossRef CAS PubMed.
- F. Xia and L. Jiang, Adv. Mater., 2008, 20, 2842–2858 CrossRef CAS.
- R. G. Flemming, C. J. Murphy, G. A. Abrams, S. L. Goodman and P. F. Nealey, Biomaterials, 1999, 20, 573–588 CrossRef CAS.
- C. M. Preuss, T. Tischer, C. Rodriguez-Emmenegger, M. M. Zieger, M. Bruns, A. S. Goldmann and C. Barner-Kowollik, J. Mater. Chem. B, 2014, 2, 36–40 RSC.
- D. J. Kim, J. K. Seol, G. Lee, G. S. Kim and S. K. Lee, Nanotechnology, 2012, 23, 9 Search PubMed.
- X. Yao, R. Peng and J. Ding, Adv. Mater., 2013, 25, 5257–5286 CrossRef CAS PubMed.
- C. S. Chen, M. Mrksich, S. Huang, G. M. Whitesides and D. E. Ingber, Science, 1997, 276, 1425–1428 CrossRef CAS.
- W. Ye, Q. Shi, S. C. Wong, J. W. Hou, H. C. Shi and J. H. Yin, Macromol. Biosci., 2013, 13, 676–681 CrossRef CAS PubMed.
- Y. Ito, Biomaterials, 1999, 20, 2333–2342 CrossRef CAS.
- A. S. Sarvestani, Soft Matter, 2013, 9, 5927–5932 RSC.
- H. L. Fan, P. P. Chen, R. M. Qi, J. Zhai, J. X. Wang, L. Chen, L. Chen, Q. M. Sun, Y. L. Song, D. Han and L. Jiang, Small, 2009, 5, 2144–2148 CrossRef CAS PubMed.
- L. B. Koh, I. Rodriguez and S. S. Venkatraman, Biomaterials, 2010, 31, 1533–1545 CrossRef CAS PubMed.
- R. S. Kane, S. Takayama, E. Ostuni, D. E. Ingber and G. M. Whitesides, Biomaterials, 1999, 20, 2363–2376 CrossRef CAS.
- M. Hernandez-Guerrero and M. H. Stenzel, Polym. Chem., 2012, 3, 563–577 RSC.
- H. M. Ma and J. C. Hao, Chem. Soc. Rev., 2011, 40, 5457–5471 RSC.
- P. Escale, L. Rubatat, L. Billon and M. Save, Eur. Polym. J., 2012, 48, 1001–1025 CrossRef CAS PubMed.
- J. R. McMillan, M. Akiyama, M. Tanaka, S. Yamamoto, M. Goto, R. Abe, D. Sawamura, M. Shimomura and H. Shimizu, Tissue Eng., 2007, 13, 789–798 CrossRef CAS.
- T. Kawano, Y. Nakamichi, S. Fujinami, K. Nakajima, H. Yabu and M. Shimomura, Biomacromolecules, 2013, 14, 1208–1213 CrossRef CAS PubMed.
- Y. Fukuhira, E. Kitazono, T. Hayashi, H. Kaneko, M. Tanaka, M. Shimomura and Y. Sumi, Biomaterials, 2006, 27, 1797–1802 CrossRef CAS PubMed.
- Z. H. Nie and E. Kumacheva, Nat. Mater., 2008, 7, 277–290 CrossRef CAS PubMed.
- B. W. Muir, A. Fairbrother, T. R. Gengenbach, F. Rovere, M. A. Abdo, K. M. McLean and P. G. Hartley, Adv. Mater., 2006, 18, 3079–3081 CrossRef CAS.
- T. Kawano, M. Sato, H. Yabu and M. Shimomura, Biomater. Sci., 2014, 2, 52 RSC.
- L. Heng, R. Hu, S. Chen, M. Li, L. Jiang and B. Z. Tang, Langmuir, 2013, 29, 14947–14953 CrossRef CAS PubMed.
- J. R. McMillan, M. Akiyama, M. Tanaka, S. Yamamoto, M. Goto, R. Abe, D. Sawamura, M. Shimomura and H. Shimizu, Tissue Eng., 2007, 13, 789–798 CrossRef CAS.
- A. Sanz de Leon, J. Rodriguez-Hernandez and A. L. Cortajarena, Biomaterials, 2013, 34, 1453–1460 CrossRef CAS PubMed.
- D. K. S. Choy, V. D. W. Nga, J. Lim, J. Lu, N. Chou, T. T. Yeo and S.-H. Teoh, Tissue Eng., Part A, 2013, 19, 2382–2389 CrossRef CAS PubMed.
