DOI:
10.1039/C4RA10399H
(Review Article)
RSC Adv., 2014,
4, 63741-63760
Nanomaterials-based electrochemical detection of chemical contaminants
Received
13th September 2014
, Accepted 18th November 2014
First published on 18th November 2014
Abstract
Owing to the high toxicity and detrimental effects of chemical contaminants to human health and the environment, public concerns over chemical contaminants in the environment and in foods have been mounting drastically. It is therefore significant to monitor contaminants via portable sensing devices, which encompass the demands of being low-cost and the potential for online environmental monitoring and food safety applications. This review will assess various concepts and recent advancements in design and the application of state-of-the-art nanomaterials through the incorporation of carbon nanomaterials, metallic and metallic oxide nanoparticles, titanium dioxide nanotubes, and dendrimers toward the development of electrochemical sensors for the detection of chemical contaminants in the environment and in foods. The development of nanomaterials based sensors facilitated by recent advances is having a major impact on sensor industries for environmental and food safety monitoring. Electrochemical sensing strategies have spurred intense interest in the research community as they have the capacity to serve as ideal sensor technology candidates, having such features as rapid response, robustness, high sensitivity and selectivity, low cost, miniaturization, and the potential for real-time monitoring. Nanomaterials have strong potential for increasing the competitiveness of new sensors for environmental monitoring and food safety applications through the combination of efficacious, yet simple fabrication techniques in the development of critical nanometric interfaces, and the optimization of their design and performance. Opportunities and future considerations for the use of nanomaterials in electrochemical sensors for producing advanced environmental and food sensing devices will also be addressed.
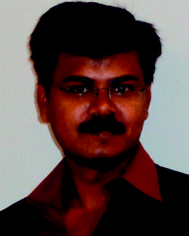 Maduraiveeran Govindhan | Maduraiveeran Govindhan received his PhD in Chemistry from Madurai Kamaraj University, Tamil Nadu, India. He is currently working as a Postdoctoral Researcher in Department of Chemistry at Lakehead University, Canada. His research interests involve nanostructured metal particle assemblies and thin films, the design of optical and electrochemical sensors, fuel cells, electrochemical metal dissolution and water splitting, potentiometric high-temperature NOx gas sensors and solid-state chemiresistive gas sensors. He has been conferred Senior Research Fellowship from the Council of Scientific and Industrial Research, India (2009) and Emerging Scientist Award from Lakehead University, Canada (2014). |
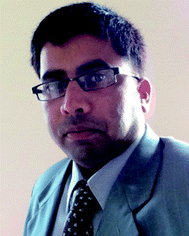 Bal-Ram Adhikari | Bal-Ram Adhikari is a PhD candidate in the Biotechnology programme at Lakehead University. He received his Master's degree in Microbiology from Tribhuvan University, Nepal. His research interests are in nanomaterials and nanochemistry for biological applications. His current research focuses primarily on the development of nanomaterials based sensors and biosensors for pharmaceutical, environmental and food safety applications. |
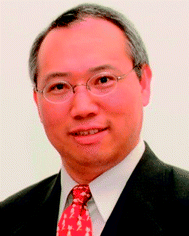 Aicheng Chen | Aicheng Chen is a Professor of Chemistry and Canada Research Chair in Materials and Environmental Chemistry at Lakehead University. He received his PhD in electrochemistry at the University of Guelph in 1998. His research interests span the areas of electrochemistry, biosensors, green chemistry, materials science and nanotechnology. Chen is a receiptant of the Ontario Premier's Research Excellence Award, the Japan Society for the Promotion of Science Invitation Fellowship, the Fred Beamish Award of the Canadian Society for Chemistry, the Lash Miller Award of The Electrochemical Society Canadian Section, the Keith Laidler Award of the Canadian Society for Chemistry, the Lakehead University Distinguished Researcher Award, and the Canadian Catalysis Lectureship Award. In addition, he was elected as a Fellow of the Royal Society of Chemistry (UK) in 2011, and a Fellow of International Society of Electrochemistry in 2014. |
1. Introduction
Enormous challenges currently confront environmental and food safety organizations that are responsible for the mitigation of risks associated with myriad complex mixtures of toxic chemical contaminants, which are constantly changing. Approximately 700 new chemicals are introduced into the marketplace annually in the US alone. Many of these substances are subsequently released into the ambient environment even though their toxicity and effects on human health and ecosystems are unknown, and where they are likely to be further combined with additional chemicals.1 An expanding group of chemical contaminants of concern encompasses those that emanate primarily from industry, human and animal fecal waste, natural toxins, drinking water disinfection byproducts, through the corrosion of household plumbing systems, from food additives, personal care products, discarded pharmaceuticals, and via food preparation and packaging processes.2 Chemical contaminants are of particular concern in that they may cumulatively cause severe damage to human health. Specifically, some chemicals are suspected of being cancer promoters, whereas other compounds have been associated with endocrine disrupting effects, or may be accumulated and biomagnified through the food chain.3 The development of analytical methods and techniques in the environmental and food sciences have been developed parallel with growing consumer concerns as relates to food safety and security.4 These research areas have been complicated by the increasing mobility and globalization of foodstuffs and associated raw materials, which are directly and indirectly exacerbating contamination events that are also growing on a global scale.5 In an effort to decrease the complexity of food safety, food laboratories have been forced to replace their classical analytical methods with 21st century techniques, which may facilitate the provision of adequate responses to this expanding global demand. In EU countries, new European regulations (Regulation EC 258/97 or EN 29000 and subsequent issues), the Nutrition Labeling and Education Act in the U.S. as well as the Montreal Protocol, have had a major impact on food laboratory operations.6 Dudarev et al.7 have recently reported problems related to food security in the Russian Arctic e.g., dietary imbalance, predominance of carbohydrates, shortage of dairy products, fruits and vegetables, chronic deficits of vitamins and microelements, and the presence of chemical, infectious agent, and parasitic food contaminants. Dudarev and his co-workers collected data from the Federal Automatic System “Social-Hygienic Monitoring”, which contained data on ∼178
000 analyzed food samples from all selected regions during 2007–2011. A chart related to the major chemical contaminants in foods is depicted in Fig. 1. Owing to the escalating demands on environmental and food monitoring, more sophisticated and appropriate instrumentation methods that have the capacity to provide improved qualitative and quantitative measurements are definitely warranted, as are increased sensitivity, precision, specificity, and/or speed of analyses.8
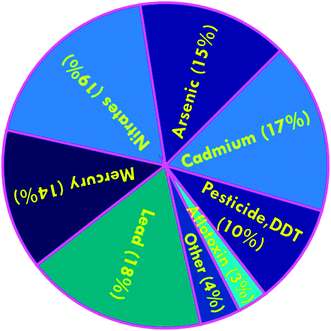 |
| Fig. 1 Chart of chemical contaminants in food samples from all selected regions, percentage of total number of samples analyzed. Reproduced with permission from ref. 7. Copyright© 2013 International Journal of Circumpolar Health. | |
A variety of analytical techniques are commonly employed in environmental and food analysis. Typically, they encompass nuclear magnetic resonance (NMR), infrared (IR), atomic spectroscopy (AS), fluorescence, high-performance liquid chromatography (HPLC), gas chromatography (GC), capillary electrophoresis (CE), supercritical fluid chromatography (SFC), solid phase extraction (SPE), supercritical fluid extraction (SFE), headspace (HS), and flow injection analysis (FIA), etc. Electrochemical sensors are emerging to be a technology of choice in this respect as they are self-contained, compact and low cost, with minimal power requirements. These integrated devices also offer high sensitivity, as they are capable of providing specific quantitative analytical information via the utilization of chemical/biological recognition elements, which can translate interfaced contaminant inputs into useable output signals.9 Electrochemical sensors are generally easy to fabricate, while enabling the detection of single and multiple pollutants simultaneously. The primary drawback of these sensors for environmental and food applications is that they are not currently commercially available. Environmental and food samples are typically sent to laboratories, resulting in high costs and difficulties associated with real time monitoring.10 The prerequisites of large sample analysis and the requirement for continuous monitoring in remote areas, make the development of these sensors even more challenging with respect to the establishment of first principles and specific applications.11,12 A critical need for advanced and portable electrochemical sensors to facilitate environmental and food safety and security has been heightened, and is anticipated to expand tremendously over the next few years. The further integration of materials science, micro-fabrication, functional nanomaterials, and electrical engineering with sensor technologies will be required to advance wireless, real time environmental and food monitoring and analysis. Although some detection limits have been reported in the literature to be at femto-molar, ppt, or even lower concentrations,13–15 in terms of ensuring the safety and security of food and the environment, an assessment on the detection levels that are suitable for these real-world applications will have to be made. The establishment of detection limits will be crucial in terms of risk assessment analyses. The desirable sensors should address the presence of specifical contaminants in environmental and food samples.
Over the last decade, nanomaterials have increasingly garnered attention in the disciplines of electrocatalysis and analytical chemistry, where they provide high surface area to volume ratios and can be tailored to encourage particular reaction pathways. Interestingly, morphologically dependent interatomic bond distances, melting points, chemical reactivity, as well as optical and electronic properties can have profound influences on the functionality of nanomaterials.16 Nanomaterials may enable specific functionalities that are selected to align with targeted applications, which are derived from the interactivity of constituent nanometric elements with their immediate environments. The electrocatalytic activity of nanomaterials can be analyzed in detail using electrochemical measurements.17 Nanomaterials make possible the potential development of novel devices with unique functionalities and processes that are activated on exposure to chemical contaminants, as well as the capacity for enhanced mobility and real time monitoring in environmental and food applications.18 The evolution of nanostructured materials based electrochemical sensor technologies constitutes a very active and robust research area that is expected to provide next generation technologies for environmental and food science analyses, toward improving and/or supplanting conventional sensing strategies. This review will endeavor to elucidate the use of nanomaterials toward the design of potential electrochemical sensors and biosensors for the detection of chemical contaminants, in facilitating efficacious environmental and food safety monitoring. One of its chief aims is to deliver an expansive overview and describe relevant advances in the field of nanomaterials based electrochemical sensing of chemical contaminants. Several core issues related to this rapidly emerging area must be taken into consideration for future research, and will be articulated in the last section.
2. Electrochemical methods in sensing
A variety of electrochemical methods have been explored for sensing chemical contaminants and biomolecules.19–21 Typically, voltammetric techniques such as cyclic voltammetry (CV) and linear sweep voltammetry (LSV), differential pulse voltammetry (DPV), square wave voltammetry (SWV) etc., amperometric and potentiometric techniques, and electrochemical impedance (EIS) techniques are utilized in the determination of analytes. For instance, electrochemical principles for the sensing of analytes are highly relevant in microfluidics and the broader field of separation science for the purposes of detection, injection, pumping, and valving. A number of microscopic techniques are coupled with electrochemical principles, such as scanning electrochemical microscopy (SECM) and chemically selective scanning tunneling microscopy (CSSTM), which are actually spatially resolved electrochemical sensors, even though they are classified as microscopic techniques. This operates via microdialysis probes that are coupled to an electrochemical detection system, which is an option following an on-line separation step.22 An electrochemical sensor consists of two major components: (i) a chemical or biological recognition element; and (ii) a physical transducer (electrode) that transduces the analytical signal of the sensing event to an electronic circuit. Interactions between the sensing element of the substrate and the analytes are determined via the sensitivity, selectivity, speed of response and reversibility of the designed sensors.23 Strong interactions are typically associated with higher sensitivity and selectivity, whereas perfect reversibility requires weak interactions. The critical parameters of electrochemical sensors are sensitivity, detection limit, dynamic range, selectivity, linearity, response time, and stability.24 Fig. 2 displays a schematic illustration of an electrochemical sensor system, which illustrates the three primary elements, the sample (or analyte), the transduction platform, and the signal-processing step.
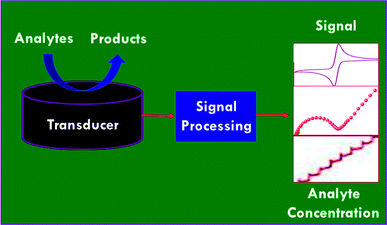 |
| Fig. 2 Principal stages involved in the operation of an electrochemical sensor. | |
Electrochemistry based analytical methods have impacted multiple fields, including diagnostics, environmental analysis, food sciences, enzymatic kinetics and pharmacology.25–27 In 2002, Wang reviewed miniaturizing electroanalytical systems and their incorporation into microfluidic devices for point of care applications, environmental analysis, and genetic testing.28 In 2003, Baeumner29 surveyed the application of electrochemical biosensors for environmental pollutants and food contaminants, which focused mainly on the detection of chemical and biological contaminants using electrochemical biosensors. Recently, the assembly of miniaturized electrochemical devices using graphene oxide (GO) with ionic liquid (IL) composites has been demonstrated for the electrochemical detection of several electroactive targets of importance in food analyses, environmental monitoring, and clinical diagnoses.30 Banks and co-workers31 reviewed the electrochemical applications and advancements of graphene (GR) based materials for sensor applications. The environmental and biological monitoring of organophosphorus (OP) pesticides and nerve agents using nanomaterials based biosensors have reviewed by Zhang et al.32 Cliffel and co-workers33 examined the advances made in electrochemical and biochemical sensor development and usage, which primarily covered the areas of reference electrodes, potentiometric sensors, voltammetric sensors, amperometric sensors, biosensors, immunosensors, and mass sensors. Our research group has recently surveyed the design and the development of electrochemical sensors based on nanomaterials for biomedical applications,34 the applications of SWV in electrochemical sensing,35 and the synthesis and application of Pt based nanomaterials.36 In the present review, we will focus on the development of electrochemical sensors and biosensors based on nanomaterials for the detection chemical contaminants and their promising applications in environmental monitoring and food safety.
