DOI:
10.1039/C4RA10128F
(Paper)
RSC Adv., 2014,
4, 50331-50337
Visible light driven photocatalytic oxidation of thiols to disulfides using iron phthalocyanine immobilized on graphene oxide as a catalyst under alkali free conditions
Received
3rd March 2014
, Accepted 29th September 2014
First published on 30th September 2014
Abstract
The present paper describes the synthesis of graphene oxide immobilized iron phthalocyanine (FePc) for the photocatalytic oxidation of thiols to disulfides under alkaline free conditions. Iron phthalocyanine tetrasulfonamide was immobilized on carboxylated graphene oxide supports via covalent attachment. The loading of FePc on GO nanosheets was confirmed by FTIR, Raman, ICP-AES, UV-Vis and elemental analyses. The synthesized catalyst was found to be highly efficient for the photo-oxidation of thiols to disulfides in aqueous medium using molecular oxygen as oxidant under visible light irradiation. The identification of photo-oxidation products and their quantitative determination was done using GC-MS. After completion of the reaction, the catalyst was easily recovered by filtration and reused for several runs without loss in activity and no leaching was observed during the reaction.
Introduction
The use of visible-light photoredox catalysis to initiate organic transformations is a fascinating approach towards the development of green and sustainable chemistry.1,2 In this regard, photoredox catalysis using metal complexes based on ruthenium or iridium has gained particular interest.3–5 These complexes are advantageous with respect to their stability and higher activity, however, the scarcity and toxic nature of these metals makes their utility limited for practical applications. Therefore, we have focused our attention on the use of iron especially iron phthalocyanine which is easily accessible, inexpensive and non-toxic in nature. Further, immobilization of iron phthalocyanine to solid supports provides an elegant way to recover the catalyst for its recyclability and reusability.6–8
Oxidation of thiols to disulfides is an important transformation from synthetic, biological and industrial viewpoints. In addition, disulfides find extensive applications as protecting groups in synthesis of pharmaceutical, bioactive compounds and as vulcanizing agents for rubbers.9,10 Besides the conventional oxidants such as manganese dioxide, dichromates, chlorochromates, etc., a number of catalytic methods including cobalt, manganese, copper, vanadium, cerium, and nickel based catalysts have been reported for the aerobic oxidation of thiols into disulfides.9,11–13 Among the known catalysts, cobalt phthalocyanine complexes have been studied extensively and utilized in both the petroleum industry and the large-scale syntheses of disulfides.14–16 Besides the disadvantage of their relatively high cost and homogeneous nature, these reactions require strongly alkaline conditions to be efficient. In this regard, many studies have been directed to the immobilization of cobalt phthalocyanines on solid supports particularly to solid basic materials, such as magnesium containing oxides and surface-modified carbons.17–21 Since solid basic catalysts are usually easy to deactivate, therefore it is desirable to develop a simple heterogeneous catalyst for the alkaline free oxidation of thiols to disulfides.12,13 Graphene oxide due to its outstanding surface properties has emerged to be a matter of choice for supporting various homogeneous catalysts for various applications. In this regard, Zhu et al.22 reported the immobilization of zinc phthalocyanine to graphene oxide for broadband optical limiting applications. Zhang and coworkers23 have grafted phthalocyanine complex to graphene oxide via π–π interaction. Xiao et al.24 reported the immobilization of Ru(bpy)2(py)Cl dye to pyridine functionalized graphene for the visible light mediated water splitting reaction. Similarly, Min et al.25 and Mou et al.26 reported Eosin-Y sensitized reduced graphene oxide (RGO) for the hydrogen evolution through water splitting reaction. Furthermore, to the best of our knowledge photocatalytic oxidation of thiols to disulfides under visible light irradiation is rarely known in the literature. In the present work we have used graphene oxide (GO) as a support for immobilization of iron phthalocyanine complex via covalent attachment. The synthesized heterogeneous catalyst was used for the photocatalytic oxidation of thiols to disulfides using molecular as oxidant in visible light under alkaline free conditions (Scheme 1).
