DOI:
10.1039/C4SC02377C
(Edge Article)
Chem. Sci., 2015,
6, 259-263
An aqueous molecular tube with polyaromatic frameworks capable of binding fluorescent dyes†
Received
6th August 2014
, Accepted 12th September 2014
First published on 12th September 2014
Abstract
An aqueous molecular tube composed of polyaromatic frameworks with peripheral hydrophilic groups was prepared. The new tube has a well-defined hydrophobic cavity with a diameter of ∼1 nm and quantitatively binds two molecules of fluorescent coumarin dyes in aqueous solutions. The bound coumarin dimers in a stacked fashion exhibit unusual excimer-like emissions in the confined space through efficient host–guest energy transfer.
Introduction
Molecular rings and tubes composed of entirely aromatic frameworks, as contrasted with well-studied aliphatic ones (e.g., cyclodextrins and cucurbiturils), have recently attracted greater attention from the viewpoint of both synthetic and physical chemistries due to their relevance to the partial structures of single-walled carbon nanotubes.1,2 Such aromatic macrocycles have been prepared by linking multiple benzene, naphthalene, pyrene, or chrysene rings3,4 through transition metal-mediated coupling reactions and they exhibit intriguing fluorescence properties based on their aromatic frameworks. However, their host–guest interactions have been limited to large spherical molecules, i.e. fullerenes,5 probably due to their large hydrophobic nature. Moreover, the supramolecular host–guest photo-phenomena of the aromatic macrocycles, as well as previous aliphatic tubes, have been relatively unexplored.6,7 We anticipated that the development of water-soluble molecular tubes with polyaromatic frameworks would lead to establishment of new photofunctional molecular flasks, providing wide-ranging host capabilities and unique host–guest photophysical properties (Fig. 1a). Here we present the preparation of a new aqueous molecular tube 1 with polyaromatic anthracene panels and peripheral hydrophilic sulfonate groups (R = –OCH2CH2CH2SO3−·Na+; Fig. 1b). The tube quantitatively binds two molecules of fluorescent coumarin dyes in aqueous solutions at room temperature. We emphasize that the bound coumarin dimers exhibit unusual excimer-like emissions in the confined tubular cavity through efficient energy transfer from the host framework to the guest dimers.
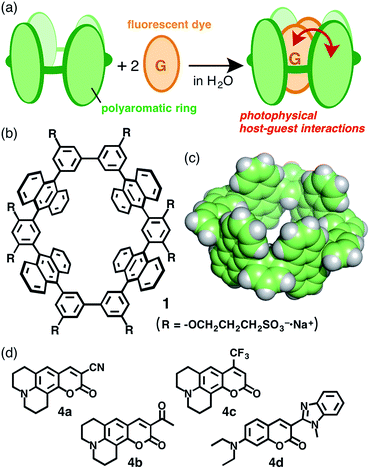 |
| Fig. 1 (a) Schematic representation of the encapsulation of guest molecules by an aqueous molecular tube with polyaromatic frameworks. (b) Aqueous molecular tube 1 and (c) the polyaromatic framework (R = –H). (d) Coumarin dyes 4a–d employed as guest molecules. | |
Recently, we have reported molecular tube 1′ (R = –OCH3 and –OCH2CH2OCH3) composed of four anthracene panels8,9 linked by phenylene and biphenylene spacers at the meta positions (Fig. 1b).10 The tubular structure has a well-defined 1 nm-sized cavity surrounded by hydrophobic anthracene frameworks (Fig. 1c), however its synthetic protocol demands a multistep preparation (15 steps; Scheme S1†) including a cross-coupling reaction between triflate-capped and boronate-capped half-tubes, which limits its further exploration. Besides, no host–guest interactions have been observed for the hydrophobic tube owing to its solubility in only organic solvents (e.g., CHCl3 and acetone).
Results and discussion
Synthesis of an aqueous molecular tube
Water-soluble molecular tube 1 was obtained from anthracene dimer 3a by the following straightforward synthetic route, which is 7-steps shorter than the previous route and includes bromo-capped half-tube 2a as a key intermediate (Fig. 2 and ESI†). After the iodination of 3a, the Suzuki–Miyaura coupling of 3b (X = –I) with 3-bromo-5-methoxyphenyl boronate afforded 2a in 54% yield. After the methoxy groups on 2a were converted into 2-methoxymethoxy groups, a homo-coupling reaction of the methoxymethoxy-protected 2b using a Ni(cod)2/bipyridine catalyst11 gave rise to tube 1′′ in a moderate yield (30%). Conversion of the peripheral substituents on 1′′ to hydrophilic sulfonate groups by sequential deprotection and etherification gave rise to aqueous tube 1 in 50% yield (2 steps).
