DOI:
10.1039/C4RA11318G
(Paper)
RSC Adv., 2014,
4, 54936-54947
A commercial approach for the fabrication of bulk and nano phosphors converted into highly efficient white LEDs†
Received
3rd September 2014
, Accepted 3rd October 2014
First published on 6th October 2014
Abstract
Herein, we report a strategy to synthesize a highly efficient yellow light emitting Y3−xAl5O12:Cex (x = 0.03 to 0.3) based bulk as well as nano (rod-shaped) phosphors, which are the main component of solid state white light-emitting diodes (WLEDs). The as-synthesized phosphors were well characterized by several experimental techniques related to material characterization and spectroscopy. The bulk and nano phosphors emit with maximum photoluminescence intensities at 549 and 530 nm, respectively, upon excitation at a wavelength of 468 nm. These phosphors exhibit higher photoluminescence intensity as compared to commercially available bulk phosphors coated on WLED strips. Moreover, the integration of commercially available InGaN blue LED strips with the synthesized bulk and nano phosphors demonstrates better CIE coordinates and lower colour temperature with high brightness (>81% quantum yield) compared to commercially available WLED-based strips, lanterns and torches. These highly efficient light-emitting phosphors are a feasible candidate for potential use in commercial WLED applications.
1. Introduction
Solid state lighting (SSL) devices have become a paragon source for generating light due to their rapidly improving efficiency and huge energy savings compared to conventional high energy discharge lamps (e.g., metal halides and high pressure sodium); therefore, SSL devices require less energy input to deliver the same amount of output power.1–3 Over the past decades, a new generation of SSL in the form of white light-emitting diodes (WLEDs) have attracted considerable attention in display and lighting because of their high efficiency, compactness, good material stability, long operational lifetime (>100
000 h) and eco-friendliness, which gives them a leading edge over other lighting and display applications.1–7 Nowadays, the most general approach to generate white light from LEDs is the combination of blue LEDs (emission at ∼460 nm) or near-ultraviolet (N-UV) LEDs (∼370 − 410 nm) with complementary colour-emitting phosphors.8 Commercially, these are fabricated by combining blue LED chips with yellow-emitting phosphors. The operating mechanism of WLEDs depends upon the optical pumping of the integrated luminophors through emission from coloured LEDs. The subsequent collection of photoluminescence from phosphors preceded by the electro-luminescence of LEDs determines the properties of the device.9 To fully exploit the potential of WLEDs, there are still numerous drawbacks that have to be overcome simultaneously to make them feasible in real-life lighting applications. Among them, the low colour rendering index (Ra < 80) due to deficiency of red colour in emission and the different degradation rate of blue LEDs and phosphors, causing chromatic aberration and poor colour stability, are the most critical.10 Moreover, WLEDs emit cool white light with Commission International de I'Eclairage (CIE) coordinates of (0.292, 0.325) and a correlated colour temperature (CCT) of 7756 K, aggravating the situation and restricting their use in versatile applications.11
Ever since, WLEDs are based on the combination of yellow phosphors with blue LEDs. Numerous phosphors including NaSr4(BO3)3:Ce3+,Mn2+, NaBa4(BO3)3:Ce3+,Mn2+, Sr3B2O6:Ce3+,Eu2+, LiSrBO3:Eu2+ and Sr3(Al2O5)Cl2:Eu2+ have been reported for application in WLEDs. Most of them have claimed of their ability to replace Y3Al5O12:Ce (YAG:Ce) in commercial applications, but none of them have been successful so far.11–19 To date, the combination of the yellow phosphor YAG:Ce and InGaN-based blue LEDs is still the most popular method to generate white light.20 The strength of YAG:Ce lies in its unique features such as its strong absorption of blue light in the range of 440 to 480 nm, which results from dipole-allowed electronic transitions in activated Ce3+ ions, its subsequent broad emission in the yellow region, fast luminescence decay time (<100 ns), high external quantum efficiency (∼75% under blue LED excitation) and remarkably high chemical and thermal stabilities.21 However, there are still some critical problems associated with commercially used YAG:Ce in WLEDs, such as harsh synthesis conditions, the temperature quenching of luminescence and cool light (8500 K).22 Recently, many researchers have focused on the development of YAG:Ce nanophosphors to tackle the problems associated with bulk YAG:Ce.23,24 In order to comprehend these reports, we have carried out a comparative study between bulk and nano phosphors in this manuscript. The goal of the present investigation is to address all the above issues by tailoring and customising the synthetic method for both bulk and nano YAG:Ce phosphors. All the recently published reports in the literature focus only on the feasibility of either bulk or nano phosphors, not on both simultaneously.23–26 To the best of our knowledge, no one has compared the performances of WLEDs fabricated with bulk and nano phosphors (especially nanorods) of YAG:Ce; in our present work, the combined comparative study of these devices using the commercially available products has been accomplished. Due to their unique structural properties, nanorods are very efficient in extracting light. For the efficient operation of WLEDs, a larger effective surface area of the phosphor with good brightness and a minimum lattice distortion are desirable; nanorods possess both these features. In addition, they have very high aspect ratios, which eases the extraction of generated photons. Since we utilise a hydrothermal method for the preparation of nanorods, the temperature required for their synthesis is also reduced due to higher effective surface area. This leads to a more efficient synthesis method requiring less power consumption, which is further supported by the reduced initial growth temperature for phosphor nucleation along with a shorter annealing time. As the nano form of the phosphor is lightweight, the gravimetric mass of phosphor required for WLED fabrication is also less. Despite these great advantages, the use of nanorod-based phosphors for practical applications like the fabrication of WLEDs is severely restricted because of their lower luminous efficiency and photobleaching compared to bulk phosphors.27
Concisely, in this paper, we have synthesised bulk as well as nano Y3−xAl5O12:Cex (x = 0.03–0.3) phosphors and integrated them successfully with blue LED commercial strips. The shape-dependent structural/microstructural characteristics and photoluminescence properties of the synthesized phosphors have also been explored in detail. Furthermore, comparative studies between WLEDs fabricated with bulk and nano YAG:Ce phosphors have been discussed in terms of their commercial use, which is scarcely reported in the literature. In addition, we have also compared in-house fabricated WLED strips with commercially available Chinese WLED torches (Small Sun) and locally fabricated lanterns (Ashirwad, India) on the market. The obtained results strongly suggest that our synthesised phosphors are a promising candidate to replace existing phosphors utilised in commercial LEDs.
2. Experimental procedures
We have performed several experiments to synthesise bulk as well as nano YAG: Ce phosphors using techniques such as solid state reaction, co-precipitation (CP), sol–gel (SG), auto-combustion and hydrothermal methods. However, in terms of photoluminescent intensity, we obtained better results in the present investigation using auto-combustion with solid state diffusion (for bulk) and hydrothermal reaction (for nano) compared to the other methods. Thus, we have chosen to discuss our best obtained synthesis methods and the related results for the synthesis of bulk and nano phosphors.
