Tailoring hierarchical meso–macroporous 3D scaffolds: from nano to macro
Received
20th September 2013
, Accepted 21st October 2013
First published on 22nd October 2013
Abstract
Bone tissue regeneration requires the use of 3D scaffolds which mimic the architecture of the natural extracellular matrix, creating an adequate microenvironment for bone cell growth. Such 3D scaffolds need surface properties suitable for biological recognition in the early stage of cell adhesion, necessary to ensure complete cell colonization, retained cell functionality, and subsequently bone regeneration. Herein, hierarchical 3D scaffolds based on new hydroxyapatite/mesoporous glass nanocomposite bioceramic (MGHA) exhibiting different scales of porosity have been synthesized. These 3D scaffolds possess: (i) highly ordered mesopores with diameters of 10 nm; (ii) macropores with diameters in the 30–80 μm range with interconnections of 1–10 μm; and (iii) large macropores of ca. 500 μm. To improve their surface properties, 3D scaffolds were modified through direct functionalization with amine propyl groups, which notably improve preosteoblast adhesion, proliferation (2.3 fold), differentiation (4.8 fold) and further cell colonization of these scaffolds. The observed enhancement can be related to these amine groups which favour early adhesion, e.g., based on nonspecific protein adsorption as was demonstrated by ellipsometry. These results suggest that the combination of hierarchical structure design and amine surface modification of hydroxyapatite/mesoporous nanocomposite scaffolds yields a double increase in cell proliferation, as well as a quadruple increase in cell differentiation, demonstrating the potential of these nanocomposite materials for bone tissue regeneration purposes.
1. Introduction
Bone tissue engineering requires the use of tridimensional (3D) scaffolds which act as temporary templates for migration, proliferation, and differentiation of cells to guide bone repair, stimulating natural mechanisms of bone regeneration.1–3 These processes are affected by both pore structure at different length scales and the chemical surface composition of these implants, which constitute fundamental pillars in the design and manufacture of these scaffolds.1 The macroporous architecture of the scaffold should maintain the mechanical integrity of the material during bio-resorption, and also sustain both cell proliferation and new extracellular matrix deposition. In vivo studies have shown that interconnected macropores in the range of 150–500 μm and porosity over 50% led directly to bone mineralization.4–6 Moreover, pore interconnection is directly related to vascularisation and nutrient diffusion, both critical for osteogenesis.5 Furthermore, it has been established that the nanoscale in the scaffold surface has important implication in the control of cell behaviour. In fact, natural bone has nanoscale dimensions, where the extracellular matrix (ECM) is mainly constituted by the collagen-fiber framework (10–300 nm) with interconnected nanopores, and hydroxyapatite nanocrystals (25–4 nm).7,8 Thus, several studies have shown that nanostructured porous surfaces significantly enhance cell attachment, cell viability, alkaline phosphatase (ALP) activity, and gene expression of osteoblasts on hydroxyapatite scaffolds.9
However, most synthetic 3D scaffolds lack chemical surface properties involved in the biological recognition processes and in the early stage of cell adhesion, necessary to ensure the complete cell colonization for subsequent bone regeneration.6,10 Cell adhesion is the first event in the recognition between the 3D scaffold surface and cells, playing a primary role in posterior cellular responses.11,12 This process depends on both non-specific and specific cell/biomaterial surface interactions with cooperative effects.11 Non-specific cell adhesion through physical/chemical interactions is responsible for the early stage cell adhesion, which involves the contact of the biomaterial surface with the pericellular coat (PCC) containing hyaluronan, highly negatively charged under the physiological conditions.13,14 Subsequently, specific adhesion mechanisms are triggered by conjugation of extracellular ligands to receptors in the cell membrane.12 These receptors are heterodimeric transmembrane proteins called integrins, which, in a natural environment, interact with adhesion peptide sequences of extracellular matrix proteins.15
Numerous studies have focused on the anchoring of specific adhesion peptides, e.g., RGD, a peptide to enhance both processes.1,16–18 These approaches are, however, expensive and require high control of peptide location and final conformation on the scaffold surface. In fact, cell adhesion on these modified supports is often not significantly better than on commercialized tissue-culture plastic (TCP), based on a simple functionalization with lysine moieties on the plate surface.19 In this sense, TCP exhibits good cell adhesion due to its ability to enable absorption of proteins in culture medium and then enhances non-specific adhesion of cells. Based on this strategy, there is considerable interest in using simple chemistry to improve cell responses, addressing the incorporation of positively charged amino moieties in the biomaterial surface which significantly favour cell adhesion and further differentiation.20,21
Recently, a nanocomposite bioceramic (MGHA), formed by particles of nanocrystalline apatite embedded into amorphous mesoporous bioactive glass in the SiO2–P2O5–CaO system, has been reported.22 Due to the synergy of the features of its two components, including (i) ordered mesoporous arrangement with pores of 8 nm, (ii) high surface area and pore volume, (iii) high bioactivity, (iv) presence of nanocrystalline apatite particles homogeneously distributed, and (v) improved in vitro biocompatibility, this nanocomposite material is an excellent candidate for bone tissue engineering. However, with this objective, it is necessary to obtain 3D macroporous scaffolds based on this nanostructured material with the porosity ranges required for proper cell colonization, preserving the intrinsic characteristics of both components during the scaffold manufacturing process. The aim of the present study was therefore to prepare hierarchical meso–macroporous 3D MGHA scaffolds in a one-pot process by the combination of different meso- and macro-structural directing agents through the rapid prototyping (RP) technique. Moreover, to improve the cell adhesion and the scaffold colonization, a simple surface functionalization with aminopropyl groups directly on the scaffolds was also carried out. MC3T3 preosteoblast proliferation and differentiation on these 3D MGHA scaffolds were analysed to confirm the performance of the scaffold design, while ellipsometry was employed for investigating the potential role of protein adsorption in cell adhesion.