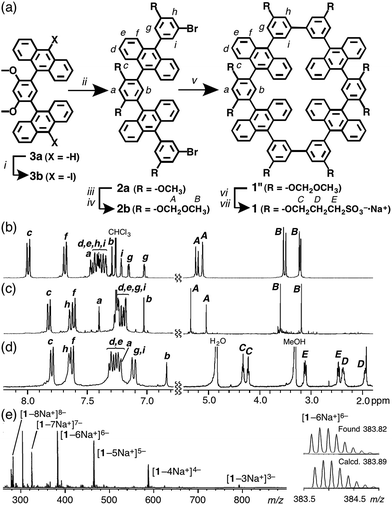 |
| Fig. 2 (a) Preparation of aqueous molecular tube 1. Reagents and catalysts: (i) 1,3-diiodo-5,5-dimethylhydantoin; (ii) 3-bromo-5-methoxyphenylboronic acid pinacol ester, Pd(PPh3)4, Na2CO3; (iii) BBr3; (iv) chloromethyl methyl ether, Cs2CO3; (v) Ni(cod)2, 2,2′-bipyridyl; (vi) HClaq.; (vii) 1,3-propanesultone, NaH. 1H NMR spectra (400 MHz, r.t.) of (b) bromo-capped half-tube 2b and (c) hydrophobic tube 1′′ in CDCl3, and (d) aqueous tube 1 in CD3OD. (e) ESI-TOF MS spectrum of aqueous tube 1 in CH3OH with an expansion and simulation of the [1–6Na+]6− signal. | |
The structures of molecular tubes 1 and half-tubes 2 were fully confirmed by NMR spectroscopy and mass spectrometry.† The 1H NMR spectrum of tube precursor 2b (Fig. 2b) showed the corresponding aromatic Ha–i and aliphatic HA,B signals as assigned by COSY and NOESY analyses. Characteristically, two sets of proton signals derived from the functionalized phenyl moieties (Hg,i and HA,B) were observed in a 1
:
1 ratio.12 On the other hand, the 1H NMR analysis of 1′′ showed a simple spectrum displaying nine aromatic and four aliphatic signals (Fig. 2c). This spectral change is reasonable upon the formation of tubular compound 1′′ with D2h symmetry. Matrix-assisted laser desorption ionization time-of-flight mass spectrometry (MALDI-TOF MS) revealed the formation of 1′′ with a prominent peak at m/z = 1641.44. After isolation of aqueous tube 1, the 1H NMR spectrum in CD3OD exhibited new aliphatic signals attributed to the decorated sulfonate groups (HC–E) in the range 4.4–1.8 ppm (Fig. 2d). Electrospray ionization (ESI)-TOF MS spectrum of 1 showed a series of intense peaks at e.g., 791.0 [1–3Na+]3−, 587.2 [1–4Na+]4−, and 465.2 [1–5Na+]5− (Fig. 2e), which unambiguously elucidated full modification of the tube by the hydrophilic groups.
Obtained sulfonate-coated tube 1 is highly soluble in water (>100 mM) but tubes 1′ and 1′′ are effectively insoluble in water. In the 1H NMR spectrum of 1 in D2O, the proton signals were significantly broadened (Fig. S20†), most probably originating from slow intramolecular motion on the NMR timescale. Dynamic light scattering (DLS) analysis of aqueous solutions of 1 showed small particles with an average diameter of 1.4 nm (Fig. S21a†), which indicates no aggregation of 1 in water. An optimized structure of 1 shows that its external and core diameters are approximately 1.9 and 1.0 nm, respectively (Fig. 3a).
