A novel pyrene-based two-photon active fluorescent dye efficiently excited and emitting in the ‘tissue optical window (650–1100 nm)’†
Received
23rd August 2014
, Accepted 17th October 2014
First published on 17th October 2014
Abstract
The development of two-photon (TP) active fluorophores remains an important issue. Dyes that can be excited and fluoresce efficiently in the ‘tissue optical window’ (650–1100 nm) are especially in demand to maximize the underlying performance of two-photon fluorescence microscopy (TPFM) as an advanced optical technique. Ideally, such dyes would be compatible with the 1050 nm femtosecond fibre laser, which has recently been developed as an inexpensive excitation source to make the TPFM technique universal. In this work, we designed and synthesized a novel pyrene-based acceptor–π–acceptor (A–π–A) dye, PY, which exhibited outstanding properties such as bright fluorescence (λem = 650 nm and ΦFL = 0.80) and a large two-photon absorption cross-section (1100 GM (1 GM = 10−50 cm4 per photon per molecule) at 950 nm and 380 GM at 1050 nm) in the tissue optical window. In living mitochondria, PY provided more sensitive microscopic images than current dyes and showed great potential to be a building block of TP active fluorescent probes for the 1050 nm fibre laser. We believe that the exceptional properties of PY will be extended to other fluorescent probes through further chemical modification.
Introduction
Two-photon fluorescence microscopy (TPFM) has received considerable attention as an advanced optical technique, especially in the biomedical field, because it can provide both in vivo and in vitro three-dimensional (3D) images deep within the samples of interest using low-energy photons, which significantly reduces the photodamage and photobleaching of tissues and organs.1–4 The usability of this technique strongly depends on the performance of the probe molecules; therefore, the development of two-photon (TP) activatable fluorophores remains an urgent issue. In recent years, remarkable progress has been made in the molecular design concepts for dyes to be used in practical applications.1,2,5,6 For instance, TP active dyes that function as detectors of chemical or biological events in the target system and can realize ratiometric imaging are highly important.5,6 The significance of this type of function is apparent from the substantial amount of research on the use of one-photon activatable molecular probes.7,8
The attractive, but still challenging feature of TP active dyes is their efficient TP absorption and bright fluorescence bands in the 650–1100 nm region.5 This wavelength region is the so-called ‘tissue optical window’, where undesired absorption of excitation light and fluorescence, light scattering, and autofluorescence due to biological media are minimal.4 However, the development of such dyes has been limited,6j–l as seen from current reviews.1,2 Furthermore, TP dyes would ideally be excited with the 1050 nm femtosecond fibre laser, which has recently been developed as an inexpensive excitation source.9 One of the advantages of fluorescence techniques is that they are relatively cheaper than other diagnostic tools like single-photon emission computed tomography (SPECT).10 Considering the expensive hardware used, this is currently not the case for TPFM; however, the compatibility of TP dyes with such a fibre laser would make TPFM universally available. The inherent advantages of the TPFM technique would be truly maximized by employing probes that satisfy all the above mentioned requirements.
To this end, we have developed a novel pyrene-based acceptor–π–acceptor (A–π–A) dye based on the following rationale. Our group has previously exploited a molecular design strategy to construct A–π–A structures (i.e. fluorene-based FLW, naphthalene-based NP, and anthracene-based AC, shown in Fig. 1) for TPFM.5c In these structures, two pyridinium moieties, acting as electron acceptors (A), were linked by vinyl linkers to a π-centre consisting of a planer and rigid aromatic chromophore, typified by polycyclic aromatic hydrocarbons (PAHs). The dyes comprising such structures exhibited large TP absorption cross-sections (σ(2)) at longer wavelengths owing to strong intramolecular charge transfer characteristics and extended π-conjugation lengths. However, the PAH-based A–π–A dyes we have developed so far do not satisfy the above-mentioned performance characteristics. Herein, we focused on the pyrene chromophore as a new π-centre for A–π–A dyes. Pyrene often has several photophysical and structural advantages when compared with the other PAHs, e.g. longer excitation and emission wavelengths, higher fluorescence quantum yields (ΦFL), higher absorption extinction coefficients (ε), more π-electrons, and greater planarity and symmetry.11,12 These properties are thought to be related to the spectral red shift of fluorescence and TP absorption and the enhancement of σ(2) values.1
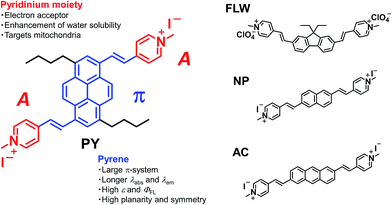 |
| Fig. 1 Molecular design of PAH-based A–π–A dyes and the role of each moiety. | |
In this paper, we report the synthesis of a pyrene-based A–π–A dye PY [(4,4′-((1E,1′E)-(3,8-dibutylpyrene-1,6-diyl)bis(ethene-2,1-diyl))bis(1-methylpyridin-1-ium) iodide)] and its outstanding optical properties in dimethylsulfoxide (DMSO). We also show the actual performance of PY in mitochondrial imaging using the TPFM technique and compare its performance with those of FLW, NP, AC, commercially available dyes, and other existing TP activatable dyes. These results demonstrated that PY is applicable in biological applications, and its use could be extended to other organic diagnostic tools, like fluorescent nanoparticles,13 through further structural modification.