2. Materials and methods
2.1. 3D MGHA scaffold preparation
3D scaffolds based on a mesoporous glass/hydroxyapatite nanocomposite (MGHA) were prepared by rapid prototyping (RP) via direct-write assembly of precursor slurry using an EnvisionTEC GmbH Prefactory® 3D Bioplotter™ (Gladbeck, Germany). This RP technique constitutes an excellent alternative for the manufacture of scaffolds suitable for different clinical applications and individuals.23 The slurry is derived from a sol precursor of MGHA nanocomposite previously synthesized in powder,22 to which was added methylcellulose (MC) to produce a slurry with a suitable consistency to be extruded during the RP process.24,25 In a typical synthesis, 19.5 g of F127 was dissolved in 168.6 mL of absolute ethanol (99.5%, Panreac) with 12.8 mL of 1.0 M HCl (prepared from 37% HCl, Panreac) solution and 19.4 mL of Milli-Q water. Afterwards, the appropriate amounts of tetraethyl orthosilicate (TEOS, 98%, Sigma-Aldrich), triethyl phosphate (TEP, 99.8%, Sigma-Aldrich), and calcium chloride (CaCl2·4H2O, 99%, Sigma-Aldrich) as SiO2, P2O5, and CaO sources, respectively, were added in 1 h intervals under continuous stirring for 4 h at 40 °C and subsequently maintained under static conditions at the same temperature overnight. Subsequently, the MGHA sol precursor was aged for 4–5 days until reaching a final volume reduction of ≈30% through evaporation-induced self-assembly (EISA). Then, MC was added under vigorous stirring in a F127/MC molar ratio of 6 obtaining a suitable consistency to be extruded in the RP process. Cylindrical 50 × 133 × 38 mm scaffolds were designed and fabricated layer-by-layer by direct ink deposition over a plate at room temperature. Each layer was pre-designed showing a 90° rotation with respect to the previous. To obtain the best results, the dispensing speed and pressure were slightly modified from the initial machine parameters during the dispensing process for each scaffold. The scaffold hardening process occurred by solvent evaporation and the pieces were left to dry at 30 °C. Finally, the pieces were calcined at 700 °C for 6 h.
2.2. Amine functionalization of 3D MGHA scaffolds
Nanocomposite 3D MGHA scaffolds were functionalized directly post-synthesis with amine groups using 3-aminopropyltriethoxysilane (C9H23NO3Si, APTES, ≥98% wt, Sigma-Aldrich). In doing so, two scaffold pieces (25 mg of weight per piece) were degassed overnight at 100 °C, followed by the addition of 12.5 mL of dried toluene (98%, Aldrich). Subsequently, 0.5 mL of APTES reactant was incorporated and kept for 12 h in reflux under a nitrogen atmosphere at 80 °C. Then, the scaffolds were removed and washed in aqueous medium under continuous stirring for 24 h. The resulting amine functionalized scaffolds are denoted as MGHA-NH2.
2.3. Characterization
X-ray diffraction (XRD) experiments were performed on a Philips X'Pert diffractometer (Eindhoven, The Netherlands), equipped with Cu Kα (40 kV, 20 mA). Transmission electron microscopy (TEM) was performed on a JEOL 3010 electron microscope (Jeol Ltd., Japan), operating at 300 kV (Cs; 0.6 mm, resolution 1.7 Å). All TEM images were recorded employing a CCD camera (MultiScan model 794, Gatan Inc., UK) under low-dose conditions. Fourier transform (FT) patterns were extracted from the images of thin crystal regions using a Digital Micrograph (Gatan Inc., UK). Scanning electron microscopy (SEM) was performed using a field emission JEOL JSM-6335F microscope (Tokyo, Japan) at an acceleration voltage of 10 kV. Textural properties were determined by N2 adsorption porosimetry using a Micromeritics ASAP2020 analyzer (Norcross, USA). Prior to these measurements, both MGHA and MGHA-NH2 scaffolds were degassed at 60 °C for 24 hours under vacuum (<0.3 kPa). The surface area was determined using the multipoint Brunauer–Emmett–Teller method included in the software. The porosity of 3D scaffolds was measured by mercury intrusion porosimetry using a Micromeritics Autopore IV 9500 device (Micromeritics Instrument Corporation, Norcross, GA, USA). Elemental analyses (C, H, N) were carried out on a LECO CHNS-932 microanalyzer (Saint Joseph, Michigan USA). Fourier transform infrared (FTIR) spectroscopy was performed in a Thermo Nicolet Nexus spectrometer (Thermo Scientific, USA) from 4000 to 400 cm−1, using the KBr pellet method and operating in transmittance mode. 29Si, 31P and 13C single pulse (SP) and cross-polarization (CP)/MAS (magic angle spinning) solid-state nuclear magnetic resonance (NMR) measurements were performed to evaluate the different silicon, phosphorus, and carbon environments in synthesized scaffolds. NMR spectra were recorded on a Bruker Avance 400 WB spectrometer (Karlsruhe, Germany). Samples were spun at 10, 6, and 12 kHz for 29Si, 31P and 13C, respectively. These spinning speeds were sufficient to make the spinning sidebands of low intensity and outside the region of interest. Spectrometer frequencies were set to 79.49, 161.97, and 75.45 MHz for 29Si, 31P, and 13C, respectively. Chemical shift values were referenced to 3-trimethylsilyl-1-propanesulfonic acid sodium salt (DDS), 85% phosphoric acid (H3PO4) and glycine for 29Si, 31P, and 13C, respectively. Time periods between successive accumulations were 5, 4, and 3 ms for 29Si, 31P, and 13C, respectively, and the number of scans was ca. 13
000 for all spectra. Zeta-potential (ζ) measurements were performed on a Malvern Zetasizer Nano Series instrument (Malvern Instruments Ltd., UK). For this purpose, 10 mg of each powdered sample was added to 20 mL of KCl solution (20 mM) and the mixture was vigorously stirred to reach a homogenous suspension. The measurements were carried out at in the pH range of 2–10 by the addition of HCl solution (0.01 M) and NaOH (0.01 M). Six ζ-potential runs were recorded for each sample at 37 °C, with a minimum of 30 sub runs per measurement, whereafter average values and standard deviations were calculated (n = 6).