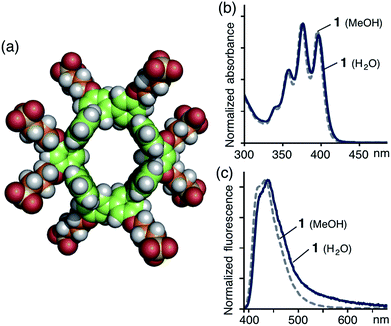 |
| Fig. 3 (a) An optimized structure of aqueous tube 1 without counterions or solvents by PM3 calculation. (b) UV-vis spectra (10 μM, r.t.) and (c) fluorescence spectra (λex = 378 nm, 10 μM, r.t.) of 1 in H2O (solid line) and CH3OH (dashed line). | |
The UV-vis spectrum of tube 1 in H2O exhibited the typical π–π* transition bands of the anthracene moieties (Fig. 3b). The emission spectra of 1 in H2O and CH3OH displayed a broad band in the range 390–550 nm (λmax = ∼440 nm; Fig. 3c) with good absolute quantum yields (ΦF = 30 and 59%, respectively).† Thus, the obtained polyaromatic nanotube providing both good water-solubility and fluorescence properties would be expected to act as a photofunctional molecular host in aqueous media.
Encapsulation of coumarin dyes
The open-ended and 1 nm-length cylindrical cavity of tube 1 facilitated the quantitative encapsulation of two molecules of coumarin 337 (4a) and 334 (4b), well-known hydrophobic fluorescent dyes, in aqueous solutions through hydrophobic and aromatic–aromatic interactions (Fig. 1a and d). When a slight excess of 3-cyano-substituted 4a was suspended in an aqueous solution (0.2 mM) of tube 1 at room temperature for 1 h, the color of the solution obviously changed from colorless to yellow due to the formation of a 1
:
2 host–guest complex, 1 ⊃ (4a)2, as revealed by UV-vis and MS analyses.13 The UV-vis absorption spectrum of the product showed a new prominent band at λmax = 446 nm corresponding to the bound coumarin guests (Fig. 4a). The band is considerably narrower than that of free 4a in CH3OH (Fig. S24†). It is worthy of note that another new absorption band was observed as a shoulder at around 480 nm, which can presumably be attributed to a π-stacked coumarin dimer. In combination with ESI-TOF MS analysis,14 a UV-vis spectral titration study clearly illustrated the quantitative formation of the 1
:
2 host–guest structure (Fig. 4b).† Similarly, two molecules of 3-acetyl-substituted coumarin 4b were quantitatively bound in the cavity of tube 1 to produce 1 ⊃ (4b)2, which showed an absorption band for the guests at λmax = 455 nm (Fig. 4a). Notably, the absorption bands of 4a and 4b overlap considerably with the emission band of 1 (Fig. 3c). An optimized structure of 1 ⊃(4a)2 (R = –OCH3) obtained by force-field calculations13,15 indicated that the two coumarin guests adopt a π-stacked anti-head-to-tail conformation with an interplanar distance of 3.5 Å in the cavity (Fig. 4c and d). The stacked dimer fully occupies the nanospace and is in tight contact with the four anthracene panels of the tube.
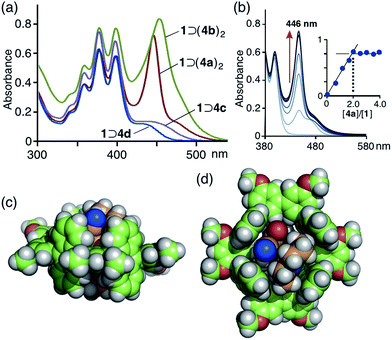 |
| Fig. 4 (a) UV-vis spectra (0.2 mM, H2O, r.t.) of 1 ⊃ (4a)2, 1 ⊃ (4b)2, 1 ⊃ 4c, and 1 ⊃ 4d. (b) Titration UV-vis spectra (0.2 mM, H2O, r.t.) and the plot (λabs = 446 nm) of 1 with the addition of 4a ([4a]/[1] = 0.5, 1.0, 1.5, 2.0, 2.5, 3.0, 3.5, 4.0). Optimized structure of 1 ⊃ (4a)2 (R = –OCH3): (c) side and (d) top views. | |
Interestingly, in the case of 4-trifluoromethyl-substituted coumarin 153 (4c) and 3-(N-methyl)benzimidazolyl-substituted coumarin 30 (4d), tube 1 recognized the substituents on the coumarin dyes and yielded 1
:
1 host–guest complexes.10 UV-vis and ESI-TOF MS studies revealed the formation of 1 ⊃ 4c and 1 ⊃ 4d (in 40 and 30% yields, respectively) under conditions similar to those used for 1 ⊃ (4a)2. The UV-vis spectra showed new absorption bands resulting from bound coumarins 4c and 4d at λmax = 437 and 430 nm, respectively (Fig. 4a). Force-field calculation studies suggested that the 4-trifluoromethyl and N-methylbenzimidazolyl groups on the coumarin dyes prevent the formation of 1