2.1 Preparation of bulk YAG:Ce
Bulk Y3−xAl5O12:Cex (x = 0.03–0.3) was prepared by an integrated auto-combustion and solid state diffusion method. The starting materials including Y2O3 (99.99%), Al2O3 (99.99%) and Ce2O3 (99.99%) were used without any further purification unless otherwise stated. All the chemicals were purchased from Sigma-Aldrich. A stoichiometric amount of Y2O3, Al2O3 and Ce2O3 were separately mixed with a minimum quantity of DI water to form a milky white paste. As it is well known that oxides are insoluble in DI water, we further added a few drops of dilute HNO3 (69% GR) separately to each of the mixtures to produce a transparent solution and form the nitrates of the respective compounds. Subsequently, the mixtures were heated at 100 °C for 2 h under constant stirring. Urea (99.99%) was added to the mixture of prepared metal nitrates in a 20
:
1 volumetric ratio. The concentration of cerium in Y3−xAl5O12:Cex is varied from x = 0.03 to 0.3; x = 0.15 was found to be optimum value for the synthesis of high quality phosphors with high brightness. A typical doping concentration of Ce (x value) was 0.15 (5 mol%).
The prepared solution was heated on an electric coil heater (∼400 °C). The obtained yellow-coloured bulk mass was re-fired at 1400 °C for 4 h in the presence of boric acid as flux under ambient conditions in order to obtain the uniform diffusion of Ce in the YAG host. The yield of the product obtained was 88%. The procedure is highly reproducible and can be scaled up to large quantities. Details about the optimisation of Ce concentration and synthetic temperature are well discussed in the photoluminescence section. The overall reaction can be summarised as follows:
(3−x)Y(NO3)3 + xCe(NO3)3 + 5Al(NO3)3·9H2O + 20NH2·CONH2 → Y3−xAl5O12:Cex + 32N2 + 49H2O + 20CO2 |
For bulk YAG:Ce, we used an organic PVA (polyvinyl alcohol) cage to restrict the agglomeration of particles, which is well established in our previous studies on other phosphor materials.28 This encapsulation was eliminated at high temperature in the form of CO2 and H2O. For nanophosphors, we used cetyl trimethyl ammonium bromide (CTAB) as a surfactant, which is also removed from the synthesised phosphor when sintered at high temperature. However, for clarity, we have not incorporated the formulae of these encapsulants in our main equation.
2.2 Preparation of nano YAG:Ce
Nanorods of Y3−xAl5O12:Cex (x = 0.15) were synthesised by a hydrothermal method. The starting materials including Y2O3(99.99%), Al(NO3)3·9H2O (99.99%) and Ce(NO3)3 (99.99%) were used without further purification unless otherwise stated. All chemicals were purchased from Sigma-Aldrich. A stoichiometric amount of Y2O3, Al(NO3)3·9H2O and Ce(NO3)3 were used. The metal nitrates were dissolved in DI water to form a transparent solution. As oxides are insoluble in DI water, we further added dilute HNO3 (69% GR) into the Y2O3 paste prepared in DI water to form nitrates of the respective compounds. This step was followed by heating at 100 °C for 2 h under constant stirring until the solution became completely transparent. The doping concentration of Ce was kept the same as in the case of bulk Y3−xAl5O12:Cex (x = 0.15) to compare their responses at the same concentrations. CTAB (1 M) was added to the mixture of metal nitrate solutions and stirred for 1 h, resulting in a transparent metal nitrate solution. Subsequently, NaOH was added dropwise to the transparent metal nitrate solution without any sediments while stirring vigorously. The pH at the end of this reaction was maintained at ∼12. A yellow precipitate formed and floated on the solution; due to continuous stirring, the yellow precipitate became deformed and mixed homogenously with the solution to form a semi-dry gel that avoided any kind of sedimentation in the solution. The final solution obtained was kept in a hydrothermal bomb at 185 °C for 10 h. The lightweight and fine yellowish-white powder obtained after hydrothermal reaction was washed, dried and re-fired at 1400 °C for 1 h in the presence of boric acid as flux under ambient conditions. The yield of the product obtained in this case was 70%, and the synthesis can be scaled up to large quantities for commercial purposes.
The synthesis protocols of the bulk and nano phosphors are summarised in Scheme S1 see ESI.†
2.3 Fabrication of WLED strips
A solvent exchange process was developed to disperse the bulk and nanorod phosphors of YAG:Ce in an epoxy resin (structural formula shown in Fig. S1; see ESI†) with appropriate ratios. Epoxy resin is preferred because of its high refractive index (1.5), which reduces the total internal reflection.29 Initially, we dispersed the YAG:Ce phosphors (approximately 500 mg of bulk YAG:Ce and 10 mg of YAG:Ce synthesized powders) in epoxy solutions composed of mixtures of epoxy resin and curing agent (triethylene tetra amine, chemical structural formula shown in Fig. S1; see ESI†), which acts as a hardener. A micro syringe was used for dropping the epoxy solution on the LED chip The nozzle of the syringe used in our experiments had a diameter of 0.5 mm and a drop volume of ∼0.065 mm3. The amount of epoxy solution used was the same for both bulk and nano phosphors. The ratio of epoxy resin to hardener was 100
:
12.5 by volume. The above mixture was uniformly mixed to make a homogeneous mixture. Subsequently, it was carefully dispensed in the empty space available on the commercially purchased blue LEDs (LED 60 strip, DC 12 V, SMD Model 3528; non-waterproof blue InGaN-based ROHS, ISO 9001 registered company, made in China) to fill it properly, as shown in Scheme S2.† It was then left without further treatment to dry at room temperature for a few hours before testing. The fabrication protocol of WLEDs involving the integration of the synthesized bulk and nano phosphors with blue LED strips is shown in Scheme S2 (see ESI†).