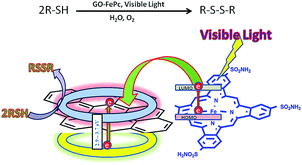 |
| Scheme 1 Photocatalytic oxidation of thiols to disulfides. | |
Synthesis and characterization of the catalyst
Graphene oxide (GO) was prepared from oxidation of graphite under harsh conditions by following modified Hummers method.27 Prior to the immobilization, the GO was treated with chloroacetic acid to convert the OH, epoxy groups into –COOH groups, which were subsequently used for the immobilization of iron phthalocyanine tetrasulfonamide (FePc-SO2NH2) via sulfonamide linkages. Covalent attachment of the FePc to graphene oxide support provided higher loading as well as stability of the catalyst towards leaching. The schematic representation of the synthesis of iron phthalocyanine immobilized to graphene oxide (GO–FePc) is shown in Scheme 2.
 |
| Scheme 2 Synthesis of iron phthalocyanine immobilized to graphene oxide support. | |
BET surface area, pore diameter, pore volume and other surface properties of GO and GO–FePc was measured with the help of nitrogen adsorption desorption isotherm at 77 K. Adsorption desorption isotherm of GO and GO–FePc is shown in Fig 1a and b respectively. The BET surface area (SBET), and total pore volume of GO was found to be 87.247 m2 g−1 and 0.1212 cm3 g−1 respectively. Total pore diameter of GO was found to be 5.5566 nm that was in conformity to mesoporous nature.28 After immobilization of FePc, the surface area of GO was decreased to 14.32 m2 g−1 that was presumed to the successful attachment of FePc at the surface of GO. Total pore volume and mean pore diameter of GO–FePc was found to be 0.08 cm3 g−1 and 28.161 nm respectively.
 |
| Fig. 1 Adsorption desorption curve of; (a) GO; (b) GO–FePc. | |
XRD patterns are used to study the changes in structure (Fig. 2). XRD diffractogram of GO reveals a characteristic peak at 2θ value 11° due to the diffraction from 002 plane29 (Fig. 2a). Compared with GO, the XRD pattern of the GO–FePc showed a new peak at 2θ = 26.6°, corresponding to the graphite (002) planes, indicating that the attachment of FePc was taken place to the GO support.30,31 Furthermore, amorphous nature of the material was confirmed by XRD (Fig. 2).
 |
| Fig. 2 XRD Pattern: (a) GO; (b) GO–FePc. | |
The surface morphologies of GO and GO–FePc were investigated via scanning electron microscopy (SEM) and transmission electron microscopy (TEM). As shown in Fig. 3a, there are large flakes of GO with macroscopic wrinkling. Compared with GO sheets, the SEM image of GO–FePc (Fig. 3b) exhibited an agglomerated layered structure incorporating phthalocyanine complex moieties between the sheets. Furthermore, the presence of iron in EDX analysis of GO–FePc showed the successful attachment of FePc to GO support (Fig. 3d).
 |
| Fig. 3 SEM images of (a) GO; (b) GO–FePc; (c) EDX of GO; (d) EDX of GO–FePc. | |
TEM image of GO, GO–COOH and GO–FePc showed twisted and crumpled nanosheets (Fig. 4a–c), which are in agglomerated phase. The dark spots in the TEM image of GO–COOH (Fig. 4b) was probably due to the deformated nanosheets of GO, which might be generated during the conversion of epoxides to carboxylic acid groups. In the TEM image of GO–FePc (Fig. 4c) the appearance of broad dark spots were assumed due to some degree of sheet folding resulting from the π–π interaction between FePc and GO.32,33 This clearly illustrate the successful attachment of FePc molecules on GO surface. Selected area electron diffraction pattern of GO–FePc (Fig. 4d) shows that material was amorphous in nature.