2.4. Protein adsorption
Hydrophilic and negatively charged silica surfaces were prepared from polished silicon slides (p-type, boron doped). The surfaces were cleaned in a mixture of 25% NH4OH, 30% H2O2, and H2O (1 : 1: 5, by volume) at 80 °C for 5 min, followed by cleaning in a mixture of 32% HCl, 30% H2O2, and H2O (1 : 1 : 5, by volume) at 80 °C for 5 min. The slides were then rinsed twice with water and ethanol. This procedure rendered the surfaces hydrophilic, with a water–air contact angle of less than 10°. MGHA was deposited on these silica slides through a dip-coating method using the MGHA precursor sol aged for 24 hours at room temperature, with a withdrawal rate of 2500 mm s−1. The coatings were dried at 100 °C (1 h) and annealed in air at 700 °C for 1 hour to remove the surfactant and to produce the MGHA nanocomposite phase. Finally, the coatings were washed with ethanol in an ultrasonic bath for 2 min. Homogeneous MGHA coatings obtained were confirmed by SEM and EDS studies. Subsequently, these coatings were amine functionalized by the same procedure, this time using APTES moieties as the functionalizing agent. The effectiveness of the amine functionalization process was confirmed by FTIR and XRD analyses. Fibrinogen adsorption to such MGHA deposited on silica surfaces was studied in situ by null ellipsometry, using an Optrel Multiskop (Optrel, Kleinmachnow, Germany) equipped with a 100 mW argon laser. All measurements were carried out at 532 nm and an angle of incidence of 67.66° in a 5 mL cuvette under stirring (300 rpm). Both the principles of null ellipsometry and the procedures used have been described extensively before.26 In brief, by monitoring the changes in the state of polarization of light reflected at a surface in the absence and presence of an adsorbed layer, the mean refractive index (n) and layer thickness (d) of the adsorbed layer can be obtained. From the thickness and refractive index the adsorbed amount (Γ) was calculated according to: |  | (1) |
where n0 is the refractive index of the bulk solution, and dn/dc the refractive index increment (0.154 cm3 g−1). Corrections were routinely done for changes in the bulk refractive index caused by changes in temperature and excess electrolyte concentration.
2.5. Cell adhesion, morphology, mitochondrial activity and cell differentiation
2.5.1. Cell culture.
MGHA and MGHA-NH2 scaffolds were sterilized under UV light for 15 min and then submerged in Dulbecco's Modified Eagle's Medium (DMEM, Sigma Chemical Company, St. Louis, MO, USA) supplemented with penicillin (800 μg mL−1, BioWhittaker Europe, Belgium), streptomycin (800 μg mL−1, BioWhittaker Europe, Belgium), β-glycerolphosphate (50 μg mL−1, Sigma Chemical Company, St. Louis, MO, USA) and L-ascorbic acid (10 mM, Sigma Chemical Company, St. Louis, MO, USA), under a CO2 (5%) atmosphere at 37 °C for 24 h to stabilize before cell culture. Subsequently, murine MC3T3-E1 preosteoblasts (as undifferentiated osteoblast-like cells) were seeded at a density of 105 cells per mL in DMEM with 10% fetal bovine serum (FBS, Gibco, BRL), 1 mM L-glutamine (BioWhittaker Europe, Belgium), penicillin (200 μg mL−1, BioWhittaker Europe, Belgium), and streptomycin (200 μg mL−1, BioWhittaker Europe, Belgium), under a CO2 (5%) atmosphere at 37 °C for 48 h.
2.5.2. Morphological studies by scanning electron microscopy.
For SEM studies, cells attached to the 3D scaffolds were fixed with glutaraldehyde (2.5% in Phosphate Buffer Saline, PBS) for 45 min. Sample dehydration was performed by slow water replacement, using a series of ethanol solutions (30%, 50%, 70%, 90%) for 30 min with a final dehydration in absolute ethanol for 60 min, allowing samples to dry at room temperature and under vacuum. Afterwards, the pieces were mounted on stubs and coated in a vacuum with gold–palladium. Cells were examined with a JEOL JSM-6400 scanning electron microscope.
2.5.3. Mitochondrial activity.
To evaluate the cell mitochondrial activity of living cells on MGHA and MGHA-NH2 3D scaffolds, as well as around them (in the bottoms of the cell culture wells) after 48 h of incubation, an MTT method was employed, based on the reduction of yellow 3[4,5-dimethylthiazol-2yl]-2,5-diphenyltetrazolium bromide to blue formazan.27 For this, the culture media were replaced with 1 mL of DMEM and 125 μL of 0.012 g L−1 MTT solution in PBS. Samples were incubated for 4 h at 37 °C and 5% CO2 under dark conditions. Then, the medium was removed and 500 μL of 0.4 N isopropanol–HCl were added. Finally, the absorbance was measured at 570 nm using a Helios Zeta UV-VIS spectrophotometer.
2.5.4. Alkaline phosphatase activity.
The alkaline phosphatase (ALP) activity of cells growing on the both MGHA and MGHA-NH2 scaffolds was used as the key differentiation marker in assessing expression of the osteoblast phenotype. ALP activity was measured using the Reddi and Huggins method based on the hydrolysis of p-nitrophenylphosphate to p-nitrophenol.28 For this, MC3T3 cells (5 × 104 cells per mL) were seeded directly onto scaffolds in 24-well plates and incubated under standard culture conditions using supplemented medium with β-glycerolphosphate (50 μg mL−1, Sigma Chemical Company, St. Louis, MO, USA) and L-ascorbic acid (10 mM, Sigma Chemical Company, St. Louis, MO, USA). In order to evaluate ALP activity both in and around the scaffolds after 7 days of incubation, each scaffold was transferred to a new well. Moreover, the total protein value after 7 days was determined by a colorimetric method at 540 nm (Bio-Analítica, S.L.), using a Helios Zeta UV-VIS spectrophotometer.
2.6. Statistics
Data are expressed as means ± standard deviations of three experiments. Statistical analysis was performed using the Statistical Package for the Social Sciences (SPSS) version 11.5 software. Statistical comparisons were made by analysis of variance (ANOVA). The Scheffé test was used for post hoc evaluations of differences among groups. In all statistical evaluations, p < 0.05 was considered as statistically significant.