2.4 Characterisation of bulk and nano phosphors
The as-synthesized bulk and nano phosphors were thoroughly characterised using a number of different techniques. Phase purity identification as well as gross structural characterisation of the YAG:Ce (bulk and nano) phosphors was performed with X-ray diffraction (XRD; Rigaku:MiniFlex, CuKα; λ = 1.5404 Å), which utilises the principle of Bragg Brantano geometry. The X-ray scan ranged from 20 to 75° at a scanning rate of 2° per minute. Prior to measurement, the diffractometer was calibrated with silicon powder (d111 = 3.1353 Å). Accurate lattice parameters were obtained by a least squares fitting method using computer-based unit cell refinement software.30 Scanning electron microscopy (SEM) was performed using a Carl Zeiss EVO MA-10 instrument. Microstructural characterisations were carried out by transmission electron microscopy (TEM) and high-resolution TEM (HRTEM; Technai, Model no. G20-twin, 200 kV with super twin lenses having point and line resolutions of 0.144 nm and 0.232 nm, respectively) equipped with energy dispersive X-ray analysis (EDAX) equipment for elemental studies. We determined the chemical composition and presence of elements using XPS spectroscopy. The photoluminescence (PL) characterisations of the bulk and nano phosphors were carried out with photoluminescence spectrometer (Edinburgh, FLSP-920) using a Xenon flash lamp as the excitation source. Time-resolved spectroscopy was performed using a photoluminescence spectrometer with an EPL 375 nm picosecond pulsed diode laser as the excitation source. The PL mapping of the bulk and nano phosphor-coated blue LED strips were performed using a WITech alpha 300R + confocal PL microscope system (WITec GmbH, Ulm, Germany) with a 375 nm diode laser as the excitation source. To estimate the absolute luminescence quantum efficiency of our synthesised phosphors, we used an integrating sphere equipped with an Edinburgh spectrometer (model F900) to measure the integrated fraction of luminous flux and radiant flux by the standard method. The colour temperature, CIE colour coordinates and luminance of the bulk and nano phosphor-based WLED strips were compared with the commercially available WLEDs using a colourimeter (C1210, serial no. 1296104; Fig. S2†) and a luminance meter (series L1000; Fig. S3, see ESI†). The instrumental facilities used are a part of the optical standard unit where national standardisation measurement is conducted at the National Physical Laboratory, New Delhi.
3. Results and discussion
In the typical synthesis procedure of Y3−xAl5O12:Cex (x = 0.03–0.3) phosphors, a customised SSR and hydrothermal method were used for the bulk and nano phosphors, respectively. The main purpose of the modification was to minimise the agglomeration of bulk and nano particles. We achieved non-agglomerated phosphors, which is an essential requirement for display applications. The versatility of this method easily allows the synthesis of large quantities of homogeneous rare earth-doped bulk and nano phosphors for commercial applications.
3.1 Gross structural characterisation
The gross structural analyses of samples were done by powder XRD. Fig. 1(a) and (b) show the XRD patterns of bulk and nanorod Y2.85Al5O12Ce0.153+ (YAG:Ce) powders, respectively. The XRD analysis is performed to examine the phase purity of the bulk and nano phosphors. The obtained experimental XRD patterns of the bulk and nano phosphors were well indexed with the Joint Committee on Powder Diffraction Standards (JCPDS) Card no. 33-0040. No additional impurities or secondary phases were observed in the XRD patterns. The crystal structural of YAG:Ce was cubic with lattice parameters of a = b = c = (12.0218 ± 0.0017) Å (bulk) and a = b = c = (11.9634 ± 0.0076) Å (nano), to the Ia
d space group. The lattice parameters of YAG:Ce were calculated from the observed ‘d’ values by a least squares fitting method using unit cell refinement software.30 The diffraction peaks of nano YAG:Ce were clearly broadened (Fig. 1(b)), which was expected according to the Scherrer equation: |
 | (1) |
where β is line broadening at full width half maximum intensity (FWHM) after subtracting the instrumental line broadening in radians, τ is the mean size of the crystallites, K is a dimensionless shape factor with a value close to unity (∼0.9), λ is the X-ray wavelength, and θ is the Bragg angle. The left inset of Fig. 1(a) shows the proposed unit cell of the Y2.85Al5O12Ce0.153+ crystal. The right inset shows the colour of as-synthesized bulk phosphor under room light. The inset of Fig. 1(b) shows the colour of the as-synthesised nano phosphor under room light. The XRD and cell parameters of the other variants of bulk Y3−xAl5O12:Cex (x = 0.03 to 0.3) phosphors are shown in Fig. S4 and Table S1 (see ESI†). It can be noted that the cell parameters and cell volume increase as the concentration of Ce increases up to x = 0.15 and decrease thereafter. This phenomenon has been previously observed for other rare earth oxide systems in our earlier publication.30 We have also performed Raman as well as FTIR analyses of the bulk and nano phosphors, as shown in Fig. S5–S8 (see ESI†). The results provide clear evidence for the presence of YAG:Ce bulk and nano phosphors.31,32 We have also carried out elemental analysis of the as-synthesised bulk phosphor by XPS spectroscopy, clearly demonstrating the presence of Al, Y, O and Ce along with their valance states (Fig. S9, see ESI†).
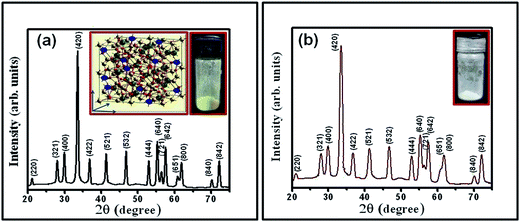 |
| Fig. 1 (a) XRD pattern of Y2.85Al5O12Ce3+0.15 bulk phosphor, indexed using JCPDS Card no. 33-0040. Left inset shows the proposed unit cell of Ce doped YAG crystal. Green, blue, whitish grey and red sites are occupied by yttrium, cerium, aluminium and oxygen, respectively. All together, the unit cell composed of eight molecular units of Y2.85Al5O12Ce3+0.15. Cerium atoms (blue colour) occupy yttrium sites substitutionally. Right inset shows as-synthesized bulk phosphor. (b) XRD pattern of Y2.85Al5O12Ce3+0.15 nano phosphor. The inset shows the as-synthesized nano phosphor. | |
3.2 Surface morphology and microstructural characterisations
Prior to SEM and TEM/HRTEM analyses, we sonicated the bulk and nano powder samples in ethanol using an ultra-sonicator operating at a frequency of 25 kHz to avoid any kind of agglomeration in the sample, consequently leading to better SEM and TEM/HRTEM image quality.
We employed SEM to explore the surface morphologies of the bulk and nano phosphors of Y2.85Al5O12Ce0.153+. Fig. 2(a) and (b) show SEM images of bulk and nano YAG:Ce phosphors, respectively. Fig. 2(c) and (d) show the magnified images of the areas marked by cross red circles in Fig. 2(a) and (b), respectively. Fig. 2(c) shows randomly distributed particles with average sizes of ∼0.5 μm (shown by arrow) for the bulk phosphor, while nano YAG:Ce exhibits rod-shaped structures with lengths of ∼1 μm and diameters of 20 nm (Fig. 2(d), shown by arrow). It is well-known from the concepts of carbon nanotubes from which nanorods or nanotubes have evolved that a material having at least one dimension in the nano regime (up to 100 nm) is considered as a nanomaterial.33 The formation of nanorods is due to the higher pH (∼12) maintained during the hydrothermal reaction process, which leads to the conversion of yttrium hydroxide to yttrium hexa-hydroxide with columnar structure. The hexa-hydroxide nanorods act as seeds for the growth of oxide nanorods. To analyse the microstructures of the synthesised phosphors and examine their crystal qualities, we used TEM and HRTEM. Fig. 3(a) and (b) show TEM images of bulk and nano YAG:Ce, respectively. In the case of bulk YAG:Ce, we observe agglomerates of particles with an average size of ∼0.5 μm. In the case of nano YAG:Ce, we obtain nanorods with an average diameter of ∼20 nm, which is consistent with our SEM data. HRTEM images of the specified areas marked by red cross circles in Fig. 3(a) and (b) are shown in Fig. 3(c) and (d), respectively. The HRTEM images indicate that both the bulk and nano samples exhibit lattice fringes with an estimated interspacing of 2.68 Å, which corresponds to the (420) plane. It can be easily noted from Fig. 3(c) and (d) that both samples (bulk and nano phosphors) exhibit distinct and continuous lattice fringes without any distortion, confirming the high crystal quality of the synthesised phosphors.