:
2 host–guest structures and efficient host–guest stacking interactions due to steric hindrance (Fig. S34 and S38†). The competitive binding experiment for tube 1 revealed the preferential binding series of 4a ≈ 4b ≫ 4c ≈ 4d for the coumarin guests.†
Unique host–guest photophysical properties
The aqueous solutions of 1
:
2 host–guest complexes 1 ⊃ (4a)2 and 1 ⊃ (4b)2 exhibited unusual emissions from the stacked coumarin dimers upon irradiation of the host framework at 378 nm at room temperature. The fluorescence spectrum of 1 ⊃ (4a)2 contained a new broad band at around 590 nm, assignable to an excimer-like emission from (4a)2 within 1,16 whereas emission from the anthracene moieties of 1 (∼440 nm) and the monomeric coumarin guest (∼510 nm) were markedly weaker (Fig. 5a) due to efficient fluorescence resonance energy transfer (FRET)17 from host 1 to guest (4a)2. Similarly, 1 ⊃ (4b)2 showed an excimer-like emission band at around 600 nm in the fluorescence spectrum, accompanying a weak emission band (∼500 nm) for the monomeric coumarin guest. In sharp contrast, the aqueous solution of a mixture of 1 ⊃ 4c and 1 displayed only host and guest emissions (∼440 and ∼510 nm, respectively) and a mixture of 1 ⊃ 4d and 1 displayed a prominent guest emission at λmax = 477 nm through FRET upon irradiation at 378 nm (Fig. 5b). The CIE chromaticity diagram for 1 ⊃ (4a)2 and 1 ⊃ (4b)2 quantified the emission colors as orange ((0.48, 0.44) and (0.48, 0.43), respectively), unlike that for 1, 4a, and 4b (Fig. 5c).
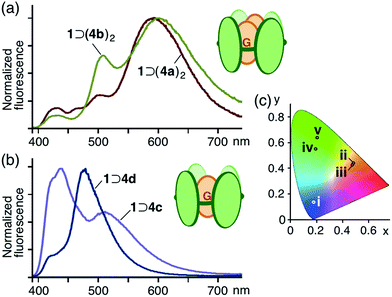 |
| Fig. 5 Fluorescence spectra (λex = 378 nm, H2O, r.t.) of (a) the 1 : 2 host–guest complexes and (b) the 1 : 1 host–guest complexes. (c) CIE chromaticity diagram of the fluorescence color of (i) 1, (ii) 1 ⊃ (4a)2, (iii) 1 ⊃ (4b)2 in H2O and (iv) 4a, (v) 4b in CH3OH. | |
The water-solubility of tube 1 is essential to both the strong binding of the hydrophobic fluorescent dyes and the unique emissions of the bound dyes through efficient host–guest and guest–guest interactions. When 1 ⊃ (4a)2 was dissolved in MeOH, the host–guest complex dissociated immediately. In the fluorescence spectrum, only emission bands from free 1 and 4a were observed upon irradiation at 378 nm (Fig. S24†).
Conclusions
In conclusion, we have succeeded in the preparation of a molecular tube with polyaromatic panels and peripheral hydrophilic groups. The new aqueous tube quantitatively binds two molecules of fluorescent coumarin dyes in its well-defined nanospace through hydrophobic and aromatic–aromatic interactions in water. Notably and most importantly, the bound fluorescent dyes in a stacked fashion exhibited excimer-like emission through efficient host–guest intermolecular interactions. This study demonstrates that photoactive and water-soluble polyaromatic tubes have potential for exploring novel host–guest supramolecular structures and subsequent unique photophysical properties.
Acknowledgements
This work was supported by the Japan Society for the Promotion of Science (JSPS) through a “Funding Program for Next-Generation World-Leading Researchers (NEXT Program)” and by the Japanese Ministry of Education, Culture, Sports, Science and Technology (MEXT) through Grants-in-Aid for Scientific Research on Innovative Areas “Soft Molecular Systems”. K.H. thanks the JSPS for a Research Fellowship for Young Scientists.
Notes and references
-
(a) S. Iijima, Nature, 1991, 354, 56–58 CrossRef CAS;
(b)
Carbon Nanotubes: Synthesis, Structure, Properties and Applications, ed. M. Dresselhaus, G. Dresselhaus and P. Avouris, Springer, Berlin, 2001 Search PubMed.