3. Results and discussion
3.1. 3D MGHA scaffolds and cell colonization
The macroporosity of the 3D MGHA scaffolds obtained by the RP technique was characterized by SEM and Hg intrusion porosimetry. The digital photograph (inset Fig. 1A) displays the image of a cylindrical piece of MGHA scaffold. The SEM micrograph (Fig. 1A) shows that MGHA scaffolds consist of a lattice of rods stacked with simple tetragonal symmetry according to the RP computer-aided design, where twenty layers of mesh were stacked to a total thickness of ca. 2 mm, with a diameter of ≈6.6 mm. These scaffolds present a hierarchical pore network with macropores around 500 μm connected by rods of 250–300 μm diameter, corresponding to the porosity required for tissue growth and vascularization in the human body.29,30 The higher magnification SEM micrograph, corresponding to a scaffold cross-section (Fig. 1B), shows notable differences between the interior and exterior scaffold surface. While the external surface exhibits cylinders with relatively smooth topography and a small fraction of pores in the range between 1 and 10 μm, the interior surface exhibits numerous pores in the range of 30 to 80 μm, resulting from the MC calcination process.24,25 Moreover, Hg intrusion porosimetry shows that the internal larger pores are accessible to the liquid probe, indicating well-connected macroporous systems (data not shown). Total percentage porosity is 60%, mainly in the 1–10 μm and 100–300 μm range according to SEM images. Low angle XRD patterns of these scaffolds (Fig. 1C) show three diffraction maxima at 0.86, 1.43, and 1.67° (2θ), which can be indexed to (10), (11), and (20) reflections of a 2D hexagonal structure (P6mm plane group) based on TEM results (Fig. 1D). N2 isotherms (Table 1) demonstrate the presence of a very narrow distribution of large mesopores centred at 10 nm, with a surface area and pore volume of 123 m2 g−1 and 0.2 cm3 g−1, respectively. Compared to the powder MGHA nanocomposite, the results obtained for the 3D MGHA scaffolds reveal a notable increase in pore size, from 8.0 nm for the powder nanocomposite22 to 10 nm for the scaffold, accompanied by a decrease both in the surface area and pore volume. This increase in pore size can be attributed to MC incorporation during the synthesis process.31
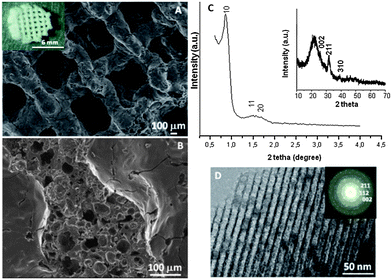 |
| Fig. 1 Macro- and mesostructural characterization of the hierarchical interconnected MGHA nanocomposite 3D scaffold. Surface (A) and cross-transversal section (B) obtained by SEM. (Inset) Digital photograph of a MGHA scaffold. (C) XRD pattern at low scattering angles showing the ordered mesoporous arrangement. (Inset) XRD pattern at wide scattering angles demonstrates the presence of the apatite phase. (D) TEM image showing the homogeneous distribution of the nanocrystalline apatite particles in the 2D hexagonal mesoporous structure. (Inset) ED pattern. | |
Table 1 Textural parameters corresponding to MGHA scaffolds before and after amine functionalization. The amount of aminopropyl moieties covalently bound to the scaffolds was determined by CHN elemental analyses and ζ-potential under physiological pH conditions
Sample |
S
BET (m2 g−1) |
V
p (cm3 g−1) |
D
p (nm) |
mmol APTES m−2 |
ζ (mV) |
Corresponds to the powder MGHA nanocomposite previously reported in ref. 22.
|
Powder MGHAa |
235 |
0.4 |
8.0 |
— |
−25 ± 5 |
MGHA scaffold |
123 |
0.2 |
10.0 |
— |
−35 ± 6 |
MGHA-NH2 scaffold |
101 |
0.2 |
8.6 |
0.02 |
+8 ± 3 |
To confirm the presence of nanocrystalline apatite in the 3D MGHA scaffolds, XRD and TEM studies were carried out. Wide-angle XRD patterns (inset, Fig. 1C) show very broad (002), (211) and (310) reflections, which could be attributed to apatite occurrence.32 Furthermore, TEM studies (Fig. 1D) showed a small darker zone homogeneously distributed in the mesoporous structure corresponding to nanocrystalline apatite phase particles. The electron diffraction (inset) of the dark zone displayed diffuse halos, showing d-spacing at 0.28, 0.27, and 0.34 nm, corresponding to the apatite phase. This confirms the nanocomposite structure of scaffolds, indicating that the incorporation of the MC polymer in the synthesis for the scaffolding process did not affect the biphasic structure of MGHA.
Therefore, the single-step sol–gel route synthesis in the presence of a surfactant (F127) as the mesostructure directing agent and a biomacromolecular polymer (methylcellulose) as the macrostructure template, followed by the RP technique, allows the formation of the hierarchical interconnected 3D scaffolds exhibiting different scales of porosity: (i) highly ordered mesopores with diameters of ca.10 nm that may act as local controlled delivery systems of biologically active molecules, and allow better nutrient diffusion;33–35 (ii) interconnected macropores with diameters in the 1–80 μm, and (iii) ultra-large macropores of ca. 500 μm, that may provide the opportunities for cell colonization.29 Furthermore, the presence of nanocrystalline apatite particles homogeneously distributed along the composite constitutes one more common element with the bone structure and may influence and control protein interactions through its surface properties, guiding cellular responses as they occur in the natural bone.36 Thus, this combination of the macroporous architecture, the nanoscale structure of the surface, and the presence of apatite particles may provide real benefits for MGHA nanocomposite scaffolds in bone tissue regeneration.