3. Nanomaterials based electrochemical sensing platforms
The schematic depiction of a variety of nanomaterials employed for the detection of chemical contaminants is illustrated in Fig. 3. Carbon based nanomaterials include single-walled carbon nanotubes (SWNTs), multiwalled carbon nanotubes (MWNTs), single-walled carbon nanohorns (SWCNHs), fullerenes (e.g., C60), and graphene, among many others. Carbon has the capacity to hybridize into sp, sp2 and sp3 configurations, contingent on the bonding relationships with neighboring atoms, which have narrow gaps between their 2s and 2p electron shells. These associations are responsible for enabling the design of a wide assortment of nanoscale carbon-based materials that are very versatile, which exhibit distinct properties in terms of size, surface area, strength, photonics and electronics. Carbon nanomaterials demonstrate many interesting attributes that make them ideal for serving as electrode materials in electrochemical sensor applications.37–42 The presence of surface resident reactive groups, or edge-plane like sites that are situated at the ends of their structures, and at defect sites, are responsible for the excellent electrocatalytic activity of carbon nanomaterials. The advantages and limitations of carbon-based nanomaterials with the respect to analytical chemistry including sample preparation, separation and detection were reviewed by Scida et al. in 2011.43 The functionalization of carbon nanotubes has been highlighted by a feature article authored by Huang and co-workers.44 For instance, the chemically reduced GO modified GC electrode that functioned as the transducer for a potassium ion sensor with a PVC-based selective membrane, which showed superior performance in contrast to conductive polymer based sensors.45 A potentiometric sensor has been developed by Michalska and co-workers46 that employed functionalized graphene as a transducer and selective film for the detection of Zn2+ ions. In addition, the sensing metal ions was performed by the functionalization of a GO-based electrochemical sensor.47 MWCNT modified electrodes were developed for the detection of europium(III) via differential pulse adsorptive stripping voltammetry (DPASV), where a wide linear range with a lower detection limit was obtained.48 CNTs have been used as electrochemical sensing platforms for the detection of microbiological chemical toxins,49 pesticides50 and explosives.51 A tyrosinase-immobilized carbon-felt-based flow biosensor was explored on the development of a highly sensitive sensor for monophenolic compounds.52 SWCNH is another type of carbon-based nanomaterials, which has a conical structure with a base diameter of ∼5 nm and a tubule length of ∼50 nm. SWCNHs have been used for the detection of chemical contaminants such as chloramphenicol,53 triclosan and bisphenol A.54
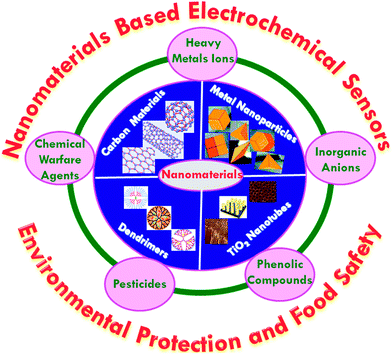 |
| Fig. 3 Schematic of the electrochemical detection of chemical contaminants with nanomaterials. | |
Metallic nanomaterials such as platinum, gold, silver and palladium with intriguing size dependent electrical, optical, magnetic, and chemical properties have been intensively studied for their fundamental scientific interest and toward many potential technological applications. Noble metal nanoparticles are under extensive study and have led to the development of numerous analytical techniques and methods for environmental monitoring and food safety applications.55–57 The inhibitory enzyme–nanoparticles based biosensors were developed for food safety and environmental monitoring applications.58 The inhibitory based biosensor can increase the sensor stability and render the regeneration of the enzyme easier and it may suffer from side reactions due to coexistence of several enzymes. The non-enzymatic electrochemical sensor for H2O2 was reported, which employed Au NPs that were embedded within a silicate sol–gel electrode.59 Monometallic and bimetallic nanoparticles, such as gold nanoparticles (Au NPs), platinum nanoparticles (Pt NPs), palladium nanoparticles (Pd NPs), and platinum–palladium nanoparticles (Pt–Pd NPs) have been widely utilized in the development of electrochemical sensors for toxic chemicals.60 Au NPs hosted by a water-soluble silsesquioxane polymer based electrochemical sensor was developed for the detection of nitrophenol isomers.61 Metal oxide based nanostructures, such as ZnO, Fe3O4, NiO, SnO2, ZrO4, TiO2, MgO and MnO2, have also been widely used in the detection of chemical contaminants due to their unique nanomorphological, functionally biocompatible, non-toxic and catalytic properties.62 Porous MgO nanoflowers have been synthesized for the fabrication of an electrochemical sensor for the detection of heavy metals. Radhakrishnan et al.63 described a highly sensitive electrochemical sensor for nitrite detection, which was based on Fe2O3 nanoparticles that were decorated with reduced graphene oxide nanosheets, showing a linear concentration range of 50 nM to 0.78 mM, with a detection limit of 15 nM. Jena et al.64 designed an electrochemical sensor that was based on ensembles of Au nanoelectrodes for the simultaneous electrochemical detection of ultra-trace concentrations of arsenic, mercury, and copper ions.
Titanium dioxide nanotubes (TiO2 NTs) have garnered significant research interest due to their ease of preparation, high orientation, extensive surface area, high uniformity and excellent stability. The electrochemical behavior of TiO2 is contingent on its crystalline structure, surface properties and textural properties, which include specific surface area, pore volume, pore dimensions and distribution.65 TiO2 NTs decorated with metal particles and enzymes have been employed for the development of electrochemical sensors and biosensors for the detection of a variety of species.66–70 Au@Pd core–shell nanoparticles supported on amino-functionalized TiO2 NTs were developed and tested as a potential electrochemical sensor for the detection of hydrazine.71 Ozturk and his co-workers72 reported rutile TiO2 NTs for the detection of volatile organic compounds.
Dendrimers are a new class of polymeric materials, which are monodisperse, three-dimensional (3D) hyperbranched, nanoscale polymeric architectures with an extremely high surface functional group density.73 The electrostatic interactions, complication reactions, various types of weaker forces, such as van der Waals, H-bonding, etc. are the prime drivers of guest encapsulation within dendrimers.74,75 In recent years, dendrimer-encapsulated metal NPs and dendrimer-cored metal NPs have attracted considerable interest due to their potential use in catalysis and sensors. Fang et al.76 investigated the electrochemical communication between Au NPs deposited onto adsorbed dendrimers of poly(amido amine) (PAMAM) and the gold or platinum electrode surface. Zheng and co-workers77 have demonstrated an electrochemical sensor for the detection of nitrite using PAMAM dendrimer-stabilized Ag NPs. Siriviriyanun et al.78 developed an electrochemical sensor approach that involved CNTs, Pt NPs, dendrimers, and enzymes that enabled effective and rapid detection of small volumes of analytes on potentially cost effective and disposable printed chips. The electrocatalytic activity of the prepared biosensors demonstrated the feasible detection of H2O2 at a low electrode potential, and the detection of an inhibitory biocontaminant (organophosphorus pesticide) over a wide range of concentrations. More details on the conducting polymer nanomaterials for sensing and biomedical applications may be found in the recent review articles.79,80
4. Applications in the detection of chemical contaminants
The development of electrochemical sensors is of great significance due to their capacity to resolve a potentially large number of analytical problems and challenges across very diverse areas, such as defense, homeland security, agriculture and food safety, environmental monitoring, medicine, pharmacology, industry, etc. A growing demographic of individuals with a high risk of diabetes and obesity, and the rising incidence of chronic diseases such as heart disease, stroke, cancer, chronic respiratory diseases, tuberculosis, etc., are driven by several factors, including medical and health issues that may be traced to significant problems with environmental monitoring. There also exist, of course, serious monitoring challenges for security and military and agriculture/food applications.81 Many research articles have been published in recent years that discuss the application of electrochemical sensors for food analysis,82,83 and the detection of specific analytes in environmental applications.84–86 Nanomaterials based electrochemical sensing platforms have been developed for the recognition and quantification of chemical contaminants in water and food. The primary focus of this review is the application of electrochemical sensors for environmental and food safety and security. Chemical contaminants may be classified chiefly as heavy metals, small organic and inorganic pollutants, pharmaceutical and personal care products, toxins of microbial origin, and pathogens.87,88 The detection of chemical contaminants may originate from a variety of sources, such as the improper use or storage of pesticides and veterinary drugs, and the creation of chemicals during processing techniques.89 The high sensitive and selective analysis of food and water contaminants is vital, not only for human and wildlife protection, but also aids global trade, by assisting with the specific identification of contaminated products, and thereby limiting its distribution.90
Chemical contaminants such as heavy metals, nitrates, sulphates, phenolics, pesticides and nerve agents, all have potentially serious health implications in terms of causing illness, or by limiting the efficacy of medicines through the development of drug resistance.91,92 Physicochemical methods such as liquid chromatography-tandem mass spectrometry (LCMS/MS) are usually employed to detect the presence of these toxic compounds; however, these techniques tend to be expensive, complicated, and time consuming.37 Electrochemical sensing methods have the potential to be integrated into portable and rapid detection tools to greatly reduce the expense and time for chemical contaminant analysis.
4.1. Detection of heavy metals
The increasing heavy metal contamination of fresh water and food systems in worldwide has become a core problem facing humanity. The detection and determination of heavy metal ion levels in potable water supplies and food samples is essential to human health and safety.93 The real-time, high resolution measurement of heavy metals such as mercury, lead, cadmium, chromium and arsenic, etc. in water and foods at ultralow concentrations are required for maintaining safe water supplies.94 Due to the non-biodegradable, non-thermo degradable, and toxic nature of heavy metals, they tend to accumulate in living organisms owing to their low clearance rates. Various health problems, including developmental disorders and organ failure are typically associated with exposure to these elements.95 Mercury has become emphasized within the family of heavy metals, and its sources of contamination are derived mainly from coal combustion, industrial production, and agricultural chemicals. Water soluble mercuric ions (Hg(II)) are an important form of this heavy metal, which has a high toxicity; it can also exist in the environment in a variety of forms.96 Mercuric ions can accumulate in the human body through the food chain and lead to permanent damage to the brain, and to other chronic diseases.97 In 2005, the U.S. Environmental Protection Agency set the maximum permissible level of mercuric ions in potable water at 10 nM. The sensing of mercury is of critical importance in environmental and food safety, as well as clinical toxicology. Zeng and co-workers98 recently developed a three-dimensional electrochemical sensor, which was constructed using mercury-specific oligonucleotides, gold nanoclusters, and an anionic intercalator, as shown in Fig. 4. This sensor spatially captured electroactive mercuric ions to enable trace mercury measurements with high sensitivity due to the steric reaction field in the electrode surface microenvironment that was formed by the Au nanoclusters, thereby exhibiting strong environmental adaptability and high selectivity. A low detection limit was achieved at 0.01 nM with a linear range of 0.05 to 350 nM.
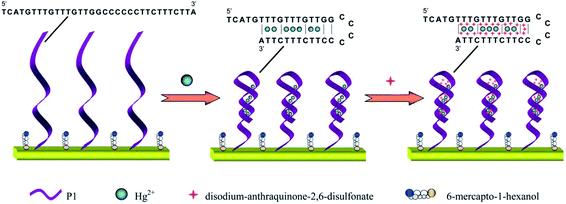 |
| Fig. 4 Detection strategies for a mercuric ion sensor using mercury-specific oligonucleotides, gold nanoclusters, and an anionic intercalator. Reproduced with permission from ref. 98 Copyright© 2014 Royal Society of Chemistry. | |
The Environmental Protection Agency has assessed that a portion of human exposure to lead and cadmium occurs through contaminated drinking water. The permissible level of lead and cadmium in drinking water is 3.0 and 10.0 mg L−1, respectively, as established by the World Health Organization (WHO). Consequently, the accurate detection and determination of these heavy metals at trace levels is of particular importance. Kong et al.99 developed a rapid and sensitive electrochemical sensor for the determination of trace amounts of Pb(II) and Cd(II) in aqueous solutions using fiber-in-tube solid-phase microextraction (SPME) in combination with anodic stripping voltammetry (ASV). These researchers investigated the major factors that influenced the preconcentration and extraction of the metal ions, pH of the aqueous solution, filling mode, sample flow rate, eluent concentration, eluent volume, and effluent flow rate with a limit of quantification of 0.001 μg L−1.