Results and discussion
Synthesis of PY
The synthetic protocol of PY is outlined in Scheme 1. Firstly, compound 1 was prepared through the introduction of two n-butyl groups into the 3 and 8 positions of pyrene. Next, Friedel–Crafts formylation was conducted to obtain a diformylated compound 2. Finally, Knoevenagel condensation between 2 and 1,4-dimethylpyridin-1-ium iodide was carried out to afford the final product PY. The role of the n-butyl groups was to enhance the solubility for the easier preparation of compound 2 and to fix the two formyl groups at the 1 and 6 positions. This pattern of substitution should make PY quadrupolar, which is linked to high σ(2) values.1,12,14 The obtained PY was characterized by 1H and 13C NMR, and high-resolution mass spectrometry (see ESI†). In addition, the solubility of PY in water was estimated to be ∼2 × 10−6 mol L−1 (see Fig. S1 in the ESI†), which satisfied the essential requirement for living cell imaging without using excess organic solvents.5c
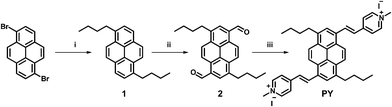 |
| Scheme 1 Synthetic route to PY. (i) (1) THF, n-BuLi, 1-bromobutane, 0 °C, 12 h (2) n-C4H9, r.t., 3 h (78%). (ii) CH2Cl2, TiCl4, Cl2CHOCH3, 0 °C, 23 h (41%). (iii) MeOH/CHCl3, piperidine, 1,4-dimethylpyridin-1-ium iodide, reflux, 12 h (59%). | |
One-photon absorption and fluorescence properties of PY
As mentioned above, PY has sufficient water solubility to stain living cells without the use of organic solvents. However, this water solubility is too low to reliably characterize the TP absorption. Therefore, all spectroscopic measurements were conducted using DMSO as the solvent, unless otherwise noted.
The one-photon absorption and fluorescence spectra of PY were recorded (Fig. 2). Interestingly, PY in DMSO exhibited an absorption peak at 510 nm, which is at a longer wavelength than those observed for FLW, NP, and AC.5c The anthracene-based dye, AC, has an absorption peak at a shorter wavelength (490 nm in DMSO), even though anthracene derivatives generally have longer absorption wavelengths than pyrene derivatives with similar substitution, as does the parent anthracene (λabs = 372 nm compared with λabs = 330 nm for pyrene in ethanol).11b A possible reason for this different behaviour is that the 1 and 6 positions of pyrene may accept stronger π-conjugation from the vinyl group-linked pyridinium moieties than the 2 and 6 positions of anthracene.15 As confirmed by DFT calculations, the substitution of acceptor groups at positions 1 and 6 in pyrene and at positions 9 and 10 in anthracene is most effective to induce red-shifted absorption spectra and to enhance the transition moments (Fig. S2 and S3†). On the other hand, it is expected that substitution at the 9 and 10 positions in anthracene by vinyl group-linked pyridinium moieties would result in a loss of planarity because of steric hindrance, which could decrease the σ(2) value. Thus, pyrene has a structural advantage when compared with anthracene, as the pyrene π-system can be extended significantly without losing its planar structure. Importantly, the energy of a TP absorption allowed state is located at around one-half the energy level of a one-photon absorption allowed state; therefore, such a red-shifted one-photon absorption wavelength for PY is preferable for our purpose.