To investigate whether the MGHA scaffold architecture and composition would permit good bone integration, cell morphology, spreading degree, and internalization of MC3T3 preosteoblasts were studied after 2 days culture at different levels (depth) inside the macroporous structure. L1 label indicates the outer level, while L2 and L3 correspond to inner levels of macropores. Fig. 2A shows a low magnification SEM micrograph evidencing the presence of cells anchored through filopodia projections (inset) on the surface of L1, L2, and L3 zones, demonstrating that the MGHA scaffolds are colonized over the entire visible surface. Fig. 2B–D provide details of the three levels in which cells exhibit a typical morphology of this cell type.37
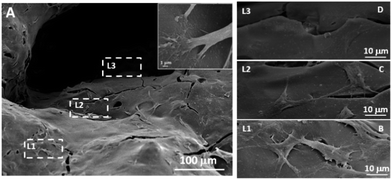 |
| Fig. 2 SEM studies of 3D MGHA scaffold colonization by MC3T3 preosteoblasts. (A) Low magnification micrograph of cell adhesion and colonization at different macroporous levels (L1, L2, and L3) of the scaffold. (Inset) cell anchoring in detail. (B)–(D) Higher magnifications micrographs showing the details of spreading MC3T3 cells at these macroporous levels. All micrographs are representative of the study. | |
The obtained results revealed adequate cell colonization over the MGHA scaffold surface. A commonly used approach for making the scaffold surface more conductive to cell adhesion is its preconditioning with cell adhesive peptides or proteins.16,17 In the present study, without any such preconditioning, the chemical and structural characteristics of these MGHA scaffolds are proved to be excellent in terms of biocompatibility. However, the cell number at the inner levels of these scaffolds could be improved by a suitable surface treatment to enhance cell colonization.
3.2. Amine functionalization of the 3D scaffold surface and its influence on cell adhesion, mitochondrial activity and cell differentiation
Since it has been demonstrated that the surface chemistry of a material exerts an important influence on cell response,7 amine functionalization of the 3D MGHA scaffold surface was carried out. Fig. 3 displays FTIR spectra before and after amine functionalization, both spectra exhibiting bands corresponding to the MGHA nanocomposite material. Thus, the bands of Si–O bonds at 1040, 800, and 470 cm−1 correspond to the amorphous silica mesoporous glass, while the doublet at 560 and 600 cm−1 is characteristic of crystalline phosphate.38 After amine functionalization, the FTIR spectrum of the MGHA-NH2 scaffold shows the appearance of new bands at 3344 and ca. 1500 cm−1, corresponding to NH stretching and deformation frequencies, respectively, whereas bands at 3030 and ≈1590 cm−1 are attributed to –NH3+ stretching and deformation frequencies, respectively.39,40 Moreover, the band at 2980 cm−1 corresponds to –CH2– groups, the band at 1260 cm−1 to the Si–C bond, and the small band at 1300 cm−1 to the C–N bond, confirming also the presence of organosilane (APTES).40
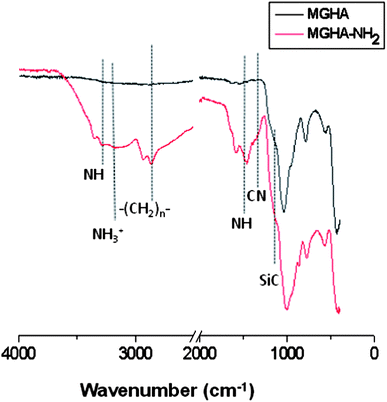 |
| Fig. 3 FTIR spectra corresponding to the 3D MGHA scaffold before and after amine functionalization. | |
To quantify the number of amine groups present on the surface of the MGHA-NH2 scaffold, a CHNS elemental chemical analysis was carried out. The experimental data indicated 0.02 mmol of APTES per square meter surface. Textural properties after the functionalization process revealed a slight decrease in the surface area, and pore diameter values (Table 1), indicating the incorporation of amino propyl silane moieties, maintaining the pore volume. Moreover, concerning meso–macroporosity, XRD, TEM, SEM, and Hg intrusion studies demonstrated that the both meso–macrostructural parameters were not altered after functionalization (data not shown).
29Si and 31P solid state SP and CP/MAS NMR measurements were carried out to further investigate the grafting of aminopropyl moieties to the MGHA scaffold surface as well as their condensation degree. Fig. 4 displays 29Si MAS NMR spectra recorded from MGHA and MGHA-NH2 3D scaffolds using single-pulse excitation, together with their component lines obtained from spectral deconvolutions. Both spectra show resonances at ca. −102 and −112 ppm, corresponding to Q3[Si(OSi)3(OX)], and Q4[Si(OSi)4] silicon sites, respectively (X = H, C). No significant changes are observed in Q3/Q4 relative populations after the functionalization process. The presence of signals attributable to Tn units [R–Si(OSi)n–(OX)3−n] (X = H, C) are indicative of the organosilane groups, only evidenced in the MGHA-NH2 3D scaffold. The presence of T3 and T2 signals demonstrates the existence of covalent linkages between the silica surface and the organic groups.41Table 2 confirms that no significant changes occur in the Q2/Q3/Q4 and q2/q1/q0 relative populations for 29Si and 31P environments after the functionalization process.