A facile electrochemical sensor was designed with functionalized GO sheets on a Au surface, resulting in the complexation-enhanced electrochemical detection of heavy metal ions, such as Pb(II), Cu(II) and Hg(II), with improved low level detection limits.100 Aneesh et al.101 demonstrated the electrochemical synthesis of a gold atomic cluster–chitosan nanocomposite film modified gold electrode for the ultra-trace determination of mercury over a wider calibration range of 1 fM to 0.1 μM with a limit of detection (LOD) of 0.8 fM. Carcinogenic chromium ions (Cr(VI)) are considered as toxic species, and highly soluble and mobile in both biological and natural ambient systems.102 Significant volumes of chromium compounds are discharged into the environment from various industrial processes such as mineral processing, pigments and electroplating. In USA, the allowable groundwater level of naturally occurring Cr(VI) can be up to 220 mg L−1 (4.2 μM).103 A provisional limit of 50 mg L−1 (0.96 μM) in groundwater was set by The World Health Organization (WHO) in order to prevent Cr(VI) toxicity in humans and the environment.104 Our group has recently developed a facile and effective electrochemical Cr(VI) sensor using Au NPs-decorated TiO2 NTs.70 Fig. 5A shows the SEM images of Au NPs decorated TiO2 NTs. The Au NPs (∼10 nm in diameter) were well dispersed on the as-prepared TiO2 NTs. The diameter of these nanotubes was estimated to be ∼120 nm, with a wall thickness of ∼60 nm and a length of 3–4 μm. Due to its nanoparticle/nanotubular heterojunction infrastructure, the electrocatalytic activity of Ti/TiO2NT/AuNPs electrode exhibited an almost 23-fold improvement in activity as compared to a smooth polycrystalline gold electrode. The Ti/TiO2NT/AuNPs electrode provided a wide linear concentration range of 0.1 μM to 105 μM, a low detection limit of 0.03 μM, and a high sensitivity of 6.91 μA μM−1 Cr(VI) using and amperometric technique, as shown in Fig. 5B and C. Furthermore, the Ti/TiO2NT/AuNPs electrode exhibited good selectivity against interference from coexisting Cr(III) and other metal ions, and excellent recovery for Cr(VI) detection in both tap and lake water samples. The phosphinic acid functionalized CNT utilized as a sensing substrate for the sensitive and selective sensing of Cr(VI) in the range of 0.01 to 10 ppb, with a detection limit of 0.01 ppb.105 Rajkumar et al.106 demonstrated an electrochemical sensor for arsenic in controlled laboratory and drinking water samples using a nano Au–crystal violet film, which was fabricated on a glassy carbon electrode. A variety of electrochemical sensors and devices have been proposed and tested for the heavy metal assays in water.107 Some of other developed electrochemical sensors for the detection and determination of heavy metal ions such as Hg(II),108–111 Cr(VI),69,109 Pb(II),113,114 Cd(II),114,115 As(III)116–118 and Cu(II)119 using the nanostructured materials are listed in Table 1.
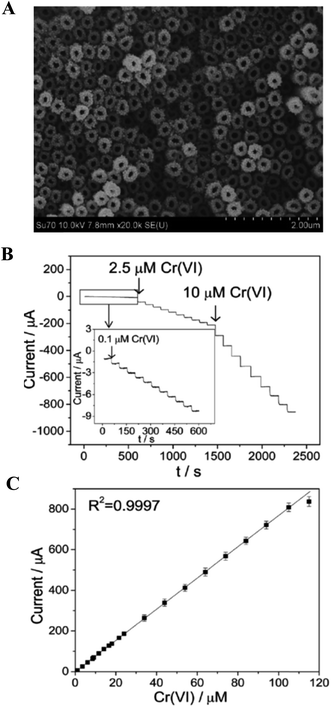 |
| Fig. 5 (A) SEM image of the Au NPs decorated TiO2NT electrode. (B) Amperometric responses at the Au nanoparticle decorated TiO2NT electrode for the successive addition of Cr(VI) with a polarized potential of 0.28 V in 0.1 M HCl. (C) Calibration plot of corresponding amperometric response. Reproduced with permission from ref. 70 © 2014 Royal Society of Chemistry. | |
Table 1 List of electrochemical sensors and biosensors based on nanomaterials for the detection of chemical contaminantsa
Nanomaterials |
Analytes |
Samples |
Detection methods |
Linear range |
Detection limit |
Ref. |
CILE – carbon ionic liquid electrode; MB – methylene blue; NFs – nanofibres; p-ABSA – p-aminobenzene sulfonic acid; Co(II)MTpAP – meso-tetra(para-aminophenyl)porphyrinato cobalt(II); CoPcF – tetrakis(3-trifluoromethylphenoxy)phthalocyaninato cobalt(II); SO(x) – sulfite oxidase; PB NPs/PPY – Prussian blue nanoparticles/polypyrrole composite; TPDT – amine functionalized silicate sol–gel matrix; ZSM – metallosilicates; OSi@CS – organo silica@chitosan; EDA – ethylenediamine; TT – terthiophene; HCF – hexacyano ferrate; NNH – nano nickel hydroxide; PANI – polyaniline; PGMA – poly(glycidyl methacrylate); HRP – horseradish peroxidase; GNs – graphene nanosheets; β-CD – β-cyclodextrin; CH – cholinesterases; 3-APTES – aminopropyltriethoxy silane; AChE – acetylcholinesterase; OMCs – ordered mesopores carbons; LSV – linear sweep voltammetry; DPV – differential pulse voltammetry; SWV – square wave voltammetry; SWASV – square-wave anodic stripping voltammetry; ASV – anodic stripping voltammetry. |
CILE–Au NPs |
Mercury(II) |
Waste and tap water |
SWASV |
0.01 to 50 ppb |
15 ppt |
108 |
MB–DNA–Au NPs |
Mercury(II) |
Tap water |
DPV |
0.2 to 100 ppb |
0.064 ppb |
109 |
Au–Pt NPs–NFs |
Mercury(II) |
River and tap water |
SWASV |
0.1 to 10 ppb |
0.008 ppb |
110 |
DNA–Au NPs |
Mercury(II) |
River water |
DPV |
0.5 to 120 nM |
0.06 nM |
111 |
Quercetin–MWCNT |
Chromium(VI) |
Mineral, river and tap water |
ASV |
1 to 20 μM |
0.3 μM |
112 |
Au NPs–TiO2NT |
Chromium(VI) |
Lake and tap water |
Amperometry |
0.1 to 105 μM |
0.03 μM |
70 |
DNA–SWCNT |
Lead(II) |
River and tap water |
DPV |
1 nM to 10 nM |
0.4 nM |
113 |
MWCNT |
Lead(II) |
Tap water, waste water and food samples |
SWASV |
0.4 to 1100 ng mL−1 |
0.25 ng mL−1 |
114 |
Cadmium(II) |
SWASV |
1 to 1200 ng mL−1 |
0.74 ng mL−1 |
Stannum/p-ABSA–GR |
Cadmium(II) |
Waste, tap and farm land irrigation waster |
SWASV |
1.0 to 70 μg L−1 |
0.05 μg L−1 |
115 |
Ag NPs–GO |
Arsenic(III) |
River and ground water |
SWASV |
13.33 to 375.19 nM |
0.24 nM |
116 |
Au NPs–Te-hybrid |
Arsenic(III) |
N/A |
SWASV |
0.1 to 10 ppb |
0.0026 ppb |
117 |
Au NPs–Fe3O4 |
Arsenic(III) |
Waste water |
SWASV |
0.01 to 1 ppb |
0.00097 ppb |
118 |
Aptamer–Au NPs |
Copper(II) |
Industrial electrolyte bath (gold, copper and brass) |
SWV |
0.1 nM to 10 μM |
0.1 pM |
119 |
MWCNT–iron(II) porphyrin |
Cyanide |
N/A |
SWV |
40 nM to 150 μM |
8.3 nM |
134 |
Au NPs/Co(II)MTpAP |
Nitrites |
Well water |
Amperometry |
0.5 μM to 4.7 mM |
60 nM |
135 |
CoPcF–MWCNT |
Nitrites |
N/A |
Amperometry |
0.096 μM to 340 μM |
0.062 μM |
136 |
Au NPs–MWCNT |
Nitrites |
Mineral water, tap water, sausage, salami and cheese |
SWV |
0.05 μM to 250 μM |
0.01 μM |
137 |
Fe2O3–RGO |
Nitrites |
Tap water |
DPV |
50 nM to 0.78 mM |
15 nM |
138 |
SOx–PB NPs–PPY |
Sulphites |
Red and white wine |
CV |
0.5 to 1000 μM |
0.1 μM |
139 |
Co NPs |
Sulphites |
Fruits, wine, tap and drinking water |
CV |
1 to 59 μM |
0.4 μM |
140 |
p-Aminophenol–MWCNT |
Sulphites |
Liquor, boiler, waste, river and drinking water |
SWV |
0.2 to 280 μM |
0.09 μM |
141 |
EDA–MWCNT |
Nitric oxide |
Rat liver tissue |
DPV |
95 nM to 11 μM |
95 nM |
142 |
Pt NPs–acetylene black |
Nitric oxide |
Rat liver tissue |
DPV |
0.18 to 120 μM |
50 nM |
143 |
RGO–Au–TPDT NRs |
Nitric oxide |
N/A |
Amperometry |
10 to 140 nM |
6.5 nM |
144 |
Nanoporous Au |
Phenylamine |
N/A |
Amperometry |
5 to 25 μM |
0.5 μM |
162 |
Nano-Zr–ZSM-5 |
p-Aminophenol |
River and tap water |
DPV |
60 nM to 500 μM |
26 nM |
163 |
o-Aminophenol |
120 nM to 500 |
30 nM |
Aptamer–MWCNT |
Polychlorinate biphenyl |
Serum |
CV |
0.16 to 7.5 μM |
10 nM |
164 |
Pt–Au–OSi@CS |
Catechol |
N/A |
Amperometry |
0.06 to 90.98 μM |
0.02 μM |
165 |
Hydroquinone |
0.03 to 172.98 μM |
0.01 μM |
RGO–TT–CNT |
Hydroquinone |
Tap water |
DPV |
0.01 to 200 μM |
7.61 nM |
166 |
Catechol |
0.5 to 200 μM |
11.7 nM |
3D-GR |
Catechol |
Lake and tap water |
DPV |
0.25 μM to 12.9 μM |
80 nM |
167 |
Fe3O4–PGMA–HRP |
Phenol |
N/A |
Amperometry |
0.5 to 8.5 mM |
0.028 mM |
168 |
Catechol |
0.5 to 11.0 mM |
0.046 mM |
CuNPs–HCF |
Hydroquinone |
N/A |
Amperometry |
10 μM to 0.2 mM |
2.2 μM |
169 |
ds DNA–PANI |
Hydroquinone |
Tap water |
DPV |
12.5 μM to 32 μM |
0.9 μM |
170 |
NNH–MWCNT |
4-Chlorophenol |
River, lake and tap water |
DPV |
1.0 to 750 μM |
0.5 μM |
171 |
MWCNT |
Bisphenol A |
Mineral water |
CV |
0.099 to 5.8 μM |
0.032 μM |
172 |
Pt–Pd NPs/CNT–RGO |
1,3,5-Trinitrophenol |
N/A |
DPV |
1 to 115 ppb |
0.52 ppb |
173 |
Nitrobenzene |
1 to 155 ppb |
0.42 ppb |
OPH-bacteria–OMCs |
Paraoxon |
Sea, tap and sewage water |
Amperometry |
0.05 to 25 μM |
9 nM |
188 |
Parathion |
0.05 to 25 μM |
15 nM |
Methyl parathion |
0.05 to 25 μM |
10 nM |
OMCs |
Paraoxon |
Sea, tap and sewage water |
DPV |
0.015 to 10 μM |
1.9 nM |
189 |
Parathion |
0.01 to 10 μM |
2.1 nM |
Methyl parathion |
0.015 to 10 μM |
3.4 nM |
GdHCF–GNs |
Methyl parathion |
River and tap water |
DPV |
0.008 to 10 μM |
1 nM |
190 |
MWCNT–CeO2–Au NPs |
Methyl parathion |
River water and soil |
DPV |
0.1 nM to 0.1 μM |
30.2 fM |
191 |
ZrO2NPs–GR |
Methyl parathion |
Garlic and cabbage |
SWV |
0.002 to 0.9 μg mL−1 |
0.6 ng mL−1 |
192 |
β-CD–GR |
Methyl parathion |
Sea water |
DPV |
0.3 to 500 ppb |
0.05 ppb |
193 |
CuO NWs–SWCNT |
Malathion |
N/A |
DPV |
0.3 to 600 nM |
0.3 nM |
144 |
PANI–SWCNT |
Malathion |
Tap water |
DPV |
0.2 μM to 1.4 μM |
0.2 μM |
194 |
CoO–RGO |
Carbofuran |
Grapes, orange, tomato and cabbages |
DPV |
0.2 to 70 μM |
4.2 μg L−1 |
195 |
AChE–Fe3O4–CH |
Carbofuran |
Cabbages |
SWV |
5.0 nM to 0.14 μM |
3.6 nM |
196 |
AChE–(3-APTES)–SWCNT |
Sarin |
N/A |
LSV |
20 to 60 nM |
15 nM |
206 |
Diisopropyl fluorophosphate |
20 to 80 nM |
25 nM |
AChE–RGO |
Dichlorvos |
River water |
Amperometry |
5 ng mL−1 to 20 μg mL−1 |
2 ng mL−1 |
207 |
Au–ZrO2NPs–SiO2 |
Paraoxon-ethyl |
N/A |
SWV |
1.0 to 500 ng mL−1 |
0.5 ng mL |
208 |
GR |
Isoproturon |
River and rice field water, tomato and lettuce |
SWSV |
0.02 to 10.0 mg L−1 |
0.02 mg L−1 |
209 |
Carbendazim |
0.5 to 10.0 mg L−1 |
0.11 mg L−1 |
4.2. Detection of inorganic anions and compounds
Environmental and food contaminants are the main vectors that may negatively impact both human and animal life. These contaminants impart mild to severe short-term, long term, or even deadly effects. The contamination of inorganic nitrite ions (NO2−) pollutants in water and food supplies continues to be a significant issue throughout the world. They can easily form carcinogenic nitrosamines by readily binding with hemoglobin, and causes methemoglobinemia, primarily in infants.120 Nitrites are present in foods as a preservative compound in meats, cheeses, and natural vegetables.121,122 The maximum permissible concentration of nitrite in portable water is 2.17 μmol L−1.123 Due to increasingly stringent environmental protection and public health measures, the quantitative analysis of nitrites in drinking water has more and more relevance. Miao et al.124 developed an electrochemical sensor by employing platinum nanoparticles for the detection of nitrites with a detection resolution limit of 5 μM, and this method was successfully applied to the determination of nitrites with ambient lake water. The Au NPs coupled with MWCNT based sensing platform was prepared for nitrite detection incorporating various experimental parameters for the voltammetric response of nitrites.125 The prepared sensor showed good selectivity in response to the most common ions and many environmental organic pollutants, with a sensitivity of 0.42 μA μM−1, and a low detection limit of 0.01 μM. A bi-enzymatic biosensor was developed by Karunakaran and co-workers126 for the simultaneous detection of nitrite and nitrate ions using copper, zinc superoxide dismutase (SOD1) and nitrate reductase (NaR) co-immobilized on a CNT–polypyrrole (PPy) nanocomposite modified electrode. Fig. 6 depicts the fabrication of a biosensor for the detection and determination of nitrite and nitrate ions.126 A CNT integrated PPy composite was prepared by casting CNT onto an as-prepared PPy film modified Pt electrode. A 1
:
1 ratio mixture of enzyme solutions was coated onto a CNT–PPy–Pt electrode, resulting in a composite electrode. The electrocatalytic activity of SOD1 occurred toward nitrite oxidation at 0.8 V, and showed a linear range of 100 nM to 1 mM, with a detection limit of 50 nM, and sensitivity of 98.57 nA mM−1 cm−2. The electrocatalytic reduction of nitrate ions occurred at −0.76 V using co-immobilized NaR and exhibited a linear range of 500 nM to 10 mM, with a detection limit of 200 nM, and sensitivity of 84.57 nA mM−1 cm−2.