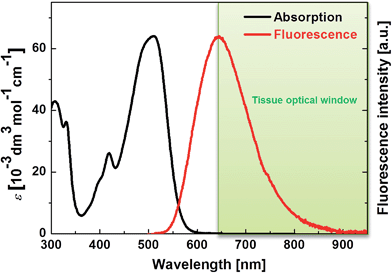 |
| Fig. 2 One-photon absorption and fluorescence spectra of PY in DMSO. The tissue optical window is indicated in green. | |
PY in DMSO showed a fluorescence peak at 650 nm, and the spectrum extended to 850 nm, which means that PY fluorescence is advantageously within the tissue optical window. Furthermore, a ΦFL value of 0.80 was measured; this value is significantly higher than those of the other PAH-based A–π–A dyes (FLW, NP, and AC have ΦFL values in DMSO of 0.44, 0.36, and 0.66, at 542 nm, 516 nm, and 640 nm, respectively).5c The reason for the high ΦFL value of PY might be related to the above-mentioned stronger perturbation from π-conjugation with the pyrene chromophore or its rigid structure, which decreases vibrational deactivation.16 The fluorescence peak of PY in aqueous solution was located at 650 nm. Thus, PY in water also fluoresced within the tissue optical window. The ΦFL value in aqueous solution was lower (0.10); highly polarized dyes exhibiting fluorescence at longer wavelengths tend to be less fluorescent in higher polarity solvents because of charge separation, which increases internal conversion.5c,17 Considering that biological tissues and organs are usually less polar than water, such a strong decrease in the ΦFL value might be preferable to obtain background-free imaging.7d Thus, it was revealed that PY exhibits one-photon absorption that is suitable for realizing a longer wavelength TP absorption band and a fluorescence band with a high ΦFL value in the tissue optical window.
Two-photon absorption properties of PY
The TP absorption spectrum of PY in DMSO was recorded using the open-aperture Z-scan technique.1 The maximum σ(2) value of PY was 1100 GM (1 GM = 10−50 cm4 per photon per molecule) at 950 nm (Fig. 3); this value is significantly higher than those for FLW, NP, and AC. PY also showed significant TP absorption at 1050 nm (380 GM), indicating that PY is compatible with the 1050 nm fibre laser source, whereas the TP absorption of FLW, NP, and AC at wavelengths longer than 1000 nm is quite small.5c These characteristics of PY, including the large σ(2) value and longer TP absorption wavelength, might be explained by comparison with the other PAH-based A–π–A dyes (e.g.FLW, NP, and AC, which show 740 GM at 780 nm, 712 GM at 695 nm, and 687 GM at 703 nm, respectively5c) as follows:
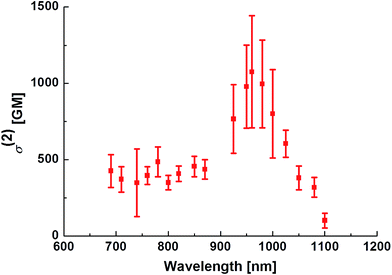 |
| Fig. 3 Two-photon absorption spectrum of PY in DMSO. | |
1. Unlike FLW, PY has a quadrupolar structure, as do NP and AC.
2. PY has a larger π-electron system than the other PAH derivatives, especially FLW and NP.
3. PY is more strongly conjugated with the vinyl group-linked pyridinium moieties than AC, as mentioned in the section concerning one-photon absorption.
The performance of a TP active fluorescent molecule is generally estimated using the value of the TP action cross-section (Φσ(2)), which is the product of the values of ΦFL and σ(2). The Φσ(2) value of PY at 950 nm was 880 GM; this value is quite large compared with the values for other TP active dyes, as described later (see Fig. 5).