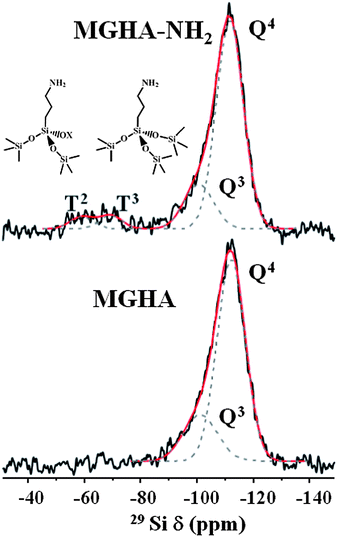 |
| Fig. 4 Solid-state 29Si single-pulse MAS NMR spectra (with their Qn and Tn silicon environments) corresponding to the 3D MGHA scaffold before and after amine functionalization. The areas for the Qn and Tn units were calculated by Gaussian line-shape deconvolutions. | |
Table 2 Population percent of 29Si and 31P environments resulting in deconvolution of the experimental NMR spectra recorded by cross-polarization (CP) corresponding to unmodified and functionalized scaffolds
Sample |
29Si CP |
31P CP |
T2 (%) |
T3 (%) |
QH2a (%) |
QH3 (%) |
Q4 (%) |
q0 (%) |
q1 (%) |
q2 (%) |
This NMR signal may comprise minor contributions from QCa2 tetrahedral.22
|
MGHA |
— |
— |
19.7 |
57.2 |
23.1 |
65.7 |
32.2 |
2.1 |
MGHA-NH2 |
45 |
55 |
16.8 |
46.8 |
36.4 |
55.5 |
35.7 |
8.8 |
Solid state 13C CP/MAS NMR spectra were collected to confirm the surface functionalization of MGHA scaffolds and to get information concerning the ionization state of amine (Table 3). As expected, no signals are observed in the 13C NMR spectrum of the unmodified MGHA scaffold (data not shown). In contrast, well-defined signals at 11, 24, and 45 ppm are observed after amine functionalization, which can be assigned to carbon atoms from APTES in three different environments (Table 3). Note that there is no resonance at ca. 28 and 85 ppm which would be attributed to the central carbon of the 3-aminopropyl chain of APTES with the –NH2 group as the nonprotonated form. The resonance appearing in the region of stronger fields, at 24 ppm, assignable to the central carbon of APTES, evidences the protonation of 3-aminopropyl groups, as it has also been confirmed by FTIR data.42,43
Table 3 Data of solid state 13C CP/MAS NMR corresponding to the MGHA-NH2 3D scaffold
MGHA-NH2 |
δ (ppm) |
Assignment |
11 |
Si–CH2–CH2–CH2–NH2 |
24 |
Si–CH2–CH2–CH2–NH3+ |
45 |
Si–CH2–CH2–CH2–NH2 |
With the chemical nature of scaffolds clarified, their charge in aqueous media was investigated by ζ-potential measurements. Fig. 5A displays the ζ-potential of MGHA and MGHA-NH2 scaffolds as a function of pH, and reveals notable differences before and after amine functionalization. Thus, at physiological pH 7.4, the ζ-potential was ≈−35 mV for the unmodified MGHA scaffold. This negative surface charge is due to the presence of deprotonated species, i.e., Si–O− (pKa = 4.5) from mesoporous glass and P–O− species (pKa = 6.5) from apatite clusters. For MGHA-NH2 scaffolds, on the other hand, the ζ-potential at pH 7.4 was +8 mV due to the presence of NH3+species (pKa = 10.0). To confirm the pKa values corresponding to all functional groups present in the MGHA-NH2 scaffold, a titration curve was carried out between pH values from 10 to 4. Fig. 5B demonstrates that, in agreement with expected bibliographic data, pKa (NH3+) is 10.0; pKa (POH) is 6.3, and pKa (SiOH) is 4.5. These results demonstrate that at physiological pH (7.4), the majority species present in the surface scaffold could correspond to NH3+, PO−, and SiO− moieties.
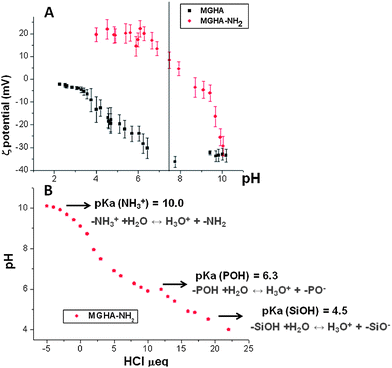 |
| Fig. 5 (A) ζ-potential curves as a function of pH before and after amine functionalisation of MGHA nanocomposite 3D scaffolds. (B) Titration curve with HCl corresponding to MGHA-NH2. | |
When cell adhesion on MGHA-NH2 scaffolds was analyzed by SEM after 2 days culture, a multilayer of MC3T3 cells anchored through filopodia projections (inset in Fig. 6) was observed throughout the surface of these scaffolds (Fig. 6A). Compared to MGHA scaffolds (Fig. 2), a larger number of cells are observed colonizing at all studied levels (L1, L2, and L3; Fig. 6B–D, respectively) of the MGHA-NH2 scaffold. In all cases there is multilayer cell growth, also at the inner level (L3). This multilayer growth at all levels demonstrates intercellular communication which constitutes a fundamental pillar for total scaffold colonization, ensuring new bone in-growth.44
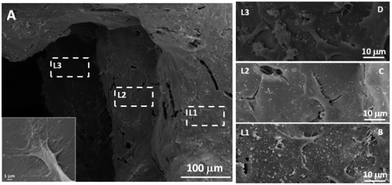 |
| Fig. 6 (A) SEM studies of MGHA-NH2 scaffold colonization by MC3T3 preosteoblasts showing internalization and cell spreading of preosteoblast MC3T3 cells cultured for 2 days on the amine functionalized 3D-MGHA scaffold at different macropore levels (L1, L2, and L3). Inset: detail of the filopodia projections. (B–D) Higher magnification SEM micrographs of spreading MC3T3 cells at the different macropore levels. All micrographs are representative of the study. | |
This notable enhancement of cell colonization on MGHA-NH2 with respect to unmodified MGHA scaffolds could be explained by the different surface chemistry of these samples. At physiological pH (7.4), the MGHA scaffold surface is negatively charged (−35 mV) due to the presence of SiO− and PO− groups, whereas after amine functionalization (MGHA-NH2), the scaffold surface attains a positive surface charge (+8 mV) because of the presence of NH3+ groups. Thus, there is an increased direct electrostatic attractive interaction between cationic surfaces and negatively charged membrane cells.45 In addition, the positive charge promotes adsorption of culture medium proteins, which are involved in the non-specific early stage of cell adhesion.18 To confirm the role of the surface amine groups in protein adsorption, ellipsometry studies with both surfaces, MGHA and MGHA-NH2, were carried out. In analogy to the increased cell adhesion, the amine functionalized surfaces display increased adsorption of serum proteins, exemplified for fibrinogen in Fig. 7. As can be seen, adsorption of this important serum protein is much higher for the cationic MGHA-NH2 compared to unmodified and negatively charged MGHA. This is a reflection of the net negative charge of fibrinogen under these conditions (IEP ≈ 5.5),46 promoting its adsorption to the oppositely charged MGHA-NH2 surface. This is in agreement with similar findings obtained for surfaces rendered cationic also by other approaches.47 Particularly, for serum proteins stimulating cell adhesion, such as fibrinogen and fibronectin, the mechanism for promoting increased cell adhesion and spreading of the MGHA-NH2 scaffold is likely facilitated by the formation of a conditioning protein layer.