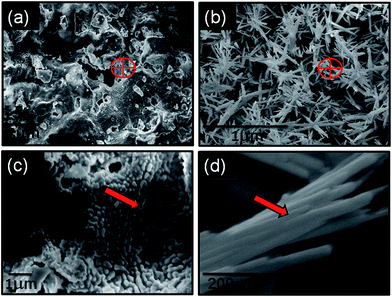 |
| Fig. 2 (a) SEM micrograph of bulk Y2.85Al5O12Ce3+0.15 phosphors. (b) SEM micrograph of nano Y2.85Al5O12Ce3+0.15 phosphors. (c) Magnified view of Y2.85Al5O12Ce3+0.15 bulk phosphors. (d) Magnified view of Y2.85Al5O12Ce3+0.15 nano phosphors. | |
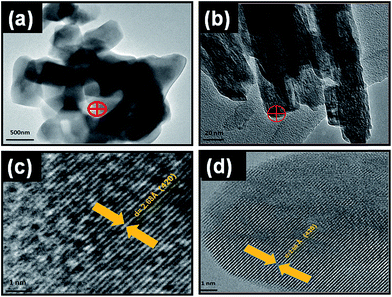 |
| Fig. 3 (a) TEM micrograph of bulk Y2.85Al5O12Ce3+0.15 phosphors (b) TEM micrograph of Y2.85Al5O12Ce3+0.15 nano phosphors (c) HRTEM image of Y2.85Al5O12:Ce3+0.15 bulk phosphors. (d) HRTEM image of Y2.85Al5O12:Ce3+0.15 nano phosphors. | |
3.3 Photoluminescence and time-resolved spectroscopy of YAG:Ce phosphors
PL characterisations of the YAG:Ce bulk and nano phosphors were performed using a luminescence spectrometer with a xenon lamp as the excitation source. The concentration of cerium in Y3−xAl5O12:Cex was varied from x = 0.03 to 0.3; x = 0.15 (Y2.85Al5O12Ce0.15) was found to be the optimum value for the synthesis of high quality phosphors with high brightness (see details in ESI Fig. S10†). Fig. S10† shows an initial steady increase in the PL intensity as the Ce concentration is increased up to x = 0.15; however, beyond this optimum value, the PL intensity began to decrease rapidly. This may be due to luminescence quenching as a result of excess Ce3+ ions. It is well known that the Ce doping concentration can affect the distances between Ce ions in the host lattice. When the Ce concentration is ≤5 mol%, the distance between two Ce ions is large, and every Ce ion can be regarded as an isolated luminescent centre that independently emits light without any interference. On the other hand, for doping concentrations beyond 5 mol%, nearby Ce ions can mutually interact by an electric multipolar process due to the shortened distances between Ce ions; in this case, the energy transfer rates of Ce ions easily exceed the radiative rates. Thus, the absorbed photon energy rapidly migrates among Ce ions in the host lattice, decreasing the probability of radiative transitions of Ce ions, and even quenching the fluorescence if the excited state gets trapped in an energy sink with a high non-radiative deactivation rate constant. This phenomenon is also known as concentration quenching of fluorescence.30 Therefore, it is very crucial to choose an appropriate doping concentration to obtain highly efficient photoluminescence when designing lanthanide-doped phosphors.34
Once the Ce concentration was optimised, we carried out further experiments to optimise the growth temperature for perfect phase formation. Growth time and growth temperature are important parameters that can improve the overall crystallinity of the bulk phosphor without significantly increasing the size of the nanophosphor, to further improve the PL intensity. Fig. S11† shows the dependence of the relative PL peak intensity (emission at 549 nm) on growth temperature as well as growth time. The relative peak intensity and brightness increase as the growth temperature is increased from 900 to 1400 °C. However, the PL intensities of the phosphors synthesized at temperatures above 1400 °C decrease sharply compared to that of the bulk phosphor synthesised at 1400 °C (optimum sample). This could be due to the creation of secondary oxide phases of yttrium and cerium at high temperatures or the improper diffusion and placement of Ce3+ ions at unfavourable yttrium sites.30 The XRD pattern of the optimum sample (5 mol%) at high temperature (1500 °C) indicates secondary phases of YAP (YAlO3 having a perovskite structure) and YAM (Y4Al2O9 having a monoclinic structure), as shown in Fig. S12.† The other cause may be that the number of radiative recombinations gradually decreases compared to non-radiative recombinations at temperatures above the optimum temperature and that material defects form quenching centres, leading to non-radiative recombination and luminescence quenching.30 Therefore, the change in emission intensity should be mainly associated with the defects that come from the surface states of phosphors after the recombination of electron–hole pairs. The crystallisation of the phosphor improved with increasing growth temperature up to the optimum temperature (1400 °C), and defects decreased accordingly as a consequence. Further increases in growth temperature lead to increased defects.
Fig. 4(a) shows the normalised excitation and emission spectra of bulk Y2.85Al5O12:Ce0.153+ phosphor with an emission wavelength of 549 nm, which corresponds to a 468 nm excitation wavelength. The inset of Fig. 4(a) shows the body colour of the as-synthesized bulk phosphor under ordinary light. Fig. 4(b) shows the normalised excitation and emission spectra of nano Y2.85Al5O12:Ce0.153+ phosphor with an emission wavelength of 530 nm, corresponding to a 468 nm excitation wavelength. The inset of Fig. 4(b) shows the body colour of the as-synthesised nano phosphor under ordinary light. Here, the emission of nanophosphors exhibits a blue shift compared to that of the bulk phosphor. This blue shift in the PL spectrum of nanophosphors can be understood as follows. Many studies have reported that the emission and absorption peaks of YAG:Ce phosphors vary with the synthesis method, size of phosphor particles, doping concentration and other factors.35 This occurs due to the extremely high sensitivity of Ce ion transitions (4f–5d) to the host environment.35 In addition, it is a well-established fact that materials entering into the nano regime exhibit size dependent blue shifts in their emission spectra; for example, ZnO quantum dots or Ag nanoparticles show blue shifts in their emissions depending upon their particle sizes. This phenomenon appears due to change in the band-gap of the materials occurring due to quantisation of energy levels in nano materials as compared to energy bands of bulk materials. In the present case, Ce ion-doped nanostructures also exhibit the phenomenon explained above. In spite of the blue shifting, both emission spectra are located well within the yellow region (520 nm–580 nm), which is desirable for the generation of white light as it is complementary to the blue light emitted by blue LEDs. Fig. 4(c) shows the chromaticity diagram of the CIE colour coordinates for bulk phosphor, which are x = 0.4753 and y = 0.4451. Fig. 4(d) shows the CIE colour coordinates for nano phosphor, which are x = 0.4613 and y = 0.4321.