- M. A. Birch, M. Tanaka, G. Kirmizidis, S. Yamamoto and M. Shimomura, Tissue Eng., Part A, 2013, 19, 2087–2096 CrossRef CAS PubMed.
- Y. Zhang and C. Wang, Adv. Mater., 2007, 19, 913–916 CrossRef CAS.
- E. Min, K. H. Wong and M. H. Stenzel, Adv. Mater., 2008, 20, 3550–3556 CrossRef CAS.
- C. Duan, J. Gao, D. Zhang, L. Jia, Y. Liu, D. Zheng, G. Liu, X. Tian, F. Wang and Q. Zhang, Biomacromolecules, 2011, 12, 4335–4343 CrossRef CAS PubMed.
- Z. Zhang, J. Ni, L. Chen, L. Yu, J. W. Xu and J. D. Ding, Biomaterials, 2011, 32, 4725–4736 CrossRef CAS PubMed.
- X. J. Shi, Y. Y. Wang, D. Li, L. Yuan, F. Zhou, Y. W. Wang, B. Song, Z. Q. Wu, H. Chen and J. L. Brash, Langmuir, 2012, 28, 17011–17018 CrossRef CAS PubMed.
- D. J. Menzies, A. Cameron, T. Munro, E. Wolvetang, L. Grondahl and J. J. Cooper-White, Biomacromolecules, 2013, 14, 413–423 CrossRef CAS PubMed.
- Z. Zhang, M. Zhang, S. F. Chen, T. A. Horbetta, B. D. Ratner and S. Y. Jiang, Biomaterials, 2008, 29, 4285–4291 CrossRef CAS PubMed.
- Z. Zhang, T. Chao, S. F. Chen and S. Y. Jiang, Langmuir, 2006, 22, 10072–10077 CrossRef CAS PubMed.
- J. Ladd, Z. Zhang, S. Chen, J. C. Hower and S. Jiang, Biomacromolecules, 2008, 9, 1357–1361 CrossRef CAS PubMed.
- M. J. Hancock, K. Sekeroglu and M. C. Demirel, Adv. Funct. Mater., 2012, 22, 2223–2234 CrossRef CAS PubMed.
- M. L. Carman, T. G. Estes, A. W. Feinberg, J. F. Schumacher, W. Wilkerson, L. H. Wilson, M. E. Callow, J. A. Callow and A. B. Brennan, Biofouling, 2006, 22, 11–21 CrossRef CAS PubMed.
- D. W. Bechert, M. Bruse and W. Hage, Exp. Fluids, 2000, 28, 403–412 CrossRef.
- H. Tianxian, Z. Li and D. Wenli, Mater. Chem. Phys., 2011, 131, 23–26 CrossRef PubMed.
- D. Wenli, Nature Precedings, 2008 Search PubMed , http://hdl.handle.net/10101/npre.2008.1513.1.
- T. Sun, L. Feng, X. Gao and L. Jiang, Acc. Chem. Res., 2006, 39, 487–487 CrossRef CAS.
- E. Cukierman, R. Pankov, D. R. Stevens and K. M. Yamada, Science, 2001, 294, 1708–1712 CrossRef CAS PubMed.
- S. Schlie-Wolter, A. Ngezahayo and B. N. Chichkov, Exp. Cell Res., 2013, 319, 1553–1561 CrossRef CAS PubMed.
- C. Fairman, J. Z. Ginges, S. B. Lowe and J. J. Gooding, ChemPhysChem, 2013, 14, 2183–2189 CrossRef CAS PubMed.
- S. F. Chen, L. Y. Li, C. Zhao and J. Zheng, Polymer, 2010, 51, 5283–5293 CrossRef CAS PubMed.
- L. Petrone, Adv. Colloid Interface Sci., 2013, 195–196, 1–18 CrossRef CAS PubMed.
- M. Rabe, D. Verdes and S. Seeger, Adv. Colloid Interface Sci., 2011, 162, 87–106 CrossRef CAS PubMed.
- S. Chen and S. Jiang, Adv. Mater., 2008, 20, 335–338 CrossRef CAS.
- L. Heng, X. Meng, B. Wang and L. Jiang, Langmuir, 2013, 29, 9491–9498 CrossRef CAS PubMed.
- E. A. Evans and D. A. Calderwood, Science, 2007, 316, 1148–1153 CrossRef CAS PubMed.
Footnote |
† Electronic supplementary information (ESI) available. See DOI: 10.1039/c4bm00233d |
|
This journal is © The Royal Society of Chemistry 2015 |