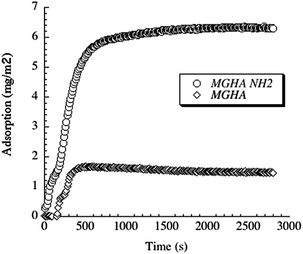 |
| Fig. 7 Fibrinogen adsorption to MGHA and MGHA-NH2 surfaces detected by ellipsometry. | |
Moreover, in order to investigate the broader potential of these 3D scaffolds, other cell parameters as mitochondrial activity and cell differentiation were evaluated. The results show a significant increase of mitochondrial activity (2.3 folds, p ≤ 0.05) when the scaffold surface is previously amine functionalized (Fig. 8A) which is due to the higher number of cells on these scaffolds. Concerning MC3T3 preosteoblast differentiation, evaluated after 7 days culture by ALP activity per total protein, the results reveal a significant increase (4.8 folds, p < 0.05) of this cell differentiation parameter on MGHA-NH2 scaffolds with respect to MGHA scaffolds and TCP control (Fig. 8B). Non-significant differences between the MGHA scaffold and TCP control were observed (p > 0.05).
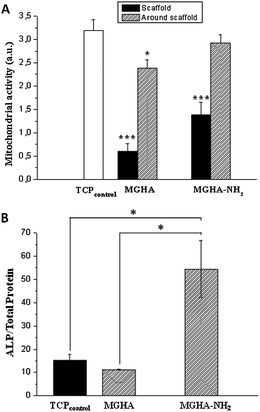 |
| Fig. 8 (A) Mitochondrial activity of preosteoblast MC3T3 cells on the surface scaffold and around the scaffold of unmodified and amine modified ones after 2 days of incubation. (B) ALP per total protein of preosteoblast MC3T3 cells on the surface scaffold of unmodified (MGHA) and amine modified (MGHA-NH2) ones after 7 days of incubation. Statistical significance: *p < 0.05, ***p < 0.005. | |
Taken together, these results thus demonstrate that both the structure design of the 3D MGHA scaffold at different length scales (macro and nanoscale) and the use of basic chemistry to incorporate simple amine functional groups to their surface enhance notably the cell adhesion, scaffold colonization and preosteoblast differentiation (Fig. 9).
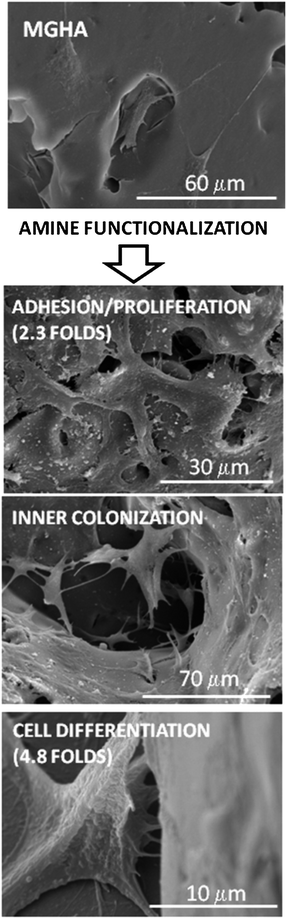 |
| Fig. 9 Summary of effects produced by amine functionalisation of MGHA nanocomposite 3D scaffolds. | |
Conclusions
3D scaffolds based on a new nanocomposite (MGHA) composed of hydroxyapatite nanocrystals embedded into mesoporous glass have been fabricated through a rapid prototyping technique. The 3D MGHA scaffolds exhibit three scales of porosity: ordered mesopores (10 nm), interconnected macropores (1–80 μm), and ultra-large macropores (500 μm). This hierarchical meso–macroporous system is obtained by using a sol precursor containing different scale-length polymers (Pluronic F127 and methylcellulose as meso and macrostructure directing agents, respectively) together with a robocasting process. The surface properties of these MGHA scaffolds, concerning cell recognition have been enhanced through a direct functionalization with aminopropyl moieties. This simple and cost-effective amine modification causes noticeable improvements in the preosteoblast adhesion, as well as a double increase in cell proliferation and a quadruple increase in cell differentiation. Moreover, ellipsometry studies demonstrated that the protonated amino propyl moieties favour the early step in the adhesion process by an increase in the nonspecific protein adsorption. Therefore, these studies reveal that both the structure design of hierarchical meso–macroporous 3D MGHA scaffolds and the amine chemical modification of their surface significantly improve the biocompatibility of these nanocomposite scaffolds.
Acknowledgements
This study was supported by research grants from Comunidad de Madrid (S2009/MAT-1472) and Ministerio de Ciencia e Innovacion (MICINN) through the projects MAT2012-35556 and CS2010-11384 E. M. Cicuéndez is grateful to MICINN for the financial support through the FPI fellowship. The authors wish to thank also the staff of the ICTS Centro Nacional de Microscopia Electrónica (Spain) and Centro de Citometría y Microscopia de Fluorescencia, Centro de Difracción de Rayos X, Centro de Microanálisis Elemental and Centro de Resonancia Magnética Nuclear of the Universidad Complutense de Madrid (Spain).
Notes and references
- S. J. Hollister, Adv. Mater., 2009, 21, 330–3342 CrossRef PubMed.
- R. Langer and J. P. Vacanti, Science, 1993, 260, 920–926 CAS.
- T. G. Kim, H. Sim and D. W. Lin, Adv. Funct. Mater., 2012, 22, 2446–2468 CrossRef CAS.
- X. Yu, Z. Xia, L. Wang, F. Peng, X. Jiang, J. Huang, D. Rowed and M. Wei, J. Mater. Chem., 2012, 22, 9721–9730 RSC.
- V. Karageorgiou and D. Kaplan, Biomaterials, 2005, 26, 5474–5491 CrossRef CAS PubMed.
- M. Vallet-Regí, Chem.–Eur. J., 2006, 12, 5934–5943 CrossRef PubMed.
- M. M. Stevens and J. H. George, Science, 2005, 310, 1135–1138 CrossRef CAS PubMed.
- M. Vallet-Regi and J. M. Gonzalez-Calbet, Prog. Solid State Chem., 2004, 32, 1–31 CrossRef CAS PubMed.
- L. Xia, K. Lin, X. Jiang, Y. Xu, M. Zhang, J. Chang and Z. Zhang, J. Mater. Chem. B, 2013, 1, 5403–5416 RSC.