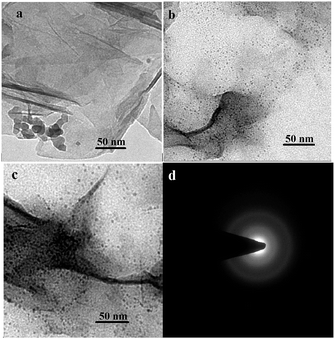 |
| Fig. 4 TEM images of (a) GO; (b) GO–COOH; (c) GO–FePc; (d) SAED pattern of GO–FePc. | |
Fig. 5 shows the FT-IR spectra of the GO, FePc(SO2NH2)4 and GO–FePc. The pure support GO (Fig. 5a) exhibited bands at 3408, 1721, 1620, 1220, and 1058 cm−1 due to the stretching mode of O–H, C
O, C
C, C–O and C–O–C bands, respectively.34,35 After the immobilization, the peak at 1733 cm−1 was reduced and a new peak appeared at 1710 cm−1 which is attributed to sulfonamide (–SO2NH–CO–) groups. This band confirmed the covalent attachment of FePc to GO via sulfonamide bond formations.36 Furthermore, the presence of phthalocyanine peaks at 1386 and 1035 cm−1 suggested the successful grafting of FePc to GO support.
 |
| Fig. 5 FTIR spectra of (a) FePc(SO2NH2)4; (b) GO and (c) GO–FePc. | |
Covalent attachment of FePc(SO2NH2)4 to GO–COOH support was further confirmed by Raman spectroscopy (Fig. 6). Two characteristic peaks at about 1351 (D-band) and 1599 (G-band) cm−1 were appeared in the Raman spectrum of GO.37 The intensity ratio of these bands is frequently used for indicating the level of chemical modification of the graphitic carbon sample. In contrast to GO, both D and G bands were found to be shifted towards lower wavenumbers in GO–FePc, probably due to the electron donating nature of π–π bonded FePc molecules. The D- to G-band intensity ratios (ID/IG) was found to be decreased from 0.98 for GO to 0.78 for GO–FePc.
 |
| Fig. 6 Raman spectra of (a) GO and (b) GO–FePc. | |
UV-Vis absorption spectra of FePc(SO2NH2)4 in DMF showed two characteristic broad peaks at 350 nm (Soret band) and 690 nm (Q band) respectively (Fig. 7a). Absorption spectra of GO Fig. 7b indicated the characteristics absorption band near to 230 nm due to π → π* transition of aromatic ring electrons and a small hump near to 300 nm due to n → π* of carbonyl groups.38 After immobilization of FePc to GO, the absorbance was shifted towards longer wave length 310 nm and a peak at 650 nm due to Q band absorption of FePc was observed (Fig. 7c). It further confirmed the successful attachment of FePc to the GO support.
 |
| Fig. 7 UV-Vis absorption spectra of (a) FePc(SO2NH2)4; (b) GO; (c) GO–FePc. | |
Thermal stability of the developed photocatalyst GO–FePc was determined by TGA (Fig. 8). Thermogram of GO (Fig. 8a) showed initial weight loss near 95–100 °C, evidently owing to evaporation of water molecules which are held in the material. The second significant weight loss approx 50% was observed in the range of 160–350 °C, is due to thermal decomposition of oxygen carrying functionalities. Thermogram of GO–FePc (Fig. 8b) catalyst illustrated an exothermic major weight loss over a wide range of temperature (300–500 °C) due to the slow decomposition of the phthalocyanine moieties, indicating that the catalyst is sufficient thermally stable. Finally the weight loss starting from 650 °C was mainly due to loss of carbonaceous material. The absence of weight loss in the temperature range from 150 °C to 300 °C clearly indicated that the oxygen carrying functionalities located on graphene oxide support was utilized in sulfonamide bond formation with iron phthalocyanine during the immobilization process.
 |
| Fig. 8 TGA pattern of; (a) GO; (b) GO–FePc. | |
The photocatalytic oxidation of thiols
The photocatalytic activity of the synthesized graphene oxide immobilized iron phthalocyanine (GO–FePc) was tested for the oxidation of various aliphatic and aromatic thiols to the corresponding disulfides using molecular oxygen as oxidant in aqueous alkaline free medium under visible light using a household white LED (20 W) (Scheme 1). The reactions were followed by GC and the conversion/selectivity of the product was confirmed by GC-MS. The results of these experiments are summarized in Table 1. The selectivities for corresponding disulfides were virtually 100% with no even trace amounts of other products being detected by GC (Table 1). As shown in Table 1, aromatic mercaptans were found to be less reactive and provided poor product yields than those of aliphatic mercaptans. In the case of aliphatic mercaptans the reactivity decreases with the increase in chain length and accordingly the mercaptans with longer chain length require more reaction time.