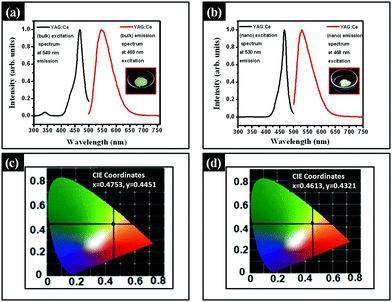 |
| Fig. 4 (a) Normalized excitation and emission spectra of Y2.85Al5O12:Ce0.153+ bulk phosphor. The inset exhibits yellow body colour of as-synthesized bulk phosphor under ordinary light. (b) Normalized excitation and emission spectra of Y2.85Al5O12Ce3+0.15 nano phosphors and its inset shows white colour powder of as-synthesized nano phosphor under ordinary light. (c) CIE coordinates of Y2.85Al5O12Ce3+0.15 bulk phosphors. (d) CIE coordinates of Y2.85Al5O12Ce3+0.15 nano phosphors. | |
The obtained excitation spectra of the bulk YAG:Ce phosphor exhibit two peaks at wavelengths of 468 nm and 341 nm. The existence of these two excitation peaks can be candidly explained by means of the energy level diagram of the YAG:Ce phosphor with Ce3+ as its activator (Fig. 5). As we know, Ce has an electronic configuration of [Xe] 4f26s2, and trivalent Ce3+ is described by a single electron system with an electronic configuration of [Xe] 4f1. Due to the hyperfine splitting that occurs as a consequence of electronic spin and nuclear spin coupling, the ground state of Ce3+ (4f) splits into two energy levels corresponding to the 2f 7/2 and 2f5/2 states, which have an energy difference of about 2200 cm−1 (0.28 eV).36 The next higher state corresponds to the 5d state, which splits into many levels under the influence of the crystal field. The observed excitation spectrum is a consequence of the 4f–5d dipole allowed transitions. These transitions are extremely sensitive to the local environment of the Ce3+ ion, which is basically a substitutional defect.36 As a result, more than one Ce3+ absorption band is observed in the excitation spectrum; however, there are two prominent bands corresponding to wavelengths of 341 nm and 468 nm. The excitation peak occurring between 400 to 500 nm is the most intense and desirable because this is the most essential requirement of a yellow phosphor in order for it to absorb the emission from InGaN-based blue LEDs, which lies in the region of 400 to 480 nm. The excitation spectra of YAG:Ce phosphors significantly overlap with the emission spectra of blue LEDs, making them functional for the fabrication of WLEDs.
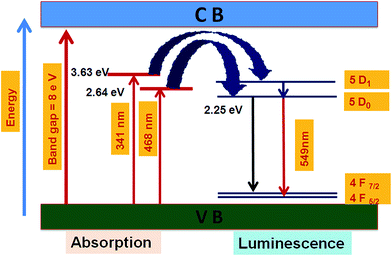 |
| Fig. 5 Proposed energy level diagram of Y3Al5O12:Ce3+. | |
Fig. 6(a) and (c) show the time-resolved photoluminescence spectra of the bulk and nano YAG:Ce phosphors, respectively. Time-resolved PL (TRPL) is a non-destructive and powerful technique generally used to determine the excitation lifetimes of phosphors, which indicates the quality of the phosphors. Excitation lifetime is a size-dependent parameter; smaller sizes correspond to shorter excitation lifetimes and higher recombination rates.37 For both bulk and nano YAG:Ce, the decay time is in the range of nanoseconds. However, bulk YAG:Ce has larger decay time compared to nano YAG:Ce. The difference in the decay times of bulk and nano phosphors can be explained by the localisation of Ce3+ ions near the surface. In nanorods, the surface to volume ratio is dramatically increased, and a large number of Ce3+ ions thus remain localised near the nanorod surfaces compared to the bulk phosphor. These Ce3+ ions act as highly reactive sites due to their higher surface energies. Thus, there will be a very high probability of Ce3+ ions oxidizing into the Ce4+ state, reducing the absorption and optical efficiency of the nano phosphors. In addition, there are large numbers of trap/defect sites available on the nanorod surfaces, enhancing non-radiative recombination. As the luminescence decay time (τ) depends on both radiative and non-radiative recombinations according to formula 1/τ = 1/τ radiative + 1/τ non-radiative, higher non-radiative recombination rates result in faster decay times. Fig. 6(a) and (c) show the time-resolved photoluminescence spectra of bulk and nano Y2.85Al5O12:Ce3+0.15 phosphors, respectively. Fig. 6(b) and (d) show the fitting curves and corresponding parameters for bulk and nano phosphors, respectively. The PL decay was recorded for the Ce3+ transitions at emission wavelengths of 549 nm and 530 nm, which correspond to a 375 nm excitation wavelength, for bulk and nanophosphors, respectively. The lifetime data of Ce3+ transitions (549 nm for bulk and 530 nm for nanorod) in Y2.85Al5O12:Ce0.153+ were fit well by the following double-exponential function:
|
I(t) = A1 exp(−t/τ1) + A2 exp(−t/τ2)
| (2) |
where
τ1 and
τ2 are the luminescence decay lifetimes, and
A1 and
A2 are the weighting parameters. The parameters generated from the fittings are shown in
Fig. 6(b) and (d). The observed lifetimes for bulk phosphor are
τ1 ∼ 8.26 ns and
τ2 ∼ 53.26 ns. In the case of nanophosphors, the observed lifetimes are
τ1 ∼ 4.56 ns and
τ2 ∼ 12.39 ns. These results indicate second order exponential decay, which is consistent with earlier published papers on YAG:Ce phosphors.