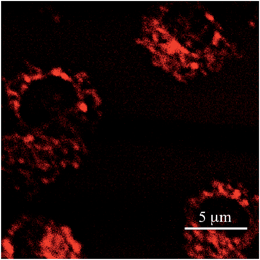 |
| Fig. 4 Two-photon fluorescence microscope images of Hek293 cells stained with PY. This image was obtained with 950 nm excitation, and the fluorescence was collected through a 600–700 nm band-pass filter. | |
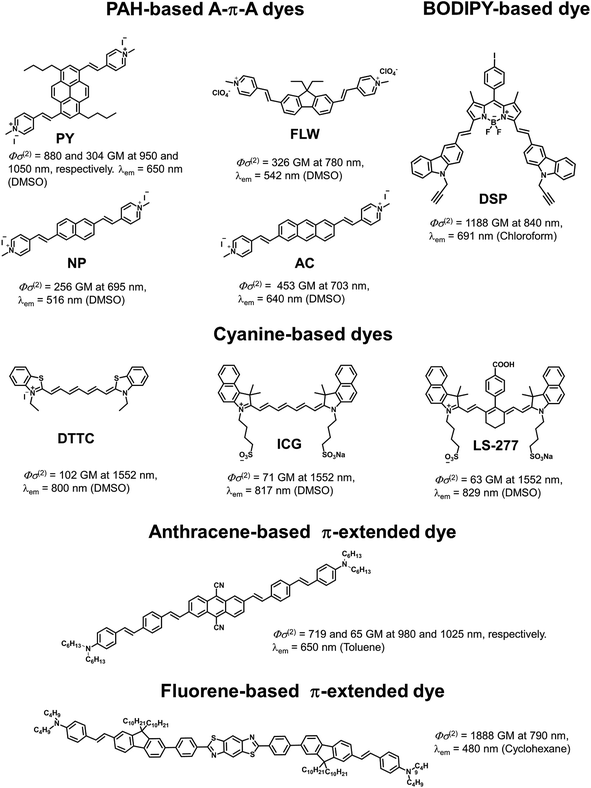 |
| Fig. 5 Examples of TP active fluorophores: PAH-based A–π–A dyes,5c a BODIPY-based dye,5a cyanine-based dyes,5e an anthracene-based π-extended dye,1,21 and fluorene-based π-extended dye.2,22 These dyes were selected because they show attractive spectroscopic properties (TP absorption and fluorescence wavelengths, and Φσ(2)). | |
Imaging of living mitochondria with TPFM
To evaluate the actual performance of PY as a TP active fluorescent probe, the imaging of living mitochondria in Hek293 cells was carried out by TPFM. It is well-known that fluorophores possessing a pyridinium moiety can function as mitochondrial probes.5c,d,8 A co-staining experiment with another mitochondrial probe (FLW)5d revealed that PY could also selectively accumulate on the mitochondria (Fig. S4†). As shown in Fig. 4, TPFM images of the mitochondria stained by PY were obtained with 950 nm excitation at a power of less than 3 mW. Considering that rhodamine 123,18 a commercially available mitochondrial probe, requires a power of 50 mW in the same instruments, PY achieved extremely sensitive imaging, as expected from its high Φσ(2) value. Importantly, the excitation power of a dye is proportional to the Φσ(2) value. Therefore, if a TPFM that is equipped with an excitation light source operating at 1050 nm is available, TPFM imaging could be measured by employing 8.7 mW of a 1050 nm light source. This power is sufficiently lower than the maximum output power of recent 1050 nm fibre lasers.9 As the optical components of current TPFMs are not optimized for excitation at 1050 nm, it is not easy to obtain TPFM images with this excitation wavelength. However, after necessary optimization is realized, PY will be usable as a probe for TPFM employing the 1050 nm fibre laser.