Table 1 Photocatalytic oxidation of thiols to disulfidesa
Entry |
Thiol |
Disulfide |
T (h) |
Conv.b (%) |
Yieldc (%) |
Reaction conditions: thiol (2 mmol), catalyst (1 mol%), under oxygen atmosphere at room temperature in visible light. Determined by GC. Isolated yield. |
1. |
 |
 |
1.0 |
99 |
98 |
2. |
 |
 |
1.0 |
99 |
97 |
3. |
 |
 |
1.0 |
98 |
97 |
4. |
 |
 |
1.5 |
95 |
92 |
5. |
 |
 |
2.5 |
94 |
92 |
6. |
 |
 |
3.5 |
94 |
91 |
7. |
 |
 |
4.0 |
87 |
84 |
8. |
 |
 |
4.0 |
85 |
82 |
9. |
 |
 |
4.5 |
84 |
80 |
10. |
 |
 |
4.5 |
78 |
75 |
The blank experiments were carried out by choosing dodecane thiol (C12) as the representative substrate, the results of the blank experiments are plotted in Fig. 9. As shown that the reaction did not proceed in the absence of photocatalyst under identical reaction conditions. The oxidation was found to be very slow while using graphene oxide alone as catalyst and yielded only 20% yield of the disulfide under visible light illumination. However, the oxidation was found to be completed within 3.5 h providing 100% conversion in the presence of GO–FePc as catalyst under visible light.
 |
| Fig. 9 Results of experiments using dodecane thiol as model substrate (a) without photocatalyst; (b) using FePc in dark; (c) using GO–FePc in dark; (d) using GO in visible light; (e) using FePc in visible light; (f) using GO–FePc in visible light. | |
Furthermore, in the dark, the oxidation of dodecane thiol using GO–FePc as a photocatalyst was found to be virtually slow and yielded corresponding disulfide (22%) as low as in blank experiment using GO as catalyst. Furthermore, the oxidation of dodecane thiol provided 62% yield of the corresponding disulfide when homogeneous FePc was used as photocatalyst under similar experimental conditions. Blank reaction using FePc as catalyst in dark gave lower oxidation of dodecane thiol and afforded very low yield (30%) of corresponding disulfide. These results confirmed that the reactions were assisted by visible light and graphene oxide enhanced the photocatalytic activity of the catalyst. The quantum yield for conversion of dodecane thiol to corresponding disulfides using GO–FePc catalyst was found to be 0.27 mole per Einstein.
After the photocatalytic reaction, the catalyst was recovered by centrifugation and recycled for the subsequent experiments. The recyclability of the catalyst was tested for oxidation of dodecane thiol for seven subsequent runs (Fig. 10). As shown that the recovered catalyst provided almost similar conversion and yield of the desired product in all cases. These results confirmed the efficient recycling of the catalyst for oxidation of thiols. Furthermore, ICP-AES analysis of recovered catalyst after seven runs was found to be 2.18 wt% which was nearly similar to the fresh catalyst (2.23 wt%), indicating that there was no appreciable leaching had occurred during the reaction.
 |
| Fig. 10 Recycling experiment for seven run using dodecane thiol. | |
Moreover, the possible photocatalytic mechanism of the oxidation of thiols has been demonstrated for the better understanding of the reaction.39,40 It is well documented in the literature that when metal phthalocyanine complexes are irradiated in visible light they get excited to singlet state (1MPc)* which then converted to triplet state (3MPc)*. This excited triplet state then interacts with ground state (triplet state) of molecular oxygen to generate excited singlet oxygen. The singlet excited oxygen interacts with thiol to give thiolate radical. Compared with the homogeneous FePc, the GO–FePc showed a remarkably enhanced photocatalytic activity under visible light irradiation. The superior electron-accepting and electron-transporting properties of graphene accelerate the electron transfer from excited FePc to oxygen,41 which promote the activation of thiol to give thiolate radical. In the final stage, coupling of these radicals provided corresponding disulfide as shown in Scheme 3.
 |
| Scheme 3 Possible mechanism of the reaction. | |
Conclusions
We have demonstrated a simple and efficient visible light promoted photocatalytic oxidation of thiols to disulfides selectively using molecular oxygen as oxidant in aqueous medium without using alkaline reagents. Covalent attachment of the FePc(SO2NH2)4 to GO–COOH support via sulfonamide linkages provided higher loading and stability towards leaching of metal/ligand. Furthermore, alkaline free oxidation under mild experimental conditions using visible light makes the developed method feasible for large scale applications. We believe that the developed system will open new avenues for various other applications in synthetic organic chemistry.