38 However, the values of the decay components depend upon the quality, composition, and intrinsic and extrinsic parameters of the material. For double-exponential decay, the average lifetime,
τaν, is determined by the following equation:
39–42 |
τaν = (A1τ12 + A2τ22)/(A1τ1 + A2τ2)
| (3) |
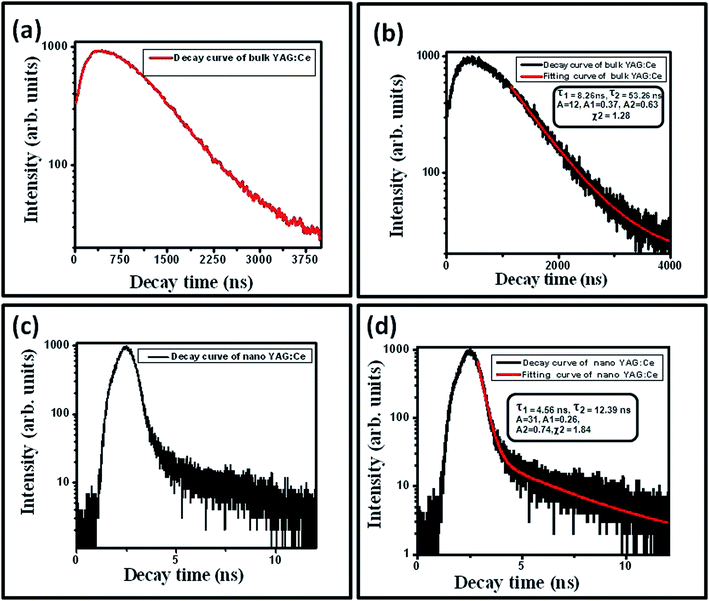 |
| Fig. 6 (a) Time-resolved photoluminescence spectrum of Y2.85Al5O12Ce3+0.15 bulk phosphors. (b) shows the fitting curve and corresponding parameters for bulk phosphor.(c) Time-resolved photoluminescence spectrum of Y2.85Al5O12Ce3+0.15 nano phosphors. (d) shows the fitting curve and generated parameters of nano phosphor. | |
The average lifetime for the bulk and nanophosphors are calculated to be τaν ∼ 48.897 ns and τaν ∼ 11.493 ns, respectively.
Fig. 7(a) compares the normalised PLE spectra of YAG:Ce bulk and nano phosphors excited at a wavelength of 468 nm. Interestingly, the bulk phosphor has a higher PL intensity than the nanophosphor at a growth temperature of 1400 °C and a cerium concentration of 5 mol%. The obtained results reveal that the bulk phosphor is more suitable for LED application as compared to the nanophosphor. However, the nanophosphor has its own advantages due to its lower gravimetric mass, which compensates for its lower intensity by requiring the incorporation of less material (50 times less than the bulk sample) for the fabrication of LEDs. We also performed photo-beaching experiments of bulk and nano phosphors. The obtained results reveal that both phosphors exhibit good photoluminescence stability, as shown in Fig. S13 and S14.† Keeping the commercial approach in mind, a comparative study has been performed among the synthesized bulk and nano phosphors and a commercial YAG:Ce bulk phosphor (synthesised using solid state reaction) obtained from uncoated white LED strips. Fig. 7(b) compares normalised PL spectra (fixed at an excitation wavelength of 468 nm) and PLE spectra (fixed at an emission wavelength of 549 nm) of bulk, nano and commercial YAG:Ce bulk phosphors coated on WLED commercial strips (LED 60 strip, DC 12 V, SMD Model 3528; non-waterproof blue InGaN-based ROHS, ISO 9001 registered company, fabricated in China). In order to maintain consistency, we compared the as-synthesized phosphor and in-house fabricated WLEDs with same company-based commercial YAG:Ce bulk phosphor coated on commercial WLED strips. The comparison of PL emission intensities suggests the feasibility of the synthesized phosphors (bulk and nano) for commercial use in white LED applications. Fig. 7(c) compares the CIE coordinates of bulk and nano phosphors. Fig. 7(d) compares the CIE coordinates of bulk, nano and commercially available YAG phosphors.
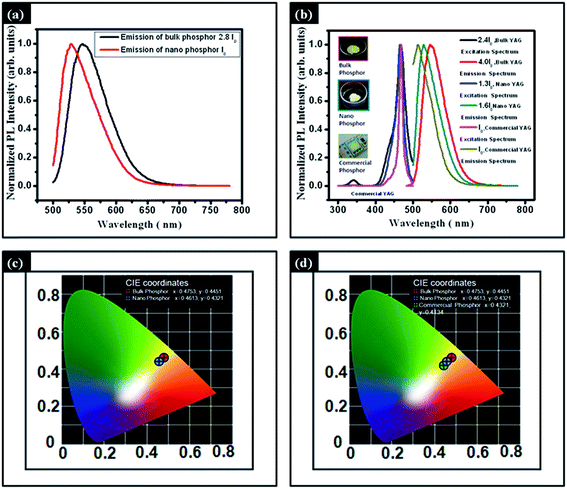 |
| Fig. 7 (a) Comparison of normalized PL emission spectra of YAG:Ce (bulk and nano) phosphors at 468 nm excitation wavelength. (b) Comparison of normalized PLE and PL spectra of bulk (as-synthesized), nano (as-synthesized) and commercial available YAG:Ce bulk phosphor. (c) Comparison of CIE coordinates of bulk and nano phosphor. (d) Comparison of CIE coordinates of bulk, nano and commercially available phosphor. | |
3.4 Electroluminescence studies of phosphor-converted WLED (pc-WLED) strips (in-house fabricated and commercially available)
In order to explore the electroluminescence (EL) properties of pc-WLEDs, in-house fabricated bulk and nano phosphor-converted white LED strips were compared with the commercially available white LED strips using a 12 V DC power supply. For the precise measurement of EL, we placed fabricated strips on a PL holder while covering all regions except a single LED by black paper (Fig. S15†). The exposure position of the LED is also optimised by employing the visible green emission at 532 nm during the experiment, as shown in Fig. S15.† As we know that the LED emission intensity is quite high, we placed a quartz diffuser in front of the strips throughout the experiment to prevent the saturation of the PMT detector.
The EL emission spectra of Fig. 8(a) and (b) exhibit the emission wavelength at 460 nm upon 12 volt DC supply. The EL emission spectra of blue LED significantly overlaps with excitation spectra of bulk and nano phosphors as shown in Fig. 4(a) and (b) which implies that this excitation wavelength is perfectly suitable for a white light generation device using blue strips. The insets of Fig. 8(a) and (b) show the uncoated blue strips as well as the bulk and nanophosphor-coated blue LED strips, respectively. Fig. 8(c) and (d) display the EL emission spectra of in-house fabricated bulk and nano phosphor-coated blue LEDs at a 12 V DC power supply, respectively. The EL emission spectra reveal the white light conversion from blue LEDs through the yellow phosphors. The right insets of Fig. 8(c) and (d) show the bulk and nano phosphor-coated blue strips emitting highly efficient white light, respectively. In order to examine the uniformity of the phosphor coatings on the blue LED strips, we performed PL mapping. The obtained results confirm the highly efficient photoluminescence intensity with uniform distribution throughout the surface, as shown in the left insets of Fig. 8(c) and (d) along with the scale bar.