Comparison of PY with other dyes for TPFM
Finally, we compared PY with existing TP active dyes to demonstrate the superiority of our fluorophore (Fig. 5). As is apparent from the above discussion, PY has many desirable properties for TPFM, such as a high Φσ(2) value and longer TP absorption and fluorescence wavelengths, compared with other PAH-based A–π–A dyes and commercially available mitochondrial dyes, such as green fluorescent protein (GFP; Φσ(2) = 6 GM at 800 nm and λem = 509 nm)19 and Rhodamine 123 (Φσ(2) = 72 GM at 800 nm and λem = 534 nm).18 There are a number of cyanine-based near infrared region (NIR) fluorescent dyes for TPFM that have recently been reported by Berezin and co-workers, such as ICG (Φσ(2) = 71 GM at 1500 nm and λem = 817 nm) and LS-277 (Φσ(2) = 63 GM at 1500 nm and λem = 829 nm).5e These dyes are highly attractive owing to their fluorescence within the tissue optical window and TP absorption activated by an ultrafast turnkey fibre laser source. However, these dyes are excited using 1500 nm light, which means their TP absorption bands are outside the tissue optical window. Furthermore, such dyes have relatively lower Φσ(2) values at 1500 nm. Belfield et al. recently presented a large number of TP active fluorescent probes for bioimaging.2 Although there are a few examples of dyes with Φσ(2) values at their maximum TP absorption wavelength higher than that of PY, to the best of our knowledge, there are no dyes showing both TP absorption bands around 1000 nm and fluorescence bands within the optical tissue window that are more efficient than PY. In fact, most of the existing TP-active dyes can be excited in the short-wavelength NIR region around 700–800 nm rather than the so-called “second near-infrared window (NIR II, in the range 1000–1350 nm),” where it was recently proposed that the penetration of excitation light is ideal.20 However, this means that these dyes are not compatible with a 1050 nm fibre laser even if they possess higher Φσ(2) values than PY. As shown in Fig. 5, highly π-extended fluorene or anthracene derivatives, as well as BODIPY derivatives, exhibit relatively similar properties to PY with respect to Φσ(2) values and fluorescence wavelengths, however, these dyes might not be suitable for bio-imaging due to their low solubility in water. In addition, the syntheses of these dyes are not trivial compared to that of PY. Thus, PY can be regarded as an exceptionally superior TP active dye with a comparatively simple and small structure. We emphasise that the outstanding properties of PY are strongly related to both the structural and electronic advantages of the pyrene chromophore, i.e. high planarity and symmetry, rigidness, synthetic accessibility for efficient π-extension and derivatization, and an underlying large π-system. Therefore, we believe that the strategic use of pyrene as a chromophore in TP active dyes will be of great importance and that PY itself can be further derivatized for other practical applications.
Conclusions
We have developed a novel pyrene-based A–π–A dye, PY, which showed a TP absorption band around 1000 nm and fluorescence in the tissue optical window with an outstanding Φσ(2) value. Almost all the properties of PY are derived from the inherent structural and electronic advantages of the pyrene chromophore, including planarity, symmetry, rigidness, a large π-system, and synthetic accessibility for the efficient extension of π-conjugation. We have also demonstrated the ability of PY to provide 3D images of the mitochondria in living Hek293 cells with higher sensitivity than the other PAH-based dyes and commercially available mitochondrial probes, as was expected from the performance of PY in spectroscopic measurements in solution. Finally, the comparison of PY with other known TP active fluorescent probes revealed that PY is an exceptional dye in terms of the location of its TP absorption around the NIR II window and its high Φσ(2) value, indicating that PY is a promising candidate as a diagnostic tool and its ability to be used with the 1050 nm fibre laser will help the TPFM technique become more universal. To date, pyrene derivatives have been developed for many applications; therefore, there are a number of well-established synthetic methodologies where pyrene is used as a building block.23 Thus, PY can be modified for many purposes, e.g. the further improvement of the compatibility of PY to the 1050 nm laser source by introducing other functional groups into the 3 and 8 positions on the pyrene chromophore, use of ligands for active targeting,24 addition of environment sensitivity for the detection of chemical or biological events such as the generation of reactive oxygen species in mitochondria,8 and further derivatization for building fluorescent nanoparticles.13 Based on these facts and our findings, we believe that PY is a useful building block as a TP absorber and fluorophore for TPFM probes. In fact, we are currently focusing on the use of PY for not only in vitro cell imaging but also in vivo deep tissue imaging, using the 1050 nm fibre laser.
Acknowledgements
We thank the JSPS Fellowship for Young Scientists (to Y. N.). This work was supported by a Grant-in-Aid for Scientific Research (no. 23350068) from the Ministry of Education, Culture, Sports, Science and Technology, Japan and Yamaguchi Prefecture Contracted R&D for the Industrial Cluster of Next Generation from Yamaguchi Prefectural Industrial Technology Institute, Japan.
Notes and references
- M. Pawlicki, H. A. Collins, R. G. Denning and H. L. Anderson, Angew. Chem., Int. Ed., 2009, 48, 3244–3266 CrossRef CAS PubMed.
- S. Yao and K. D. Belfield, Eur. J. Org. Chem., 2012, 17, 3199–3217 CrossRef.
- W. Denk, J. H. Strickler and W. W. Webb, Science, 1990, 248, 73–76 CAS.
-
(a) R. Weissleder, Nat. Biotechnol., 2001, 19, 316–317 CrossRef CAS PubMed;
(b) Y. Pu, L. Shi, S. Pratavieira and R. R. Alfano, J. Appl. Phys., 2013, 114, 153102 CrossRef PubMed.