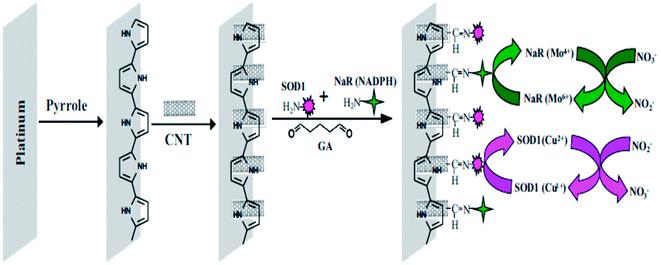 |
| Fig. 6 Construction of a NaR–SOD1–CNT–PPy–Pt electrode and reactions involved during the simultaneous determination of NO2− and NO3−. Reproduced with permission from ref. 126 © 2014 Elsevier. | |
Sulphite ions (SO32−) pollutants are widely utilized as a preservative, bleaching agent, reducing agent and antioxidant in the food industry, and also as a food additive in many countries. It may give rise to headaches, nausea, dizziness, asthma and other allergic reactions if sulphite levels are exceeded. The U.S. Food and Drug Administration (FDA) requires that sulfites must be declared on food labels when it is used as a food ingredient, a processing aid, or when present as an indirect food constituent (e.g., dried fruit pieces), with a permissible concentration in foods of ≥10 ppm. The enzymes including sulfite oxidase, tetrathiafulvalene modified screen-printed carbon electrode based biosensors were developed for the determination of sulfites in wine.127 The amperometric detection of sulfite via simple flow injection using a carbon nanotube–PDDA–Au NPs modified GC electrode was reported by Amatatongchai et al.128 The linear working range for the determination of sulfite was 2–200 mg L−1 with a detection limit of 0.03 mg L−1 and the sensor was applied to the determination of sulfite in fruit juices and wines with a throughput of 23 samples per hour. The simultaneous electrochemical sensor was developed by Maduraiveeran et al.,129 utilizing Au NPs, which were embedded in an amine functionalized silicate sol–gel (APS) modified electrode, was employed for the detection and quantification of toxic hydrazine, sulfite, and nitrite compounds in pH 7.2, as shown in Fig. 7. Fig. 7A displays a schematic depiction of Au nanoparticles dispersed in 3D network with the simultaneous electrocatalytic oxidation of hydrazine, sulfite and nitrite. The prepared Au NPs based electrode showed that the electrooxidation peaks appeared at 0.05, 0.2 and 0.55 V for hydrazine, sulfite and nitrite in pH 7.2, with a large decrease in the overpotentials to the extent of ∼750 mV, ∼600 mV, and ∼250 mV, respectively, as shown in Fig. 7B. This figure also shows the LSV responses observed for the hydrazine, sulfite and nitrite mixture at the Au NPs modified GC electrode, with the successive addition of their concentrations. The linearly increased anodic currents were obtained with the elevated concentration of analytes (Fig. 7C).
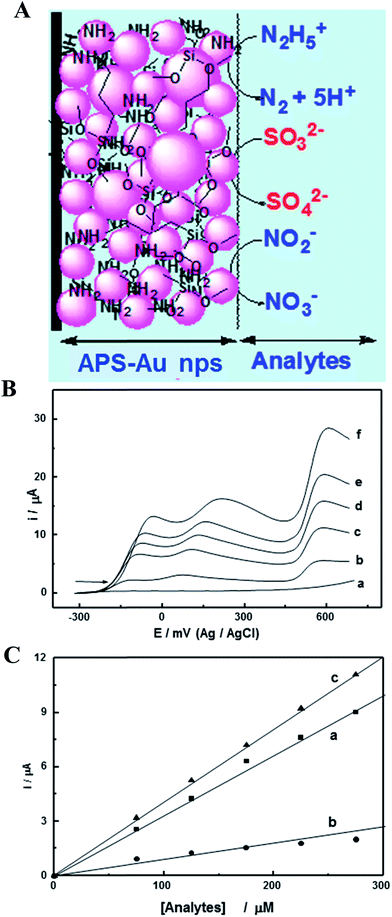 |
| Fig. 7 (A) Schematic representation of an APS–Au NPs modified GC electrode and simultaneous electrocatalytic oxidation of N2H4, SO32− and NO2−. (B) LSVs recorded at APS–AuNPs modified GC electrode for the mixture of N2H4, SO32− and NO2− with the successive addition of concentrations in 0 (a), 200 μM (b), 300 μM (c), 400 μM (d), 500 μM (e) and 600 μM (f) in 0.1 MPBS (pH 7.2). (C) Corresponding calibration plots for N2H4 (a), SO32− and NO2− (c). Reproduced with permission from ref. 129 © 2007 Elsevier. | |
The loading of Au on SnO2 hollow microtubes for the non-enzymatic detection of H2O2 was reported using an amperometric method. The linear range was measured to be from 10 μM to 1 mM with the detection limit of 0.60 μM.130 Furthermore, this non-enzymatic sensor exhibited excellent selectivity for H2O2 when challenged with other normally co-existing electroactive species. The Pd NPs decorated MWNT (PdNPs/MWNT) electrocatalyst based electrochemical sensor was demonstrated for the determination of bromate ion by Li et al.131 Bromate has been extensively used as a food additive in the production of fermented beverages and fish pastes, and also has been found in drinking water as a disinfection by-product (DBP).132 The International Agency for Research on Cancer has classified bromate as a Group 2B carcinogen, as it has been reported that bromate ion may cause renal cell tumors.133 The electrochemical reduction of bromate was obtained at between 0.15 and −0.25 V. The effects of different factors, including the scan rate, temperature and the initial concentration of bromate ions on bromate determination were studied in depth. An amperometric method showed that the PdNPs/MWNT modified electrode could be successfully employed as a sensor for bromate in the concentration range from 0.1 to 40 mM, with a sensitivity of 768.08 μA mM−1 cm−2. These Pd NPs/MWNT electrodes have good potential for applications in fabrication of bromate sensors. Salimi et al.134 demonstrated the use of MWNTs and an iron(III)–porphyrin film as a sensing substrate for the detection of chlorate (ClO3−), bromate (BrO3−) and iodate (IO3−). The greatly improved facilitation of electron transfer between Fe(III)P and CNTs that were immobilized on the electrode surface was the factor behind the enhanced electrocatalytic activity, with detection limits of 0.5 μM (chlorate), 0.6 (bromate), and 2.5 μM (iodate). This sensor possessed an excellent electrochemical reversibility of the redox couple, good reproducibility, high stability, a low detection limit, good longevity, rapid amperometric response time, wide linear concentration range, technical simplicity, and the possibility of rapid preparation and scale up. Furthermore, the electrochemical detection of inorganic anions including, cyanide,135 nitrites,136–138 sulphites139–141 and nitric oxide142–144 using nanomaterials are included in Table 1.
4.3. Detection of phenolic compounds
Phenolic compounds are known to be widespread in the environment from industrial waste due to their use as intermediates in the production of pharmaceuticals, wood preservatives, explosives, and in leather treatments. These substances have a toxic effect on humans, animals and plants, even at very low concentrations.145 A variety of ∼165 phenolic compounds are known to have a toxic effect on the environment. Phenolic compounds, as well as their vapors, are highly corrosive to the eyes, the skin, and the respiratory tract. Due to phenolics' caustic and anti-adipose properties, prolonged skin contact with phenol may cause dermatitis, or even second and third-degree burns. These compounds may also have harmful effects on the central nervous system and heart, resulting in dysrhythmia, seizures, coma and kidney trauma as well.146 The U.S. Environmental Protection Agency has established a permissible level of 1 μg L−1 for all common phenolic compounds,147 and the European Community (EC) Directive specifies a legal permissible level of 0.5 μg L−1 for phenolic compounds that are intended for human consumption.148 Owing to their toxicity and persistence in the environment and in foods, the high resolution detection and quantification of phenolic compounds are of principal importance.149,150 A variety of biological components, including microorganisms, enzymes, antibodies, antigens, nucleic acids, etc. may be used in the fabrication of biosensors for the detection and determination of phenolic compounds. Tyrosinase, peroxidase, laccase, glucose dehydrogenase, cellobiose dehydrogenase etc., are most commonly used enzymes for the fabrication of phenolic compound biosensors.
An enzymatic amperometric biosensor that was based on horseradish-peroxidase (HRP) and methylene blue (MB),151 with chitosan on Au-modified TiO2NT arrays, for the detection of trophenol, as shown in Fig. 8A. The dimensions of the TiO2NT that were fabricated using anodization, were typically ∼90 nm outer diameter, ∼70 nm inner diameter, with a ∼20 nm wall-thickness, as shown in Fig. 8B. The effective immobilization of biomolecules may be obtained by cumulative adhesion and integrity of the prepared TiO2NT layer. Fig. 8C depicts the amperometric responses of three different electrodes (a–c) in the presence of 20 μM H2O2, for the addition of 3-nitrophenol, and the response of the TiO2/Au/HRP–MB electrode was much higher than that of TiO2NT/HRP and TiO2NT/Au/HRP electrodes. The fabricated sensor in this study exhibited a linear response in the range from 0.3 μM to 120 μM for 3-nitrophenol (Fig. 8D), with a detection limit of 90 nM.151 Hernandez et al.152 demonstrated the electrochemical detection of phenolic compounds in environmental samples based on the horseradish peroxidase enzymatic reaction, by means of SWV. This sensor was coupled with a multivariate calibration method for the detection of five phenolic compounds including phenol, p-aminophenol, p-chlorophenol, hydroquinone and pyrocatechol. The LODs oscillated from 0.6 to 1.4 μM, based on the calibration model. A sensitive amperometric biosensor based on a GR–silkpeptide/tyrosinase nanointerface was developed for the detection and quantification of phenolic compounds.153 The LODs were calculated as 0.23, 0.35 and 0.72 nM for catechol, phenol and BPA, respectively, with good repeatability and long-term stability. This group also tested preliminarily practical applications in the detection and determination of BPA that leaches from plastic drinking containers. The immobilizing of tyrosinase on poly(thionine)/nafion/MWCNT composite film electrode was fabricated for matairesinol biosensor. The CNTs uniformly integrated in the film formed a 3D electron conductive network and enabled the low detection limit of 37 nM.154 The detection of phenolic compounds in plants using various electrochemical methods have been reviewed by Dobes et al.,155 and the presence of phenolic compounds in foods was cataloged.