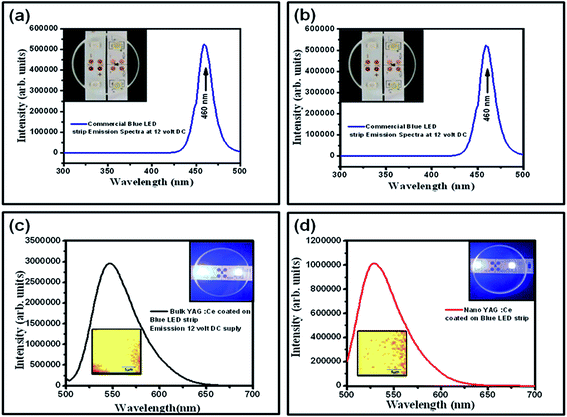 |
| Fig. 8 (a) and (b) The EL emission spectra of commercial blue LED strips without and with phosphor coatings, respectively, at 12 V DC voltage (glowing mode); the insets show the blue LEDs without and with bulk and nano phosphor-coated strips. (c) and (d) White light EL emission spectra of the in-house fabricated bulk and nano phosphor-coated blue LEDs, respectively, at a 12 V DC power supply. The right insets of (c) and (d) show the bulk and nano phosphor-coated blue strips emitting highly efficient white light. The left insets of (c) and (d) show the PL mapping images of bulk and nano phosphor-coated blue LEDs, respectively. | |
Finally, we comparatively study the white light emission from bulk and nano phosphors of YAG:Ce coated blue LED strips with the emission from commercial white LED strips (Fig. 9(a)). It is evident from these EL emission spectra that the in-house made phosphor shows encouraging results due to its high PL intensity as well as its better CIE coordinates compared to the commercial one, suggesting its promising potential for commercial applications. Fig. 9(b) compares the CIE coordinates of bulk and nano phosphor-coated WLEDs with commercial WLED strips. Fig. 9(c, i and ii) show the commercially available pristine blue LED without and with a 12 V DC power supply, respectively, with alphabet NPL. Fig. 9(c, iii and iv) portray the bulk yellow phosphor-coated blue LED without and with a 12 V DC power supply, respectively. The photographs elucidate the high efficiency of the WLED strips after phosphor integration. Furthermore, we also compared the performance of bulk and nano phosphors of YAG:Ce-coated blue LED strips with commercially available WLED strips, lanterns and torches to evaluate the commercialisation potential of our products (Table 1). The in-house fabricated devices show lower colour temperatures with better CIE coordinates and CRIs as compared to the commercially available products, indicating that these phosphors have good potential to ultimately replace commercially available phosphors.
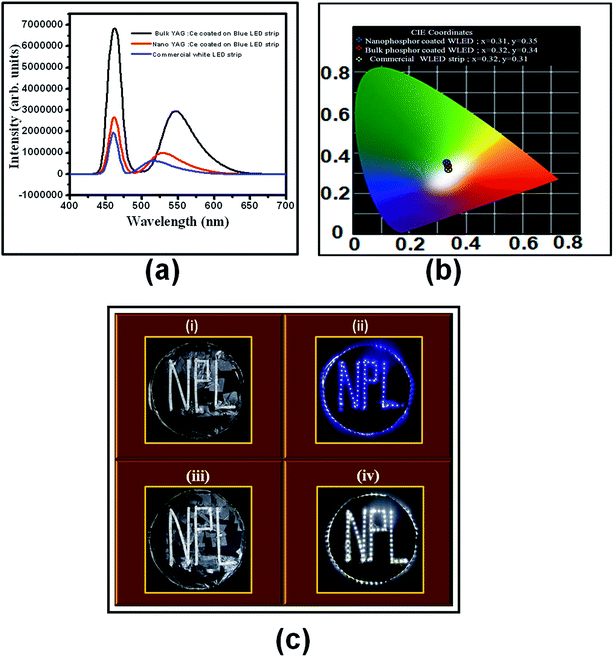 |
| Fig. 9 (a) Comparison of the EL spectra of commercial and in-house developed white LEDs to explore the feasibility of the as-synthesised bulk and nano phosphor for commercial use. (b) Comparison of the CIE coordinates of bulk and nano phosphor-coated WLEDs and commercial WLED strips. (c) (i and ii) Demonstrations of the commercially available blue LED strips without and with a 12 V DC power supply, respectively; (iii and iv) demonstrations of the bulk yellow phosphor coated on a blue LED strip without and with a 12 V DC power supply, respectively. | |
Table 1
4. Conclusions
In summary, we have successfully synthesised a bulk phosphor using an integrated auto-combustion with solid state diffusion method. Nano phosphors (rod shaped) have been synthesised via a hydrothermal route. The choices of host material (i.e., YAG) and activator (Ce) are adequately justified. The reaction conditions were simple and effective, with yields exceeding 88% for the bulk phosphor and 70% for the nanophosphor. The synthesis procedures can be easily scaled up to large quantities. Compared to the commercially available bulk phosphor, the synthesised phosphors exhibited higher photoluminescence intensities, with estimated quantum yields of more than 81% for the bulk phosphor and 72% for the nanophosphor. Furthermore, the commercially available InGaN blue LED strips integrated with the synthesised yellow bulk and nano phosphors demonstrate better CIE coordinates (bulk ∼0.32, 0.34; nano ∼0.31, 0.35), lower colour temperatures (CCT; bulk ∼6085 K, nano ∼6350 K), higher luminous efficiencies (η; bulk ∼76.13 lm W−1, nano ∼69.60 lm W−1), better colour rendering indices (Ra; bulk ∼88; nano ∼82), and high brightness as compared to commercially available WLED-based strips, lanterns and torches. The synthesis of bulk and nano phosphors for WLEDs in this work offers a new paradigm shift in the engineering of WLEDs for commercial applications. We envision that such accomplishments will be the key to developing high performance bulk and nano phosphor-based WLEDs to replace commercially available WLEDs.
Acknowledgements
The authors wish to thank Prof. R. C. Budhani, Director, N.P.L., New Delhi, for his keen interest in this work. The authors are thankful to Prof. O. N. Srivastava (Banaras Hindu University, Varanasi) for his encouragement. The authors also thank Dr V. P. S. Awana and K. N. Sood for recording XRD patterns and SEM micrographs, respectively. Jaya Dwivedi and Pawan Kumar thank CSIR and UGC for providing financial assistance.
References
- J. S. Kim, P. E. Jeon, J. C. Choi, H. L. Park, S. I. Mho and G. C. Kim, Appl. Phys. Lett., 2004, 84, 2931 CrossRef CAS PubMed.
- W. B. Im, Y. I. Kim, N. N. Fellows, H. Masui, G. A. Hirata, S. P. Den and B. R. Seshadri, Appl. Phys. Lett., 2008, 93, 091905 CrossRef PubMed.
- T. Nishida, T. Ban and N. Kobayashi, Appl. Phys. Lett., 2003, 82, 3817 CrossRef CAS PubMed.