-
(a) X. Zhang, Y. Xiao, J. Qi, J. Qu, B. Kim, X. Yue and K. D. Belfield, J. Org. Chem., 2013, 78, 9153–9160 CrossRef CAS PubMed;
(b) T. Zhang, X. Zhu, C. C. W. Cheng, W.-M. Kwok, H.-L. Tam, J. Hao, D. W. J. Kwong, W.-K. Wong and K.-L. Wong, J. Am. Chem. Soc., 2011, 133, 20120–20122 CrossRef CAS PubMed;
(c) M. Tominaga, S. Mochida, H. Sugihara, K. Satomi, H. Moritomo, A. Fuji, A. Tomoyuki, Y. Suzuki and J. Kawamata, Chem. Lett., 2014 Search PubMed , in press;
(d) S. Tani, K. Nakagawa, T. Honda, H. Saito, Y. Suzuki, J. Kawamata, M. Uchida, A. Sasaki and M. Kinjo, Curr. Pharm. Biotechnol., 2012, 13, 2649–2654 CAS;
(e) M. Y. Berezin, C. Zhan, H. Lee, C. Joo, W. J. Akers, S. Yazdanfar and S. Achilefu, J. Phys. Chem. B, 2011, 115, 11530–11535 CrossRef CAS PubMed.
-
(a) Y.-C. Zheng, M.-L. Zheng, S. Chen, Z.-S. Zhao and X.-M. Duan, J. Mater. Chem. B, 2014, 2, 2301–2310 RSC;
(b) H. Zhang, J. Fan, H. Dong, S. Zhang, W. Xu, J. Wang, P. Gao and X. Peng, J. Mater. Chem. B, 2013, 1, 5450–5455 RSC;
(c) N. Xie, K. Feng, B. Chen, M. Zhao, S. Peng, L.-P. Zhang, C.-H. Tung and L.-Z. Wu, J. Mater. Chem. B, 2014, 2, 502–510 RSC;
(d) X. Wang, J. Sun, W. Zhang, X. Ma, J. Lv and B. Tang, Chem. Sci., 2013, 4, 2551–2556 RSC;
(e) K. P. Divya, S. Sreejith, P. Ashokkumar, K. Yuzhan, Q. Peng, S. K. Maji, Y. Tong, H. Yu, Y. Zhao, P. Ramamurthy and A. Ajayaghosh, Chem. Sci., 2014 10.1039/c4sc00736k;
(f) G. Masanta, C. H. Heo, C. S. Lim, S. K. Bae, B. R. Cho and H. M. Kim, Chem. Commun., 2012, 48, 3518–3520 RSC;
(g) E. De Meulenaere, W.-Q. Chen, S. Van Cleuvenbergen, M.-L. Zheng, S. Psilodimitrakopoulos, R. Paesen, J.-M. Taymans, M. Ameloot, J. Vanderleyden, P. Loza-Alvarez, X.-M. Duan and K. Clays, Chem. Sci., 2012, 3, 984–995 RSC;
(h) J. Jing, J.-J. Chen, Y. Hai, J. Zhan, P. Xu and J.-L. Zhang, Chem. Sci., 2012, 3, 3315–3320 RSC;
(i) J. Massin, A. Charaf-Eddin, F. Appaix, Y. Bretonnière, D. Jacquemin, B. van der Sanden, C. Monnereau and C. Andraud, Chem. Sci., 2013, 4, 2833–2843 RSC;
(j) P. Yan, A. Xie, M. Wei and L. M. Loew, J. Org. Chem., 2008, 73, 6587–6594 CrossRef CAS PubMed;
(k) P. Yan, C. D. Acker, W.-L. Zhou, P. Lee, C. Bollensdorff, A. Negrean, J. Lotti, L. Sacconi, S. D. Antic, P. Kohl, H. D. Mansvelder, F. S. Pavone and L. M. Loew, Proc. Natl. Acad. Sci. U. S. A., 2012, 109, 20443–20448 CrossRef CAS PubMed;
(l) J. Massin, A. Charaf-Eddin, F. Appaix, Y. Bretonnière, D. Jacquemin, B. van der Sanden, C. Monnereau and C. Andraud, Chem. Sci., 2013, 4, 2833–2843 RSC.