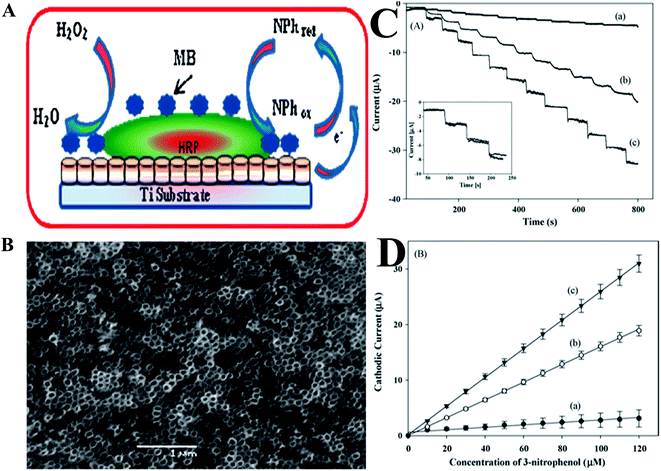 |
| Fig. 8 (A) Scheme for designing a biosensor for nitrophenol detection. (B) SEM image of TiO2NT arrays grown directly on Ti substrates. (C) Amperometric response of TiO2NT/HRP (a), TiO2NT/Au/HRP (b), and TiO2NT/Au/HRP–MB (c) on Ti electrodes with the addition of 10 μM 3-nitrophenol in 0.1 MPBS (pH 7.0) in the presence of 20 μM H2O2, Eapp = −0.05 V. Inset: amperometric response of the TiO2NT/Au/HRP–MB electrode following 45 days under identical experimental conditions (solid line); (D) calibration plots of the proposed sensors for 3-nitrophenol. Reproduced with permission from ref. 151 © 2009 Elsevier. | |
A non-enzymatic sensor was developed for the detection of p-nitrophenol using a poly(methylene blue)-modified GC electrode with a detection limit of 90 nM.156 The proposed sensor was applied to detect p-nitrophenol in real water samples using a DPV method. TiO2-modified graphene nanocomposite was used for the detection of p-chlorophenol with a low detection limit of 0.02 μM.157 Furthermore, an electrogenerated chemiluminescent sensor was demonstrated,158 which was based on a hybrid GR/MWCNT/Au nanocluster, for the detection and determination of phenolic compounds. The sensor signal increased linearly with elevated concentrations of catechol, ranging from 1.0 to 80 μM, with a detection limit of 0.3 μM. Moreover, the prepared sensor was applied to other phenolic compounds, such as hydroquinone, resorcinol, p-cresol, p-chlorophenol and 2-bromophenol, and exhibited a similar enhancement effect on the ECL at the GR/MWCNT/AuNCs/GC; however, it cannot be applied for the simultaneous/multiple determination and discrimination of these compounds using this method. The composite of MWCNT–poly(diphenylamine) modified GC electrode was used for the amperometric detection of p-nitrophenol in pH 7.159 The composite electrode showed a good electrocatalytic reduction of p-nitrophenol, and exhibited a linear range from 8.9 μM to 1.43 mM with a sensitivity of 0.6322 μA μM−1 cm−2. A chitosan–Fe3O4 nanocomposite based sensor was fabricated for the detection and determination of bisphenol-A (BPA) using a DPV method. Chitosan is a polyaminosaccharide, which provides for a stable and homogenous suspension of Fe3O4 due to their chemical structures during sensor fabrication.160 Yang et al.161 prepared an electrochemical sensor based on Au NPs–GR nanohybrid bridged 3-amino-5-mercapto-1,2,4-triazole-functionalized MWCNT for the simultaneous determination of hydroquinone, catechol, resorcinol, and nitrite, as shown in Fig. 9. Due to the synergistic effects that exist between MWCNT–SH and Au NPs–GR, along with the excellent electrocatalytically active film forming ability of MWCNT–SH@Au–GR, the prepared MWCNT–SH@Au–GR sensor was used for the simultaneous determination of the toxic compounds, hydroquinone, catechol, resorcinol, and nitrite with LODs of 4.17 μM, 1.00 μM, 7.80 μM and 23.5 μM, respectively. The proposed simultaneous sensor showed excellent sensitivity, high selectivity and good stability with practical applications in the assessment of water samples. Nanostructured materials based electrochemical sensing platforms for phenolic compounds including phenylamine,162 o and p-aminophenol,163 polychlorinate biphenyl,164 catechol,165–169 hydroquinone,166,167,170 p-chlorophenol,171 bisphenol A,172 phenol,168 1,3,5-trinitrophenol,173 nitrobenzene,173 are also comprised and listed in Table 1.
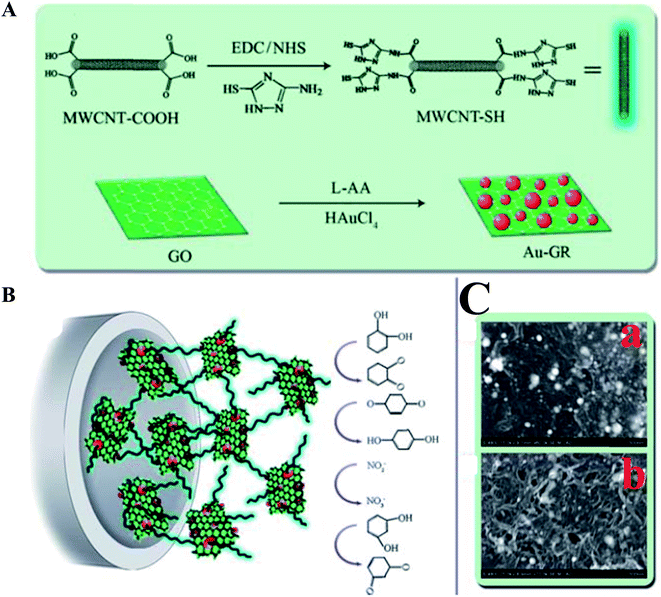 |
| Fig. 9 (A) Preparation process for MWCNT–SH and Au–GR. (B) Schematic depiction of the MWCNT–SH@Au–GR electrode. (C) SEM images of Au–GR nanohybrid (a) and MWCNT–SH@Au–GR hybrid material (b). Reproduced with permission from ref. 161 © 2013 Royal Society of Chemistry. | |
4.4. Detection of pesticides
Pesticides are regularly employed in agriculture worldwide, leading to the widespread contamination of air, water, soil and agricultural products. Due to their ability to accumulate, coupled with their potentially long-term effects on living organisms, pesticides are considered as the most dangerous of environmental contaminants.174 In particular, the presence of pesticides in the environment is especially hazardous, leading to several serious human health problems such as asthma attacks, skin rashes, chronic disorders, and neurological diseases.175 Intense research efforts have been invested in the development of sensitive and selective sensors for the detection and quantification of pesticides.176 Details on nanomaterials based electrochemical and optical sensors for the detection of pesticides and pathogens may be found in the comprehensive review articles authored by Merkoc and co-workers.177,178 State-of-art receptor selection, the use of different transduction techniques, rapid screening strategies, and the application of various biosensors have been developed for food and environmental safety and security applications. An important principle for the development of pesticide biosensors is derived from the correlation between the toxicity of pesticides and decreased enzymatic activity. The quantitative measurement of enzymatic activity, prior to, and following exposure to a target analyte relies on the development of biosensors, in particular, (i) determination of initial enzymatic activity, (ii) incubation of a biosensor in a solution that contains pesticides, and (iii) final measurement of residual activity. Amperometry and potentiometric transduction techniques are typically utilized to measure the activity of biosensing systems.179,180
Acetylcholinesterase (AChE) based biosensors have been reviewed by Hooda and co-workers169 for the electrochemical detection of organophosphorus compound based pesticides. These researchers discussed a number of characteristic AChE based biosensor features, in terms of fabrication, detection limits, linearity range, incubation time, and storage stability. AChE based biosensors for the detection of OP compounds is an intensive field of research, with myriad applications for environmental monitoring, human health concern, and food industries. An electrochemical sensing platform was reported for the highly sensitive detection of organophosphate pesticides, based on carbon nanotube assemblies deposited on a nanoporous gold electrode, as shown in Fig. 10.181 The immobilized AChE based biosensor model showed excellent activity in relation to its substrate, and enabled the quantitative measurement of organophosphate pesticides in the range from 0.001 to 0.5 μg mL−1 with a LOD of 0.5 ng mL−1. This sensitive electrochemical AChE biosensor, comprised of polyaniline (PANI) and a MWCNT core–shell modified glassy carbon electrode, demonstrated the detection of carbamate pesticides in fruits and vegetables (apple, broccoli and cabbage).182
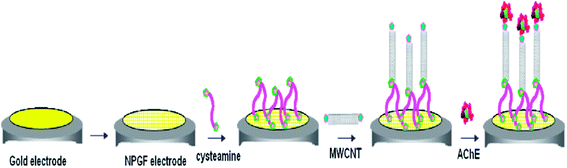 |
| Fig. 10 Scheme for the fabrication of AChE–MWCNT–CA–NPG electrode. Reproduced with permission from ref. 181 © 2014 Elsevier. | |
CNT/conductive polymer composites have exhibited a synergetic effect, leading to the augmentation of the electronic and mechanical characteristics of the constituent components.183 An electrochemical carbofuran pesticide biosensor has been reported recently, which was based on esterases from Eupenicillium shearii FREI-39 endophytic fungi.184 This enzymatic biosensor was prepared using the physical adsorption of the isolated endophytic fungus Eupenicillium shearii FREI-39 esterase on halloysite clay using graphite powder, MWCNT and mineral oil, for the determination of carbofuran pesticides via the inhibition of the esterase using SWV. A linear plot was obtained for carbofuran detection in the range from 5.0 to 100.0 μg L−1 with a LOD of 1.69 μg L−1. Fig. 11 depicts the preparation of an electrochemical immunosensor based on an assembly of SWNT that was formed via micro contact printing (MCP) on GC substrates for the simultaneous detection of endosulfan and paraoxon.185 Ferrocenedimethylamine (FDMA) and pyrroloquinoline quinone (PQQ) redox probes were loaded into a four-channel poly(dimethylsiloxane) (PDMS) patterning template. The change in the environment, due to the attenuation of electron transfer to the redox molecules and the association and dissociation of antibodies at the sensing interface, causes the modulation of the electrochemical behaviors of the redox molecules. This strategy has potential to lead to the design of portable sensing devices for the on-site detection of pesticides in environmental monitoring. A hybrid copper oxide nanowire (CuO NW)/SWCNT based non-enzymatic electrochemical sensor was developed by Huo et al.186 for the detection of organophosphorus pesticides (OPs). A DPV technique was applied for a malathion sensor that showed a wide dynamic range, with a LOD of 0.1 ppb (0.3 nM), with good selectivity against other common pesticides, inorganic ions and sugars, as well as good stability and reproducibility. A potentiometric biosensor was described for the pesticide atrazine, using urease biomolecules that were immobilized onto an insulator–semiconductor electrode with various additional materials, such as glutaraldehyde (as a cross-linking agent), bovine serum albumin, coated Fe3O4 nanoparticles, cationic poly(allylamine hydrochloride), and anionic poly(sodium 4-styrenesulfonate) polyelectrolytes, as shown in Fig. 12.187 The sensing of atrazine was enabled via inhibitory activity on the urease biosensor, with a LOD of 0.13 μM. Some other recently demonstrated electrochemical sensors and biosensors for the detection and determination of pesticides such as paraoxon,188,189 parathion,188,189 methyl parathion,188,190–193 malathion,144,194 carbofuran,195,196 and carbaryl195 are contained in Table 1.
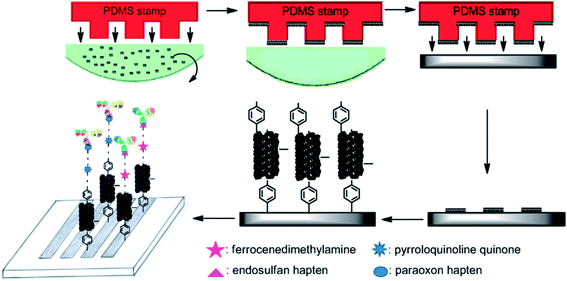 |
| Fig. 11 Schematic illustration of fabrication of simultaneous electrochemical immunosensor array for endosulfan and paraoxon. Reproduced with permission from ref. 185 © 2014 Elsevier. | |
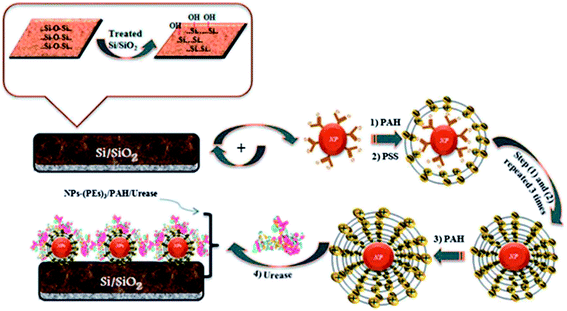 |
| Fig. 12 Biosensor construction using Fe3O4 and polyelectrolyte materials for atrazine detection. Reproduced with permission from ref. 187 © 2013 Royal Society of Chemistry. | |
4.5. Detection of chemical warfare agents
Chemical warfare agents (CWAs) are toxic chemicals that have been used historically in an effort to gain military superiority. They have been utilized on the battlefield as lachrymatory agents (causing respiratory irritation) during the World War I, and by the French and German armies in recent modern history.197 The discovery of far more potent agents has rendered these early non-lethal chemicals obsolete as warfare agents, and thus they are currently used in riot control applications. Derived from the toxic effects of CWAs on humans, CWAs are classified as seven types: nerve, blister or vesicant, chocking or pulmonary, vomiting, asphyxiant or blood, tear or lachrymatory, and incapacitating agents.198 The periodic use of chemical weapons of war and terror over history, has created an urgent need for the development of rapid CWA detection devices. Prior to the World War II, the presence of CWA vapors or other toxic chemicals, was detected via biological indicators, such as the human nose, or through the use of small animals. Modern approaches employ physical-electronic devices in their operation. Electrochemical systems are typically faster, more selective and flexible, as they may be adapted to detect multiple compounds or entire classes of compounds. In USA, a miniaturized CWA detector, dubbed as an individual chemical agent detector (ICAD), has been developed through electrochemical methods. This detector consists of a reusable electronic module (processor, audio alarm, and warning light) and a disposable sensor module that contains a battery and the sensor cells. Black199 undertook a brief historical overview of the development of bioanalytical methods for CWA, with a detailed summary of developing methods for the detection of nerve agents. Bhowmick et al.200 reviewed the detection of chemical warfare agents using surface-immobilized molecules with advanced functionalities. The design and fabrication of smart surfaces may have the capacity to immobilize functional molecules, which perform specific functions under the input of external stimuli, and describes their adequacy as sensors for the detection of CWAs in environmental applications.