-
(a) T. Kawase and H. Kurata, Chem. Rev., 2006, 106, 5250–5273 CrossRef CAS PubMed;
(b) K. Tahara and Y. Tobe, Chem. Rev., 2006, 106, 5274–5290 CrossRef CAS PubMed;
(c) M. Iyoda, J. Yamakawa and M. J. Rahman, Angew. Chem., Int. Ed., 2011, 50, 10522–10553 CrossRef CAS PubMed;
(d) H. Omachi, Y. Segawa and K. Itami, Acc. Chem. Res., 2012, 45, 1378–1389 CrossRef CAS PubMed.
-
(a) C. Grave and A. D. Schlüter, Eur. J. Org. Chem., 2002, 3075–3098 CrossRef CAS;
(b) R. Jasti, J. Bhattacharjee, J. B. Neaton and C. R. Bertozzi, J. Am. Chem. Soc., 2008, 130, 17646–17647 CrossRef CAS PubMed;
(c) H. Takaba, H. Omachi, Y. Yamamoto, J. Bouffard and K. Itami, Angew. Chem., Int. Ed., 2009, 48, 6112–6116 CrossRef CAS PubMed;
(d) S. Yamago, Y. Watanabe and T. Iwamoto, Angew. Chem., Int. Ed., 2010, 49, 757–759 CrossRef CAS PubMed;
(e) T. Iwamoto, Y. Watanabe, Y. Sakamoto, T. Suzuki and S. Yamago, J. Am. Chem. Soc., 2011, 133, 8354–8361 CrossRef CAS PubMed;
(f) T. J. Sisto, M. R. Golder, E. S. Hirst and R. Jasti, J. Am. Chem. Soc., 2011, 133, 15800–15802 CrossRef CAS PubMed.
-
(a) A. Yagi, Y. Segawa and K. Itami, J. Am. Chem. Soc., 2012, 134, 2962–2965 CrossRef CAS PubMed;
(b) S. Hitosugi, W. Nakanishi, T. Yamasaki and H. Isobe, Nat. Commun., 2011, 2, 492 CrossRef;
(c) T. Iwamoto, E. Kayahara, N. Yasuda, T. Suzuki and S. Yamago, Angew. Chem., Int. Ed., 2014, 53, 6430–6434 CrossRef CAS PubMed.
-
(a) T. Iwamoto, Y. Watanabe, T. Sadahiro, T. Haino and S. Yamago, Angew. Chem., Int. Ed., 2011, 50, 8342–8344 CrossRef CAS PubMed;
(b) H. Isobe, S. Hitosugi, T. Yamasaki and R. Iizuka, Chem. Sci., 2013, 4, 1293–1297 RSC;
(c) S. Hitosugi, R. Iizuka, T. Yamasaki, R. Zhang, Y. Murata and H. Isobe, Org. Lett., 2013, 15, 3199–3201 CrossRef CAS PubMed;
(d) Y. Nakanishi, H. Omachi, S. Matsuura, Y. Miyata, R. Kitaura, Y. Segawa, K. Itami and H. Shinohara, Angew. Chem., Int. Ed., 2014, 53, 3102–3106 CrossRef CAS PubMed.
-
(a)
J. W. Steed and J. L. Atwood, Supramolecular Chemistry, Wiley, Chichester, U.K., 2nd edn, 2009 Search PubMed;
(b) F. Hof, S. L. Craig, C. Nuckolls and J. Rebek Jr, Angew. Chem., Int. Ed., 2002, 41, 1488–1508 CrossRef CAS;
(c) N. Sakai, J. Mareda and S. Matile, Acc. Chem. Res., 2008, 41, 1354–1365 CrossRef CAS PubMed;
(d) M. Yoshizawa, J. K. Klosterman and M. Fujita, Angew. Chem., Int. Ed., 2009, 48, 3418–3438 CrossRef CAS PubMed;
(e) H. Amouri, C. Desmarets and J. Moussa, Chem. Rev., 2012, 112, 2015–2041 CrossRef CAS PubMed;
(f) T. R. Cook, Y.-R. Zheng and P. J. Stang, Chem. Rev., 2013, 113, 734–777 CrossRef CAS PubMed;
(g) M. M. J. Smulders, I. A. Riddell, C. Browne and J. R. Nitschke, Chem. Soc. Rev., 2013, 42, 1728–1754 RSC;
(h) G. Zhang and M. Mastalerz, Chem. Soc. Rev., 2014, 43, 1934–1947 RSC.