-
(a) Z. Yang, J. Cao, Y. He, J. H. Yang, T. Kim, X. Peng and J. S. Kim, Chem. Soc. Rev., 2014, 43, 4563–4601 RSC;
(b) A. P. de Silva, H. Q. N. Gunaratne, T. Gunnlaugsson, A. J. M. Huxley, C. P. McCoy, J. T. Rademacher and T. E. Rice, Chem. Rev., 1997, 97, 1515–1566 CrossRef CAS PubMed;
(c) R. W. Sinkeldam, N. J. Greco and Y. Tor, Chem. Rev., 2010, 110, 2579–2619 CrossRef CAS PubMed;
(d) A. S. Klymchenko and R. Kreder, Chem. Biol., 2014, 21, 97–113 CrossRef CAS PubMed.
-
(a) J.-T. Hou, M.-Y. Wu, K. Li, J. Yang, K.-K. Yu, Y.-M. Xie and X.-Q. Yu, Chem. Commun., 2014, 50, 8640–8643 RSC;
(b) P. Li, H. Xiao, Y. Cheng, W. Zhang, F. Huang, W. Zhang, H. Wang and B. Tang, Chem. Commun., 2014, 50, 7184–7187 RSC;
(c) S. Hirosawa, S. Arai and S. Takeoka, Chem. Commun., 2012, 48, 4845–4847 RSC;
(d) N. Jiang, J. Fan, T. Liu, J. Cao, B. Qiao, J. Wang, P. Gao and X. Peng, Chem. Commun., 2013, 49, 10620–10622 RSC.
- Examples of 1050 nm femtosecond fibre laser; Ytterbius-1100 (Tekhnoscan), FCPA MicroJewel (IMRA), Origami (Onefive) or Fidelity (Coherent), and so on.
-
(a) S. Keereweer, J. D. Kerrebijn, P. B. van Driel, B. Xie, E. L. Kaijzel, T. J. Snoeks, I. Que, M. Hutteman, J. R. van der Vorst, J. S. Mieog, A. L. Vahrmeijer, C. J. van de Velde, R. J. Baatenburg de Jong and C. W. Lowik, Mol. Imag. Biol., 2011, 13, 199–207 CrossRef PubMed;
(b) K. Licha and C. Olbrich, Adv. Drug Delivery Rev., 2005, 57, 1087–1108 CrossRef CAS PubMed;
(c) J. Rao, A. Dragulescu-Andrasi and H. Yao, Curr. Opin. Biotechnol., 2007, 18, 17–25 CrossRef CAS PubMed.
-
(a) S. Dufresne, I. U. Roche, T. Skalski and W. G. Skene, J. Phys. Chem. C, 2010, 114, 13106–13112 CrossRef CAS;
(b) Y. Niko, Y. Hiroshige, S. Kawauchi and G. Konishi, J. Org. Chem., 2012, 77, 3986–3996 CrossRef CAS PubMed.
- H. M. Kim, Y. O. Lee, C. S. Lim, J. S. Kim and B. R. Cho, J. Org. Chem., 2008, 73, 5127–5130 CrossRef CAS PubMed.
-
(a) K. Li, W. Qin, D. Ding, N. Tomczak, J. Geng, R. Liu, J. Liu, X. Zhang, H. Liu, B. Liu and B. Z. Tang, Sci. Rep., 2013, 3, 1150, DOI:10.1038/srep0115;
(b) A. S. Klymchenko, E. Roger, N. Anton, H. Anton, I. Shulov, J. Vermot, Y. Mély and T. F. Vandamme, RSC Adv., 2012, 2, 11876–11886 RSC;
(c) J. H. Kim, S. Lee, K. Park, H. Y. Nam, S. Y. Jang, I. Youn, K. Kim, H. Jeon, R. W. Park, I. S. Kim, K. Choi and I. C. Kwon, Angew. Chem., Int. Ed., 2007, 46, 5779–5782 CrossRef CAS PubMed;
(d) A. Reisch, P. Didier, L. Richert, S. Oncul, Y. Arntz, Y. Mély and A. S. Klymchenko, Nat. Commun., 2014, 5 DOI:10.1038/ncomms5089.
- M. Albota, D. Beljonne, J.-L. Brédas, J. E. Ehrlich, J.-Y. Fu, A. A. Heikal, S. E. Hess, T. Kogej, M. D. Levin, S. R. Marder, D. McCord-Maughon, J. W. Perry, H. Röckel, M. Rumi, G. Subramaniam, W. W. Webb, X.-L. Wu and C. Xu, Science, 1998, 281, 1653–1656 CrossRef CAS.