A biosensor was reported that was based on cholinesterase inhibition,201 which was assembled on a miniaturized prototype for a paraoxon nerve agent analog. Nerve agents such as Sarin, exhibit more robust inhibitory behaviors than paraoxon, thus the system might be suitable for the detection of lower concentrations of nerve agents. The OP biosensor was developed on the basis of peptide nanotubes (PNTs), and encapsulated horseradish peroxidase, surface coated with AChE, which were attached to gold screen printed electrodes, as shown in Fig. 13.202 In this biosensor, AChE catalyzed the hydrolysis of ATCh to produce thiocholine, which is then oxidized by HRP to produce dithiocholine. PNTs facilitated direct electron transfer between the HRP and the electrode. The prepared biosensor had the capacity to detect OP vapors at low concentrations below 25 ppb. A multidimensional FeO–OH nanoneedle-decorated hybrid polypyrrole nanoparticle (PFF) based electrochemical sensor was demonstrated using a dual nozzle electrospray and heat stirring process for the detection of chemical nerve gas agents.203 A simple amperometric biosensor was fabricated for sarin using Prussian blue modified silver screen-printed electrode was developed using butyrylthiocholine (BTCh) and butyrylcholinesterase (BChE), in the presence of sarin, using an amperometric method with a LOD of 12 ppb.204 Liu et al.205 have developed an indirect detection biosensor using AChE with an amperometric method to monitor the decrease in choline concentrations due to CWA exposure. A variety of nanomaterials based electrochemical sensors for the detection CWA including sarin,205,206 diisopropyl fluorophosphate,206 dichlorvos,207 paraoxon-ethyl,208 isoproturon,209 and carbendazim,209 are summarized in Table 1.
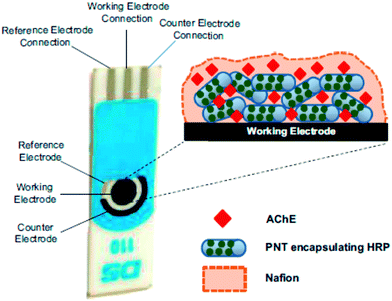 |
| Fig. 13 Schematic illustration of the peptide nanotube (PNT)-modified gold electrode containing encapsulated HRP and the surface attached AChE enzymes. Reproduced with permission from ref. 202 © 2014 Elsevier. | |
5. Conclusions and perspectives
This review has discussed and highlighted recent advancements in nanomaterials based electrochemical sensors for environmental analyses and food safety applications. Electrochemical sensors based on nanostructured materials provide a crucial analytical tool, as the demand for the ultrasensitive, rapid, and selective determination of analytes increases. They may be easily adapted to selective and multiple sensing a range of chemical contaminants inexpensively, in contrast to spectroscopic and chromatographic instrumentation. Some unique physicochemical and electrochemical properties of nanomaterials may facilitate the fabrication of potentially robust electrochemical sensor systems, with attributes such as good biocompatibility and outstanding electrical conductivity. The architectures of nanometric interfaces also afford a suitable environment for biomolecule immobilization, overpotential decreases, and improvements in sensitivity and long-term stability. Additionally, nanomaterial enabled sensors are capable of being embedded into/integrated with portable or miniaturized devices for specific applications, due to the selective catalytic activity, stability and biocompatibility of the sensor design. The design of sensitive and selective simultaneous electrochemical sensor strategies for chemical contaminants that are present in the environment and in foods also warrants exploitation.
Recent advances in synthesis of nanomaterials and electrochemical analytical methods provide a strong and promising future toward the realization of sensitive analytical devices for sensor researchers and manufacturers. The production and commercialization of low-cost nanomaterials based sensing devices remain key issues in the development of analytical devices for the environmental monitoring and food safety industries. The combination of controlled nanostructured architectural design, nanomaterials synthesis, sensor fabrication, processing, integration, miniaturization and testing techniques may offer a possible solution to existing analytical challenges in the fabrication of practical sensing devices.
One of the core issues that requires immediate resolution toward the development of future advanced, highly selective and accurate, portable/miniaturized, rapid (ideally real time), multi-detection environmental and food monitoring sensors, is the significant bottleneck that remains between research laboratory benchtop prototypes and their widespread commercial applications. The highly complex nature of myriad contaminants that increasingly continue to be released into the ambient environment and in foods, will require sophisticated and versatile sensing platforms that have an extremely high degree of selectivity and sensitivity. In the ambient environment, many chemicals have the capacity to combine with others to further exacerbate existing challenges in regard to their precise identification and toxicity assessment. Therefore, extensive collaborative efforts between multidisciplinary science and engineering disciplines (e.g., environmental sciences, nanosciences, limnology, physics, chemistry, electrochemistry, mechanical engineering, electronics, software development, etc.), will be indispensible, and indeed, are urgently warranted for the successful design, development, and implementation of optimized, “market ready” sensors. No longer can these disciplines operate in isolation in view of the immense and highly complex challenges that we presently confront. With continual and rapid advancements across many facets of sensor development, it is anticipated that unprecedented breakthroughs in the emergence of nanomaterials based sensing devices will be realized to seriously address the widespread chemical contamination of the environment and foods in the near future.
Acknowledgements
This work was supported by a Discovery Grant from the Natural Sciences and Engineering Research Council of Canada (NSERC). A. Chen acknowledges NSERC and the Canada Foundation of Innovation (CFI) for the Canada Research Chair Award in Materials and Environmental Chemistry.
References
- TSCA Chemical Substance Inventory, Section 8(b), United States Environmental Protection Agency, 2014.
- V. I. Pye and R. Patrick, Science, 1983, 221, 713 CAS.
- L. Kantiani, M. Llorca, J. Sanchis, M. Farre and D. Barcelo, Anal. Bioanal. Chem., 2010, 398, 2413 CrossRef CAS PubMed.
- E. Ibanez and A. Cifuentes, Crit. Rev. Food Sci. Nutr., 2001, 41, 413 CrossRef CAS PubMed.
- M. A. Hamburg, Science, 2011, 331, 987 CrossRef PubMed.
- V. Garcia-Canâas, C. Simo, M. Herrero, E. Ibanâez and A. Cifuentes, Anal. Chem., 2012, 84, 10150 CrossRef PubMed.
- A. A. Dudarev, E. V. Dushkina, Y. N. Sladkova, P. R. Alloyarov, V. S. Chupakhin, V. M. Dorofeyev, T. A. Kolesnikova, K. B. Fridman, B. Evengard and L. M. Nilsson, Int. J. Circumpolar Health, 2013, 72, 1 Search PubMed.
- S. Rodriguez-Mozaz, M. P. Marco, M. J. L. de Alda and D. Barcelo, Anal. Bioanal. Chem., 2004, 378, 588 CrossRef CAS PubMed.
- G. Hanrahan, D. G. Patil and J. Wang, J. Environ. Monit., 2004, 6, 657 RSC.
- J. Parellada, A. Narváez, M. A. López, E. Domínguez, J. J. Fernández, V. Pavlov and I. Katakis, Anal. Chim. Acta, 1998, 362, 47 CrossRef CAS.
- A. Baeumner, Anal. Bioanal. Chem., 2003, 377, 434 CrossRef CAS PubMed.
- R. Chen, Y. Li, K. Huo and P. K. Chu, RSC Adv., 2013, 3, 18698 RSC.
- A. Bahrami, A. Besharati-Seidani, A. Abbaspour and M. Shamsipur, Electrochim. Acta, 2014, 118, 92 CrossRef CAS PubMed.
- J. Ma, L. Adornato, R. H. Byrne and D. Yuan, TrAC, Trends Anal. Chem., 2014, 60, 1 CrossRef CAS PubMed.
- B. Chen, Z. Wang, D. Hu, Q. Ma, L. Huang, C. Xv, Z. Guo and X. Jiang, Sens. Actuators, B, 2014, 200, 310 CrossRef CAS PubMed.
- A. N. Goldstein, C. M. Echer and A. P. Alivisatos, Science, 1992, 256, 1425 CAS.
- S. E. F. Kleijn, S. C. S. Lai, M. T. M. Koper and P. R. Unwin, Angew. Chem., Int. Ed., 2014, 53, 3558 CrossRef CAS PubMed.
- V. J. Nagaraj, M. Jacobs, K. M. Vattipalli, V. P. Annam and S. Prasad, Environ. Sci.: Processes Impacts, 2014, 16, 135 CAS.
- J. Kirsch, C. Siltanen, Q. Zhou, A. Revzin and A. Simonian, Chem. Soc. Rev., 2013, 42, 8733 RSC.
- R. X. Xu, X. Y. Yu, C. Gao, J. H. Liu, R. G. Compton and X. J. Huang, Analyst, 2013, 138, 1812 RSC.
- D. Tang, J. Tang, B. Su and G. Chen, J. Agric. Food Chem., 2010, 58, 10824 CrossRef CAS PubMed.
- E. Bakker, Anal. Chem., 2004, 76, 3285 CrossRef CAS PubMed.
- A. Hierlemann and R. Gutierrez-Osuna, Chem. Rev., 2008, 108, 563 CrossRef CAS PubMed.
- M. Bonizzoni and E. V. Anslyn, J. Am. Chem. Soc., 2009, 131, 14597 CrossRef CAS.
- M. Amalraj and S. A. John, Electrochim. Acta, 2014, 117, 360 CrossRef PubMed.
- H. S. Toh, K. Tschulik, C. Batchelor-McAuley and R. G. Compton, Analyst, 2014, 139, 3986 RSC.
- J. P. Metters, R. O. Kadara and C. E. Banks, Analyst, 2011, 136, 1067 RSC.
- J. Wang, TrAC, Trends Anal. Chem., 2002, 21, 226 CrossRef CAS.
- A. Baeumner, Anal. Bioanal. Chem., 2003, 377, 434 CrossRef CAS PubMed.
- F. Valentini, M. Carbone and G. Palleschi, Anal. Bioanal. Chem., 2013, 405, 3449 CrossRef CAS PubMed.
- D. A. C. Brownson and C. E. Banks, Analyst, 2010, 135, 2768 RSC.
- W. Zhang, A. M. Asiri, D. Liu, D. Du and Y. Lin, TrAC, Trends Anal. Chem., 2014, 54, 1 CrossRef CAS PubMed.
- D. W. Kimmel, G. LeBlanc, M. E. Meschievitz and D. E. Cliffel, Anal. Chem., 2011, 84, 685 CrossRef PubMed.
- A. Chen and S. Chatterjee, Chem. Soc. Rev., 2013, 42, 5425 RSC.
- A. Chen and B. Shah, Anal. Methods, 2013, 5, 2158 RSC.
- A. Chen and P. Holt-Hindle, Chem. Rev., 2010, 110, 3767 CrossRef CAS PubMed.
- B. Shah, T. Lafleur and A. Chen, Faraday Discuss., 2013, 164, 135 RSC.
- S. Chatterjee, J. Wen and A. Chen, Biosens. Bioelectron., 2013, 42, 349 CrossRef CAS PubMed.
- A. Ahmadalinezhad, G. Wu and A. Chen, Biosens. Bioelectron., 2011, 30, 287 CrossRef CAS PubMed.
- S. Chatterjee and A. Chen, Biosens. Bioelectron., 2012, 35, 302 CrossRef CAS PubMed.
- S. Chatterjee and A. Chen, Anal. Chim. Acta, 2012, 751, 66 CrossRef CAS PubMed.
- A. K. Wanekaya, Analyst, 2011, 136, 4383 RSC.
- K. Scida, P. W. Stege, G. Haby, G. A. Messina and C. D. García, Anal. Chim. Acta, 2011, 691, 6 CrossRef CAS PubMed.
- C. Gao, Z. Guo, J.-H. Liu and X.-J. Huang, Nanoscale, 2012, 4, 1948 RSC.
- J. Ping, Y. Wang, J. Wu and Y. Ying, Electrochem. Commun., 2011, 13, 1529 CrossRef CAS PubMed.