- M. Vallet-Regí, M. Colilla and I. Izquierdo-Barba, J. Biomed. Nanotechnol., 2008, 4, 1–15 Search PubMed.
- K. Anselme, Biomaterials, 2000, 21, 667–681 CrossRef CAS.
- B. Geiger, J. P. Spatz and A. D. Bershadsky, Nat. Rev. Mol. Cell Biol., 2009, 10, 21–33 CrossRef CAS PubMed.
- M. Cohen, D. Joester, I. Sabanay, L. Addadi and B. Geiger, Soft Matter, 2007, 3, 327–332 RSC.
- S. P. Evanko, M. I. Tammi, R. H. Tammi and T. N. Wight, Adv. Drug Delivery Rev., 2007, 59, 1351–1365 CrossRef CAS PubMed.
- J. P. Xiong, T. Stehle, R. G. Zhang, A. Joachimiak, M. Frech, S. L. Goodman and M. A. Arnaout, Science, 2002, 296, 151–155 CrossRef CAS PubMed.
- M. D. Pierschbacher and E. Ruoslahti, Nature, 1984, 308, 30–33 CrossRef.
- U. Hersel, C. Dahmen and H. Kessler, Biomaterials, 2003, 24, 4385–4415 CrossRef CAS.
- Y. Lai, C. Xie, Z. Zhang, W. Lu and J. Ding, Biomaterials, 2010, 31, 4809–4817 CrossRef CAS PubMed.
- M. Cohen, D. Joester, B. Geiger and L. Addadi, ChemBioChem, 2004, 5, 1393–1399 CrossRef CAS PubMed.
- J. M. Curran, R. Chen and J. A. Hunt, Biomaterials, 2010, 31, 1463–1464 CrossRef CAS PubMed.
- D. Trimbach, B. Keller, R. Bhat, S. Zankovych, R. Pohlmann, S. Schroter, J. Bosert and K. D. Jandt, Adv. Funct. Mater., 2008, 18, 1723–1731 CrossRef CAS.
- M. Cicuéndez, M. T. Portolés, I. Izquierdo-Barba and M. Vallet-Regí, Chem. Mater., 2012, 24, 1100–1106 CrossRef.
- A. Butscher, M. Bohner, S. Hofmann, L. Gauckler and R. Muller, Acta Biomater., 2011, 7, 907–920 CrossRef CAS PubMed.
- A. García, I. Izquierdo-Barba, M. Colilla, C. López de Laorden and M. Vallet-Regí, Acta Biomater., 2011, 7, 1265–1273 CrossRef PubMed.
- H. S. Yun, S. E. Kim and Y. T. Hyeon, Chem. Commun., 2007, 2139–2141 RSC.
- M. Malmsten, J. Colloid Interface Sci., 1994, 166, 333–342 CrossRef CAS.
- M. Cicuéndez, I. Izquierdo-Barba, S. Sánchez-Salcedo, M. Vila and M. Vallet-Regí, Acta Biomater., 2012, 8, 802–810 CrossRef PubMed.
- A. H. Reddi and C. B. Huggins, Proc. Soc. Exp. Biol. Med., 1972, 140, 807–810 CrossRef CAS.
- S. J. Hollister, Nat. Mater., 2005, 4, 518–524 CrossRef CAS PubMed.
- J. R. Jones, L. M. Ehrenfried and L. L. Hench, Biomaterials, 2006, 27, 964–973 CrossRef CAS PubMed.
- I. Medved and R. Cerny, Microporous Mesoporous Mater., 2011, 142, 405–422 CrossRef CAS PubMed.
- S. Padilla, I. Izquierdo-Barba and M. Vallet-Regí, Chem. Mater., 2008, 20, 5942–5944 CrossRef CAS.
- M. Vallet-Regí, J. Intern. Med., 2010, 267, 22–43 CrossRef PubMed.
- M. Vallet-Regi, ISRN Mater. Sci., 2012, 20 Search PubMed.
- M. Cicuéndez, I. Izquierdo-Barba, M. Portolés and M. Vallet-Regí, Eur. J. Pharm. Biopharm., 2013, 84, 115–124 CrossRef PubMed.
- T. J. Webster, C. Ergun, R. H. Doremus, R. W. Siegel and R. Bizios, J. Biomed. Mater. Res., Part A, 2000, 51, 475–483 CrossRef CAS.
- S. F. Lamolle, M. Monjo, M. Rubert, H. J. Haugen, S. P. Lyngstadaas and J. E. Ellingsen, Biomaterials, 2009, 30, 736–742 CrossRef CAS PubMed.
- R. Z. Legeros, Clin. Orthop. Relat. Res., 2002, 395, 81–98 CrossRef PubMed.
- F. Zhang and M. P. Srinivasan, Langmuir, 2004, 20, 2309–2314 CrossRef CAS.
- M. Colilla, I. Izquierdo-Barba, S. Sanchez-Salcedo, J. L. G. Fierro, J. L. Hueso and M. Vallet-Regi, Chem. Mater., 2010, 22, 6459–6466 CrossRef CAS.
- M. P. J. Peeters, W. J. J. Wakelkamp and A. P. M. Kentgens, J. Non-Cryst. Solids, 1995, 189, 77–84 CrossRef CAS.
-
P. Innocenzi, Y. L. Zub and V. G. Kessler, Sol–gel methods for materials processing, Springer Science, Netherlands, 2008 Search PubMed.
- S. Sánchez-Salcedo, M. Colilla, I. Izquierdo-Barba and M. Vallet-Regí, J. Mater. Chem. B, 2013, 1, 1595–1606 RSC.
- L. G. Griffith and G. Naughton, Science, 2002, 295, 1009–1014 CrossRef CAS PubMed.
- N. G. Maroudas, J. Theor. Biol., 1975, 49, 417–424 CAS.
- H. A. Scheraga and J. R. Laskowski, Adv. Protein Chem., 1957, 12, 2–5 CrossRef.
- B. Lassen and M. Malmsten, J. Colloid Interface Sci., 1997, 186, 9–16 CrossRef CAS.
|
This journal is © The Royal Society of Chemistry 2014 |
Click here to see how this site uses Cookies. View our privacy policy here.