-
(a) S. Méry, D. Haristoy, J.-F. Nicoud, D. Guillon, H. Monobe and Y. Shimizu, J. Mater. Chem., 2003, 13, 1622–1630 RSC;
(b) M. Uchimura, Y. Watanabe, F. Araoka, J. Watanabe, H. Takezoe and G. Konishi, Adv. Mater., 2010, 22, 4473–4478 CrossRef CAS PubMed;
(c) Y. Niko and G. Konishi, J. Synth. Org. Chem., Jpn., 2012, 70, 918–927 CrossRef CAS.
-
N. J. Turro, V. Ramamurthy and J. C. Scaiano, Modern Molecular Photochemistry of Organic Molecules, University Science Books, Sausalito, California, 2010, pp. 289–290 Search PubMed.
-
(a)
N. J. Turro, V. Ramamurthy and J. C. Scaiano, Modern Molecular Photochemistry of Organic Molecules, University Science Books, Sausalito, California, 2010, pp. 261–303 Search PubMed;
(b) T. Soujanya, R. W. Fessenden and A. Samanta, J. Phys. Chem., 1996, 100, 3507–3512 CrossRef CAS;
(c) G. F. Mes, B. De Jong, H. J. Van Ramesdonk, J. W. Verhoeven, J. M. Warman, M. P. De Haas and L. E. W. Horsman-van den Dool, J. Am. Chem. Soc., 1984, 106, 6524–6528 CrossRef CAS.
- R. Wolleschensky, T. Feurer, R. Sauerbrey and U. Simon, Appl. Phys. B, 1998, 67, 87–94 CrossRef CAS.
- P. T. C. So, C. Y. Dong, B. R. Masters and K. M. Berland, Annu. Rev. Biomed. Eng., 2000, 2, 399–429 CrossRef CAS PubMed.
-
(a) R. Subha, V. Nalla, J. H. Yu, S. W. Jun, K. Shin, T. Hyeon, C. Vijayan and W. Ji, J. Phys. Chem. C, 2013, 117, 20905–20911 CrossRef CAS;
(b) J. T. Robinson, G. Hong, Y. Liang, B. Zhang, O. K. Yaghi and H. Dai, J. Am. Chem. Soc., 2012, 134, 10664–10669 CrossRef CAS PubMed;
(c) G. Hong, J. C. Lee, J. T. Robinson, U. Raaz, L. Xie, N. F. Huang, J. P. Cooke and H. Dai, Nat. Med., 2012, 18, 1841–1846 CrossRef CAS PubMed.
- S. K. Lee, W. J. Yang, J. J. Choi, C. H. Kim, S.-J. Jeon and B. R. Cho, Org. Lett., 2005, 7, 323–326 CrossRef CAS PubMed.
- S. Yao, H.-Y. Ahn, X. Wang, J. Fu, E. W. van Stryland, D. J. Hagan and K. D. Belfield, J. Org. Chem., 2010, 75, 3965–3974 CrossRef CAS PubMed.
-
(a) T. M. Figueira-Duarte and K. Müllen, Chem. Rev., 2011, 111, 7260–7314 CrossRef CAS PubMed;
(b) Y. Niko, S. Kawauchi, S. Otsu, K. Tokumaru and G. Konishi, J. Org. Chem., 2013, 78, 3196–3207 CrossRef CAS PubMed;
(c) Y. Niko, S. Kawauchi and G. Konishi, Chem.–Eur. J., 2013, 19, 9760–9765 CrossRef CAS PubMed;
(d) Y. Niko, S. Sasaki, S. Kawauchi, K. Tokumaru and G. Konishi, Chem.–Asian J., 2014, 9, 1797–1807 CrossRef CAS PubMed;
(e) Y. Niko, Y. Cho, S. Kawauchi and G. Konishi, RSC Adv., 2014, 4, 36480–36484 RSC.
- S. Luo, E. Zhang, Y. Su, T. Cheng and C. Shi, Biomaterials, 2011, 32, 7127–7138 CrossRef CAS PubMed.
Footnote |
† Electronic supplementary information (ESI) available. See DOI: 10.1039/c4tb01404a |
|
This journal is © The Royal Society of Chemistry 2015 |
Click here to see how this site uses Cookies. View our privacy policy here.