- E. Jaworska, W. Lewandowski, J. Mieczkowski, K. Maksymiuk and A. Michalska, Analyst, 2012, 137, 1895 RSC.
- X. Gong, Y. Bi, Y. Zhao, G. Liu and W. Y. Teoh, RSC Adv., 2014, 4, 24653 RSC.
- S. Yuan, Q. He, S. Yao and S. Hu, Anal. Lett., 2006, 39, 373 CrossRef CAS.
- I. Balan, I. G. David, V. David, A. I. Stoica, C. Mihailciuc, I. Stamatin and A. A. Ciucu, J. Electroanal. Chem., 2011, 654, 8 CrossRef CAS PubMed.
- L. G. Zamfir, L. Rotariu and C. Bala, Biosens. Bioelectron., 2011, 26, 3692 CrossRef CAS PubMed.
- L. S. Fifield and J. W. Grate, Carbon, 2010, 48, 2085 CrossRef CAS PubMed.
- Y. Wang and Y. Hasebe, Anal. Bioanal. Chem., 2011, 399, 1151 CrossRef CAS PubMed.
- W. Tu, J. Lei, L. Ding and H. Ju, Chem. Commun., 2009, 4227 RSC.
- H. Daia, L. Gong, G. Xu, S. Zhang, S. Lu, Y. Jiang, Y. Lin, L. Guo and G. Chen, Electrochim. Acta, 2013, 111, 57 CrossRef PubMed.
- L. Rassaei, F. Marken, M. Sillanpaa, M. Amiri, C. M. Cirtiu and M. Sillanpaa, TrAC, Trends Anal. Chem., 2011, 30, 1704 CrossRef CAS PubMed.
- L. Cui, J. Wu and H. Ju, Biosens. Bioelectron., 2015, 63, 276 CrossRef CAS PubMed.
- K. Warriner, S. M. Reddy, A. Namvar and S. Neethirajan, Trends Food Sci. Technol., 2014 DOI:10.1016/j.tifs.2014.07.008.
- A. Amine, H. Mohammadi, I. Bourais and G. Palleschi, Biosens. Bioelectron., 2006, 21, 1405 CrossRef CAS PubMed.
- G. Maduraiveeran and R. Ramaraj, J. Electroanal. Chem., 2007, 608, 52 CrossRef CAS PubMed.
- D. W. Kimmel, G. LeBlanc, M. E. Meschievitz and D. E. Cliffel, Anal. Chem., 2011, 84, 685 CrossRef PubMed.
- P. S. R. D. Silva, B. C. Gasparini, H. R. A. Magosso and A. Spinelli, J. Hazard. Mater., 2014, 273, 70 CrossRef PubMed.
- Y. Wei, R. Yang, X. Y. Yu, L. Wang, J. H. Liu and X. J. Huang, Analyst, 2012, 137, 2183 RSC.
- S. Radhakrishnan, K. Krishnamoorthy, C. Sekar, J. Wilson and S. J. Kim, Appl. Catal., B, 2014, 148, 22 CrossRef PubMed.
- B. Kumar Jena and C. Retna Raj, Anal. Chem., 2008, 80, 4836 CrossRef PubMed.
- A. Chen, Can. J. Chem., 2014, 92, 581 CrossRef CAS.
- P. Benvenuto, A. K. M. Kafi and A. Chen, J. Electroanal. Chem., 2009, 627, 76 CrossRef CAS PubMed.
- A. K. M. Kafi, G. Wu and A. Chen, Biosens. Bioelectron., 2008, 24, 566 CrossRef CAS PubMed.
- D. Reyter, D. Bélanger and L. Roué, J. Electroanal. Chem., 2008, 622, 64 CrossRef CAS PubMed.
- A. Ahmadalinezhad and A. Chen, Biosens. Bioelectron., 2011, 26, 4508 CrossRef CAS PubMed.
- W. Jin, G. Wu and A. Chen, Analyst, 2014, 139, 235 RSC.
- X. Chen, W. Liu, L. Tang, J. Wang, H. Pan and M. Du, Mater. Sci. Eng., C, 2014, 34, 304 CrossRef CAS PubMed.
- E. Sennik, N. Kilinc and Z. Z. Ozturk, J. Alloys Compd., 2014, 616, 89 CrossRef CAS PubMed.
- E. Buhleier, W. Wehner and F. Vogtle, Synthesis, 1978, 1978, 155 CrossRef.
- R. M. Crooks, M. Zhao, L. Sun, V. Chechik and L. K. Yeung, Acc. Chem. Res., 2000, 34, 181 CrossRef PubMed.
- M. Hasanzadeh, N. Shadjou, M. Eskandani, J. Soleymani, F. Jafari and M. de la Guardia, TrAC, Trends Anal. Chem., 2014, 53, 137 CrossRef CAS PubMed.
- P. P. Fang, O. Buriez, E. Labbé, Z. Q. Tian and C. Amatore, J. Electroanal. Chem., 2011, 659, 76 CrossRef CAS PubMed.
- D. Ning, H. Zhang and J. Zheng, J. Electroanal. Chem., 2014, 717, 29 CrossRef PubMed.
- A. Siriviriyanun, T. Imae and N. Nagatani, Anal. Biochem., 2013, 443, 169 CrossRef CAS PubMed.
- H. Yoon, Nanomaterials, 2013, 3, 524 CrossRef CAS PubMed.
- W.-K. Oh, O. S. Kwon and J. Jang, Polym. Rev., 2013, 53, 407 CrossRef CAS.
- J. Kirsch, C. Siltanen, Q. Zhou, A. Revzin and A. Simonian, Chem. Soc. Rev., 2013, 42, 8733 RSC.
- H. J. Salavagione, A. M. Diez-Pascual, E. Lazaro, S. Vera and M. A. Gomez-Fatou, J. Mater. Chem. A, 2014, 2, 14289 CAS.
- M. Campas, D. Garibo and B. Prieto-Simon, Analyst, 2012, 137, 1055 RSC.
- J. P. Metters, R. O. Kadara and C. E. Banks, Analyst, 2012, 137, 896 RSC.
- Z. Xiao-hong, S. Bao-dong, S. Han-chang, L. Lan-hua, G. Hong-li and H. Miao, Sens. Actuators, B, 2014, 198, 150 CrossRef PubMed.
- F. A. Esteve-Turrillas and A. Abad-Fuentes, Biosens. Bioelectron., 2013, 41, 12 CrossRef CAS PubMed.
- J. K. Fawell and G. Stanfield, in Pollution: Causes, Effects and Control, ed. R. M. Harrison, RSC, 2001, vol. 4, pp. 59–81 Search PubMed.
- An Introduction to Pollution Science, ed. R. M. Harrison, RSC, 2006, pp. 77–121 Search PubMed.
- T. F. McGrath, C. T. Elliott and T. L. Fodey, Anal. Bioanal. Chem., 2012, 403, 75 CrossRef CAS PubMed.
- S. Viswanathan, H. Radecka and J. Radecki, Monatsh. Chem., 2009, 140, 891 CrossRef CAS.
- S. J. Kim, K. V. Gobi, H. Iwasaka, H. Tanaka and N. Miura, Biosens. Bioelectron., 2007, 23, 701 CrossRef CAS PubMed.
- T. McGrath, A. Baxter, J. Ferguson, S. Haughey and P. Bjurling, Anal. Chim. Acta, 2005, 529, 123 CrossRef CAS PubMed.
- J. Gong, T. Liu, X. Wang, X. Hu and L. Zhang, Environ. Sci. Technol., 2011, 45, 6181 CrossRef CAS PubMed.
- G. Aragay, J. Pons and A. Merkoçi, Chem. Rev., 2011, 111, 3433 CrossRef CAS PubMed.
- F. Fu and Q. Wang, J. Environ. Manage., 2011, 92, 407 CrossRef CAS PubMed.
- H. H. Harris, I. J. Pickering and G. N. George, Science, 2003, 301, 1203 CrossRef CAS PubMed.
- I. Onyido, A. R. Norris and E. Buncel, Chem. Rev., 2004, 104, 5911 CrossRef CAS PubMed.
- Y. Zhang, G. M. Zeng, L. Tang, Y. P. Li, Z. M. Chen and G. H. Huang, RSC Adv., 2014, 4, 18485 RSC.
- Z. Y. Kong, J. F. Wei, Q. J. Fan, Y. H. Li, K. Y. Zhao, H. T. Guo and C. M. Wei, Anal. Methods, 2013, 5, 4905 RSC.
- X. Gong, Y. Bi, Y. Zhao, G. Liu and W. Y. Teoh, RSC Adv., 2014, 4, 24653 RSC.
- P. K. Aneesh, S. R. Nambiar, T. P. Rao and A. Ajayaghosh, Phys. Chem. Chem. Phys., 2014, 16, 8529 RSC.
- L. E. Eary and D. Rai, Environ. Sci. Technol., 1987, 21, 1187 CrossRef.
- L. McNeill, J. McLean, M. Edwards and J. Parks, in State of the Science of Hexavalent Chromium in Drinking Water, Water Research Foundation, 2012 Search PubMed.
- C. M. Welch, O. Nekrassova and R. G. Compton, Talanta, 2005, 65, 74 CAS.
- A. Deep, A. L. Sharma, S. K. Tuteja and A. K. Paul, J. Hazard. Mater., 2014, 278, 559 CrossRef CAS PubMed.
- M. Rajkumar, S. Thiagarajan and S. M. Chen, Int. J. Electrochem. Sci., 2011, 6, 3164 CAS.
- L. Pujol, D. Evrard, K. Groenen-Serrano, M. Freyssinier, A. Ruffien-Cizsak and P. Gros, Front. Chem., 2014, 2, 19 Search PubMed.
- A. Safavi and E. Farjami, Anal. Chim. Acta, 2011, 688, 43 CrossRef CAS PubMed.
- X. Liu, C. Sun, H. Wu, Y. Zhang, J. Jiang, G. Shen and R. Yu, Electroanalysis, 2010, 22, 2110 CrossRef CAS.
- J. Gong, T. Zhou, D. Song, L. Zhang and X. Hu, Anal. Chem., 2009, 82, 567 CrossRef PubMed.
- S. Tang, P. Tong, W. Lu, J. Chen, Z. Yan and L. Zhang, Biosens. Bioelectron., 2014, 59, 1 CrossRef CAS PubMed.
- S. Sadeghi and A. Garmroodi, Mater. Sci. Eng., C, 2013, 33, 4972 CrossRef CAS PubMed.
- Y. Lian, M. Yuan and H. Zhao, Fullerenes, Nanotubes, Carbon Nanostruct., 2014, 22, 510 CrossRef CAS.
- A. Afkhami, H. Ghaedi, T. Madrakian and M. Rezaeivala, Electrochim. Acta, 2013, 89, 377 CrossRef CAS PubMed.
- Z. Wang, H. Wang, Z. Zhang, X. Yang and G. Liu, Electrochim. Acta, 2014, 120, 140 CrossRef CAS PubMed.
- R. A. Dar, N. G. Khare, D. P. Cole, S. P. Karna and A. K. Srivastava, RSC Adv., 2014, 4, 14432 RSC.
- D. Wang, Y. Zhao, H. Jin, J. Zhuang, W. Zhang, S. Wang and J. Wang, ACS Appl. Mater. Interfaces, 2013, 5, 5733 CAS.
- H. Cui, W. Yang, X. Li, H. Zhao and Z. Yuan, Anal. Methods, 2012, 4, 4176 RSC.
- M. Noroozifar, M. Khorasani-Motlagh and A. Taheri, J. Hazard. Mater., 2011, 185, 255 CrossRef CAS PubMed.
- I. A. Wolff and A. E. Wasserman, Science, 1972, 177, 15 CAS.
- X.-H. Pham, C. A. Li, K. N. Han, B.-C. Huynh-Nguyen, T.-H. Le, E. Ko, J. H. Kim and G. H. Seong, Sens. Actuators, B, 2014, 193, 815 CrossRef CAS PubMed.
- D. Zhang, H. Ma, Y. Chen, H. Pang and Y. Yu, Anal. Chim. Acta, 2013, 792, 35 CrossRef CAS PubMed.
- S. M. da Silva and L. H. Mazo, Electroanalysis, 1998, 10, 1200 CrossRef CAS.
- P. Miao, M. Shen, L. Ning, G. Chen and Y. Yin, Anal. Bioanal. Chem., 2011, 399, 2407 CrossRef CAS PubMed.
- A. Afkhami, F. Soltani-Felehgari, T. Madrakian and H. Ghaedi, Biosens. Bioelectron., 2014, 51, 379 CrossRef CAS PubMed.
- T. Madasamy, M. Pandiaraj, M. Balamurugan, K. Bhargava, N. K. Sethy and C. Karunakaran, Biosens. Bioelectron., 2014, 52, 209 CrossRef CAS PubMed.
- B. A. Molinero-Abad, M. A. N. Alonso-Lomillo, O. Dominguez-Renedo and M. J. Arcos-Martinez, Anal. Chim. Acta, 2014, 812, 41 CrossRef CAS PubMed.
- M. Amatatongchai, W. Sroysee, S. Chairam and D. Nacapricha, Talanta, 2014 DOI:10.1016/j.talanta.2014.07.055.
- G. Maduraiveeran and R. Ramaraj, Electrochem. Commun., 2007, 9, 2051 CrossRef CAS PubMed.