- A recent water-soluble tube (without polyaromatic rings and fluorescent properties): Z. Li, J. Yang, G. Yu, J. He, Z. Abliz and F. Huang, Chem. Commun., 2014, 50, 2841–2843 RSC.
- Multiple anthracene assemblies:
(a) N. Kishi, Z. Li, K. Yoza, M. Akita and M. Yoshizawa, J. Am. Chem. Soc., 2011, 133, 11438–11441 CrossRef CAS PubMed;
(b) Z. Li, N. Kishi, K. Yoza, M. Akita and M. Yoshizawa, Chem.–Eur. J., 2012, 18, 8358–8365 CrossRef CAS PubMed;
(c) K. Kondo, A. Suzuki, M. Akita and M. Yoshizawa, Angew. Chem., Int. Ed., 2013, 52, 2308–2312 CrossRef CAS PubMed;
(d) A. Suzuki, K. Kondo, M. Akita and M. Yoshizawa, Angew. Chem., Int. Ed., 2013, 52, 8120–8123 CrossRef CAS PubMed;
(e) N. Kishi, Z. Li, Y. Sei, M. Akita, K. Yoza, J. S. Siegel and M. Yoshizawa, Chem.–Eur. J., 2013, 19, 6313–6320 CrossRef CAS PubMed;
(f) N. Kishi, M. Akita, M. Kamiya, S. Hayashi, H.-F. Hsu and M. Yoshizawa, J. Am. Chem. Soc., 2013, 135, 12976–12979 CrossRef CAS PubMed;
(g) N. Kishi, M. Akita and M. Yoshizawa, Angew. Chem., Int. Ed., 2014, 53, 3604–3607 CrossRef CAS PubMed;
(h) M. Yamashina, Y. Sei, M. Akita and M. Yoshizawa, Nat. Commun., 2014, 5, 4662 Search PubMed;
(i) K. Yazaki, Y. Sei, M. Akita and M. Yoshizawa, Nat. Commun., 2014, 5, 5179 Search PubMed.
- M. Yoshizawa and J. K. Klosterman, Chem. Soc. Rev., 2014, 43, 1885–1898 RSC.
- K. Hagiwara, Y. Sei, M. Akita and M. Yoshizawa, Chem. Commun., 2012, 48, 7678–7680 RSC.
- M. F. Semmelhack, P. M. Helquist and L. D. Jones, J. Am. Chem. Soc., 1971, 93, 5908–5910 CrossRef CAS.
- Because of the existence of three atropisomers caused by hindered rotation of the two anthryl–phenyl bonds.
- The proton NMR spectra of the host–guest complexes in D2O were also broadened and thus we confirmed the structures by detailed UV-vis and MS studies. Guests 4a–d are highly hydrophobic molecules and therefore we cannot determine the association constants under the heterogeneous conditions.
- The 1 ⊃ (4a)2 complex is stable enough under ESI-TOF MS conditions and therefore the spectrum showed a series of prominent peaks at e.g., m/z = 401.9 [1 ⊃ (4a)2–7Na+]7−, 472.7 [1 ⊃ (4a)2–6Na+]6−, and 571.7 [1 ⊃ (4a)2–5Na+]5− (Fig. S19†).
- Force-field calculations were carried out by the use of Materials Studio version 5.0 (Accelrys Software Inc., San Diego, CA).
-
(a) E. Arici, A. Greiner, H. Hou, A. Reuning and J. H. Wendorff, Macromol. Chem. Phys., 2000, 201, 2083–2090 CrossRef CAS;
(b) J.-A. Cheng, C.-P. Chang, C.-H. Chen and M.-S. Lin, J. Polym. Res., 2005, 12, 53–59 CrossRef CAS;
(c) W. Lin, L. Yuan and J. Feng, Eur. J. Org. Chem., 2008, 3821–3825 CrossRef CAS.
- The apparent FRET
efficiencies of 1 ⊃ (4a)2 and 1 ⊃ (4b)2 were estimated to be ∼99% from the fluorescence-quenching profile of the anthracene moieties (Fig. S23 and S29†).
Footnote |
† Electronic supplementary information (ESI) available: Experimental procedures and physical data. See DOI: 10.1039/c4sc02377c |
|
This journal is © The Royal Society of Chemistry 2015 |