- S. Nakamura and G. Fasol, The Blue Laser Diode, Springer, Berlin, 1997 Search PubMed.
- H. V. Demir, U. O. S. Seker, G. Zengin, E. Mutlugun, E. Sari, C. Tamerler and M. Sarikaya, ACS Nano, 2011, 5, 2735 CrossRef CAS PubMed.
- A. A. Setlur, W. J. Heward, Y. Gao, A. M. Srivastava, R. G. Chandran and M. V. Shankar, Chem. Mater., 2006, 18, 3314 CrossRef CAS.
- Y. D. Huh, J. H. Shim, Y. Kim and Y. R. Do, J. Electrochem. Soc., 2003, 150, 57 CrossRef PubMed.
- H. S. Jang, Y. H. Won and D. Y. Jeon, Appl. Phys. B: Lasers Opt., 2009, 95, 715 CrossRef CAS.
- X. M. Zhang, X. B. Qiao and H. J. Seo, Curr. Appl. Phys., 2011, 11, 442 CrossRef PubMed.
- X. M. Zhang and H. J. Seo, Phys. B, 2010, 405, 2436 CrossRef CAS PubMed.
- C. K. Chang and T. M. Chen, Appl. Phys. Lett., 2007, 91, 081902 CrossRef PubMed.
- Z. J. Wang, P. L. Li, Z. P. Yang, Q. L. Guo and X. C. Li, Phys. B, 2010, 19, 017801 Search PubMed.
- Y. S. Tang, S. F. Hu, W. C. Ke, C. C. Lin, N. C. Bagkar and R. S. Liu, Appl. Phys. Lett., 2008, 93, 131114 CrossRef PubMed.
- J. H. Yum, S. Y. Seo, S. Lee and Y. E. Sung, Proc. SPIE, 2001, 4445, 60 CrossRef CAS PubMed.
- Y. S. Lin, R. S. Liu and B. M. Cheng, J. Electrochem. Soc., 2005, 152, J41 CrossRef CAS PubMed.
- J. H. Yum, S. Y. Seo, S. Lee and Y. E. Sung, J. Electrochem. Soc., 2003, 150, H47 CrossRef CAS PubMed.
- S. Lee and S. Y. Seo, J. Electrochem. Soc., 2002, 149, J85 CrossRef CAS PubMed.
- P. Shlotter, J. Baur, C. H. Hielscher, M. Kunzer, H. Obloh, R. Schmidt and J. Schneider, Mater. Sci. Eng., B, 1999, 59, 390 CrossRef.
- J. Baur, P. Shlotter and J. Schneider, Festkorper Probleme, Adv. Solid State Phys., 1998, vol. 37, p. 67 Search PubMed.
- P. Shlotter, R. Schmidt and J. Schneider, Appl. Phys. A, 1997, 64, 417 CrossRef.
- J. K. Park, C. H. Kim, S. H. Park and H. D. Park, Appl. Phys. Lett., 2004, 84, 1647 CrossRef CAS PubMed.
- V. Bachmann, C. Ronda and A. Meijerink, Chem. Mater., 2009, 21, 2077 CrossRef CAS.
- T. Isobe, ECS J. Solid State Sci. Technol., 2013, 2, R3012 CrossRef CAS PubMed.
- H. J. Yang, H. R. Xu, G. S. Zhu, L. Yuan, C. Zhang, F. S. Li and A. B. Yu, Mater. Lett., 2013, 92, 161 CrossRef CAS PubMed.
- S. H. Lee, H. Y. Koo, Su M. Lee and Y. C. Kang, Ceram. Int., 2010, 36, 611 CrossRef CAS PubMed.
- H. Yang, D.-K. Lee and Y.-S. Kim, Mater. Chem. Phys., 2009, 114, 665 CrossRef CAS PubMed.
- A. Revaux, G. Dantelle, N. George, R. Seshadri, T. Gacoin and J. Boilot, Nanoscale, 2011, 3, 2015 RSC.
- B. K. Gupta, T. N. Narayanan, S. A. Vithayathil, Y. Lee, S. Koshy, A. L. Mohana Reddy, A. Saha, V. Shanker, V. N. Singh, B. A. Kaipparettu, A. A. Martí and P. M. Ajayan, Small, 2012, 8, 3028 CrossRef CAS PubMed.
- W.-F. Su, Y.-C. Fu and W.-P. Pan, Thermochim. Acta, 2002, 392–393, 385 CrossRef CAS.
- B. K. Gupta, D. Haranath, S. Saini, V. N. Singh and V. Shanker, Nanotechnology, 2010, 21, 055607 CrossRef PubMed.
- P. Lasch, A. Hermelink and D. Naumann, Analyst, 2009, 134, 1162 RSC.
- R. Asakura, T. Isobe, K. Kurokawa, H. Aizawa and M. Ohkubo, Anal. Bioanal. Chem., 2006, 386, 1641 CrossRef CAS PubMed.
- J. Tzeng Lue, Encyclopedia of Nanoscience and Nanotechnology, ed. H. S. Nalwa, 2007, vol. X, p. 1 Search PubMed.
- B. K. Gupta, V. Rathee, T. N. Narayanan, P. Thanikaivelan, A. Saha, Govind, S. P. Singh, V. Shanker, A. A. Marti and P. M. Ajayan, Small, 2011, 7, 1767 CrossRef CAS PubMed.
- D. Jia, Chem. Eng. Commun., 2007, 194(12), 1666 CrossRef CAS.
- Y. Pan, M. Wu and Q. Su, J. Phys. Chem. Solids, 2004, 65, 845 CrossRef CAS PubMed.
- B. K. Gupta, V. Shanker, M. Arora and D. Haranath, Appl. Phys. Lett., 2009, 95, 073115–31 CrossRef PubMed.
- J. D. Furman, G. Gundiah, K. Page, N. Pizarro and A. K. Cheetham, Chem. Phys. Lett., 2008, 465, 67 CrossRef CAS PubMed.
- Q. Lu, Y. Wu, L. Ding, G. Zu, A. Li, Y. Zhao and H. Cui, J. Alloys Compd., 2010, 496, 488 CrossRef PubMed.
- S. Murakami, M. Herren, D. Rau and M. Morita, Inorg. Chim. Acta, 2000, 1014, 300 Search PubMed.
- T. Fujii, K. Kodaira, O. Kawauchi and N. Tanaka, J. Phys. Chem. B, 1997, 101, 10631 CrossRef CAS.
- B. K. Gupta, P. Thanikaivelan, T. N. Narayanan, L. Song, W. Gao, T. Hayashi, A. L. M. Reddy, A. Saha, V. Shanker, M. Endo, A. A. Martí and P. M. Ajayan, Nano Lett., 2011, 11, 5227 CrossRef PubMed.
Footnote |
† Electronic supplementary information (ESI) available. See DOI: 10.1039/c4ra11318g |
|
This journal is © The Royal Society of Chemistry 2014 |