- S. Liu, B. Yu, F. Li, Y. Ji and T. Zhang, Electrochim. Acta, 2014, 141, 161 CrossRef CAS PubMed.
- D. d. Zhou, L. Ding, H. Cui, H. An, J. p. Zhai and Q. Li, Chem. Eng. J., 2012, 200, 32 CrossRef PubMed.
- H. S. Weinberg, C. A. Delcomyn and V. Unnam, Environ. Sci. Technol., 2003, 37, 3104 CrossRef CAS.
- L. Xie and C. Shang, Water Sci. Technol.: Water Supply, 2006, 6, 131 CrossRef CAS.
- A. Salimi, H. MamKhezri, R. Hallaj and S. Zandi, Electrochim. Acta, 2007, 52, 6097 CrossRef CAS PubMed.
- P. Muthukumar and S. Abraham John, J. Colloid Interface Sci., 2014, 421, 78 CrossRef CAS PubMed.
- P. Li, Y. Ding, A. Wang, L. Zhou, S. Wei, Y. Zhou, Y. Tang, Y. Chen, C. Cai and T. Lu, ACS Appl. Mater. Interfaces, 2013, 5, 2255 CAS.
- A. Afkhami, F. Soltani-Felehgari, T. Madrakian and H. Ghaedi, Biosens. Bioelectron., 2014, 51, 379 CrossRef CAS PubMed.
- S. Radhakrishnan, K. Krishnamoorthy, C. Sekar, J. Wilson and S. J. Kim, Appl. Catal., B, 2014, 1489, 22 CrossRef PubMed.
- R. Rawal and C. S. Pundir, Int. J. Biol. Macromol., 2012, 51, 449 CrossRef CAS PubMed.
- S. Devaramani and P. Malingappa, Electrochim. Acta, 2012, 85, 579 CrossRef CAS PubMed.
- A. A. Ensafi, H. Karimi-Maleh and M. Keyvanfard, Int. J. Environ. Anal. Chem., 2013, 93, 650 CrossRef CAS.
- L. Wang, P. Hu, X. Deng, F. Wang and Z. Chen, Biosens. Bioelectron., 2013, 50, 57 CrossRef CAS PubMed.
- D. Zheng, X. Liu, D. Zhou and S. Hu, Microchim. Acta, 2012, 176, 49 CrossRef CAS.
- S. Jayabal, P. Viswanathan and R. Ramaraj, RSC Adv., 2014, 4, 33541 RSC.
- R. Gatermann, H. Hühnerfuss, G. Rimkus, M. Wolf and S. Franke, Mar. Pollut. Bull., 1995, 30, 221 CrossRef CAS.
- F. Karim and A. N. M. Fakhruddin, Rev. Environ. Sci. Biotechnol., 2012, 11, 261 CrossRef CAS PubMed.
- U.S. EPA Title 40, Chapter 1, Part 141, National Primary Drinking Water Regulation, http://www.law.cornell.edu/cfr/text/40/141.11, accessed 17 August, 2014.
- Drinking Water Directive 80/778/EEC, Commission of the European Communities, 1980.
- T. C. Canevari, P. A. Raymundo-Pereira, R. Landers and S. A. S. Machado, Eur. J. Inorg. Chem., 2013, 5746 CrossRef CAS.
- J. S. Lee, S. G. Kim, J. Jun, D. H. Shin and J. Jang, Adv. Funct. Mater., 2014, 24, 6145 CrossRef CAS.
- A. K. M. Kafi and A. Chen, Talanta, 2009, 79, 97 CrossRef CAS PubMed.
- S. R. Hernandez, S. V. Kergaravat and M. I. Pividori, Talanta, 2013, 106, 399 CrossRef CAS PubMed.
- Y. Qu, M. Ma, Z. Wang, G. Zhan, B. Li, X. Wang, H. Fang, H. Zhang and C. Li, Biosens. Bioelectron., 2013, 44, 85 CrossRef CAS PubMed.
- J. A. Rather, S. Pilehvar and K. D. Wael, Analyst, 2013, 138, 204 RSC.
- J. Dobes, O. Zitka, J. Sochor, B. Ruttkay-Nedecky, P. Babula, M. Beklova, J. Kynicky, J. Hubalek, B. Klejdus and R. Kizek, Int. J. Electrochem. Sci., 2013, 8, 4520 CAS.
- K. Giribabu, R. Suresh, R. Manigandan, S. Munusamy, S. P. Kumar, S. Muthamizh and V. Narayanan, Analyst, 2013, 138, 5811 RSC.
- X. Bai, X. Huang, X. Zhang, Z. Hu, C. Wang, Q. Qin and Q. Zhang, RSC Adv., 2014, 4, 13461 RSC.
- D. Yuan, S. Chen, R. Yuan, J. Zhang and W. Zhang, Analyst, 2013, 138, 6001 RSC.
- Y. L. Yang, B. Unnikrishnan and S. M. Chen, Int. J. Electrochem. Sci., 2011, 6, 2902 Search PubMed.
- C. Yu, L. Gou, X. Zhou, N. Bao and H. Gu, Electrochim. Acta, 2011, 56, 9056 CrossRef CAS PubMed.
- C. Yang, Y. Chai, R. Yuan, W. Xu and S. Chen, Anal. Methods, 2013, 5, 666 RSC.
- B. T. P. Quynh, J. Y. Byun and S. H. Kim, Sens. Actuators, B, 2014, 193, 1 CrossRef CAS PubMed.
- B. Kaur and R. Srivastava, Electrochim. Acta, 2014, 141, 61 CrossRef CAS PubMed.
- S. Pilehvar, J. Ahmad Rather, F. Dardenne, J. Robbens, R. Blust and K. De Wael, Biosens. Bioelectron., 2014, 54, 78 CrossRef CAS PubMed.
- D. Yuan, S. Chen, F. Hu, C. Wang and R. Yuan, Sens. Actuators, B, 2012, 168, 193 CrossRef CAS PubMed.
- H. S. Han, J. M. You, H. Seol, H. Jeong and S. Jeon, Sens. Actuators, B, 2014, 194, 460 CrossRef CAS PubMed.
- J. Du, L. Ma, D. Shan, Y. Fan, L. Zhang, L. Wang and X. Lu, J. Electroanal. Chem., 2014, 722, 38 CrossRef PubMed.
- E. Cevik, M. Senel, A. Baykal and M. F. Abasiyanik, Sens. Actuators, B, 2012, 173, 396 CrossRef CAS PubMed.
- H. Wu, J. Hu, H. Li and H. Li, Sens. Actuators, B, 2013, 182, 802 CrossRef CAS PubMed.
- W. Tang, M. Zhang, W. Li and X. Zeng, Talanta, 2014, 127, 262 CrossRef CAS PubMed.
- J. Zolgharnein, T. Shariatmanesh and A. Babaei, Sens. Actuators, B, 2013, 186, 536 CrossRef CAS PubMed.
- L. Zhang, Y. P. Wen, Y. Y. Yao, Z. F. Wang, X. M. Duan and J. K. Xu, Chin. Chem. Lett., 2014, 25, 517 CrossRef CAS PubMed.
- C. X. Yuan, Y. R. Fan, Z. Tao, H. X. Guo, J. X. Zhang, Y. L. Wang, D. L. Shan and X. Q. Lu, Biosens. Bioelectron., 2014, 58, 85 CrossRef CAS PubMed.
- T. Pradeep, Anshup and M. S. Bootharaju, Nanotechnology Applications for Clean Water, 2nd edn, 2014, ch. 16, pp. 241–270 Search PubMed.
- M. Tankiewicz, J. Fenik and M. Biziuk, TrAC, Trends Anal. Chem., 2010, 29, 1050 CrossRef CAS PubMed.
- S. Liu, Z. Zheng and X. Li, Anal. Bioanal. Chem., 2013, 405, 63 CrossRef CAS PubMed.
- B. Perez-Lopez and A. Merkoc, Trends Food Sci. Technol., 2011, 22, 625 CrossRef CAS PubMed.
- G. Aragay, F. Pino and A. Merkoc, Chem. Rev., 2012, 112, 5317 CrossRef CAS PubMed.
- G. G. Guilbault, M. H. Sadar, S. S. Kuan and D. Casey, Anal. Chim. Acta, 1970, 52, 75 CrossRef CAS.
- V. Dhull, A. Gahlaut, N. Dilbaghi and V. Hooda, Biochem. Res. Int., 2013, 1 CrossRef PubMed.
- J. Ding, H. Zhang, F. Jia, W. Qin and D. Du, Sens. Actuators, B, 2014, 199, 284 CrossRef CAS PubMed.
- I. Cesarino, F. C. Moraes, M. R. Lanza and S. A. Machado, Food Chem., 2012, 135, 873 CrossRef CAS PubMed.
- L. Agüí, C. Peña-Farfal, P. Yáñez-Sedeño and J. M. Pingarrón, Electrochim. Acta, 2007, 52, 7946 CrossRef PubMed.
- G. F. Grawe, T. R. de Oliveira, E. de Andrade Narciso, S. K. Moccelini, A. J. Terezo, M. A. Soares and M. Castilho, Biosens. Bioelectron., 2015, 63, 407 CrossRef CAS PubMed.
- G. Liu, W. Guo and D. Song, Biosens. Bioelectron., 2014, 52, 360 CrossRef CAS PubMed.
- D. Huo, Q. Li, Y. Zhang, C. Hou and Y. Lei, Sens. Actuators, B, 2014, 199, 410 CrossRef CAS PubMed.
- Y. Braham, H. Barhoumi and A. Maaref, Anal. Methods, 2013, 5, 4898 RSC.
- X. Tang, T. Zhang, B. Liang, D. Han, L. Zeng, C. Zheng, T. Li, M. Wei and A. Liu, Biosens. Bioelectron., 2014, 60, 137 CrossRef CAS PubMed.
- T. Zhang, L. Zeng, L. Han, T. Li, C. Zheng, M. Wei and A. Liu, Anal. Chim. Acta, 2014, 822, 23 CrossRef CAS PubMed.
- Y. Li, M. Xu, P. Li, J. Dong and S. Ai, Anal. Methods, 2014, 6, 2157 RSC.
- J. Dong, X. Wang, F. Qiao, P. Liu and S. Ai, Sens. Actuators, B, 2013, 186, 774 CrossRef CAS PubMed.
- J. Gong, X. Miao, H. Wan and D. Song, Sens. Actuators, B, 2012, 162, 341 CrossRef CAS PubMed.
- S. Wu, X. Lan, L. Cui, L. Zhang, S. Tao, H. Wang, M. Han, Z. Liu and C. Meng, Anal. Chim. Acta, 2011, 699, 170 CrossRef CAS PubMed.
- S. Ebrahim, R. El-Raey, A. Hefnawy, H. Ibrahim, M. Soliman and T. M. Abdel-Fattah, Synth. Met., 2014, 190, 13 CrossRef CAS PubMed.
- M. Wang, J. Huang, M. Wang, D. Zhang and J. Chen, Food Chem., 2014, 151, 191 CrossRef CAS PubMed.
- T. Jeyapragasam and R. Saraswathi, Sens. Actuators, B, 2014, 191, 681 CrossRef CAS PubMed.
- P. A. D'Agostino and C. L. Chenier, Technical Report March 2006, DRDC Suffield TR 2006-022.
- S. Royo, R. Martinez-Manez, F. Sancenon, A. M. Costero, M. Parra and S. Gil, Chem. Commun., 2007, 4839 RSC.
- R. M. Black, J. Chromatogr. B: Anal. Technol. Biomed. Life Sci., 2010, 878, 1207 CrossRef CAS PubMed.
- I. Bhowmick and Neelam, Analyst, 2014, 139, 4154 CAS.
- F. Arduini, D. Neagu, S. Dall'Oglio, D. Moscone and G. Palleschi, Electroanalysis, 2012, 24, 581 CrossRef CAS.
- P. A. Baker, M. N. Goltz, A. M. Schrand, D. Y. Yoon and D. S. Kim, Biosens. Bioelectron., 2014, 61, 119 CrossRef CAS PubMed.
- J. S. Lee, D. H. Shin, J. Jun and J. Jang, ACS Nano, 2013, 7, 10139 CrossRef CAS PubMed.
- F. Arduini, A. Amine, D. Moscone, F. Ricci and G. Palleschi, Anal. Bioanal. Chem., 2007, 388, 1049 CrossRef CAS PubMed.
- G. Liu and Y. Lin, Anal. Chem., 2006, 78, 835 CrossRef CAS PubMed.
- S. P. Sharma, L. N. S. Tomar, J. Acharya, A. Chaturvedi, M. V. S. Suryanarayan and R. Jain, Sens. Actuators, B, 2012, 166, 616 CrossRef PubMed.
- S. Wu, F. Huang, X. Lan, X. Wang, J. Wang and C. Meng, Sens. Actuators, B, 2013, 177, 724 CrossRef CAS PubMed.
- Y. Yang, H. Tu, A. Zhang, D. Du and Y. J. Lin, J. Mater. Chem., 2012, 22, 4977 RSC.
- P. Noyrod, O. Chailapakul, W. Wonsawat and S. Chuanuwatanakul, J. Electroanal. Chem., 2014, 719, 54 CrossRef CAS PubMed.
|
This journal is © The Royal Society of Chemistry 2014 |