Experimental
Materials
Graphite flakes, triethylamine-99%, thiols and iron phthalocyanine (≥80%) were procured from Sigma Aldrich. Potassium permanganate 99.0%, sodium nitrate 99.0%, concentrated sulphuric acid, hydrogen peroxide (30%), hydrochloric acid, chloroacetic acid, thionyl chloride, chlorosulfonic acid-99%, diethyl ether and HPLC grade water, were indented from MERCK India. No further purification was done and all chemicals were used as received. Iron phthalocyanine tetrasulfonamide FePc(SO2NH2)4 complex was synthesized by following the literature procedure.42
Techniques used
Structural morphologies of GO and GO–FePc were determined by using scanning electron microscopy (SEM) (Jeol Model JSM-6340F). Sample for SEM was prepared by depositing aqueous paste on glass slide. While fine structure of samples was determine by high resolution transmission electron microscopy (HR-TEM) Model no. FEI-TecnaiG2 TwinTEM working at an acceleration voltage of 200 kV. Well dispersed aqueous samples were deposited on carbon coated copper grid for TEM analysis. X-ray diffraction pattern of GO and FePc attached to GO was executed using a Bruker D8 Advance diffractometer at 40 kV and 40 mA with Cu Kα radiation (λ = 0.15418 nm). Samples was taken in a glass slide and dried before analysis. Vibrational spectra (FT-IR) of samples were recorded on Perkin Elmer spectrum RX-1 IR spectrophotometer. UV-Visible absorbance for solid samples of GO and GO–FePc was determined on Perkin Elmer lambda-19 UV-VIS-NIR spectrophotometer by using BaSO4 as reference material. Thermal stability of GO and synthesized catalyst was calculated by thermo gravimetric analysis (TGA) with the help of a thermal analyzer TA-SDT Q-600. Samples were analyzed between temperature range from 40 to 900 °C with the heating rate 10 °C min−1 under nitrogen flow. N2 adsorption desorption isotherm, Brunauer–Emmett–Teller (BET) surface area, pore volume, pore diameter and other surface properties of samples were obtained on Micromeritics ASAP2010 working in liquid nitrogen at 77 K. The yield of product during photoreaction was determined by GC-MS (Varian CP-3800). Iron content of catalyst in wt% was determined by inductively coupled plasma atomic emission spectrometer (ICP-AES, DRE, PS-3000UV, Leeman Labs Inc, USA). For preparation of sample 0.01 g of catalyst was digested with conc. HNO3 and heated at 70 °C for 30 min for oxidizing organic component. Finally volume was made up to 10 ml by adding de-ionised water. Elemental composition of GO–FePc was taken on CHNS analyzer.
Synthesis of graphene oxide22
Graphene oxide (GO) was synthesized from graphite flakes using modified Hummers method. In a typical experiment, concentrated H2SO4 (68 ml) was added into a flask containing graphite flakes (2 g) and sodium nitrate (0.75 g) under stirring at 0 °C (ice bath). After that KMnO4 (9.0 g) was added slowly to this mixture and stirred for 5 days. 100 ml diluted H2SO4 (5 wt%) was added to this and heated at 90 °C for 2 h with continuous stirring. Subsequent addition of 30 wt% H2O2 solution (approximately 5.4 ml) followed by stirring for 2 h at room temperature afforded graphene oxide which was collected by centrifugation. The as synthesized material was subsequently washed with H2SO4 (3 wt%), H2O2 (0.5 wt%), HCl (3 wt%) and finally with distilled water.
Synthesis of carboxylated graphene oxide43
Graphene oxide (0.4 g) was dispersed in distilled water (200 ml) with the help of ultrasonication. The obtained suspension was treated with NaOH (2.4 g), chloroacetic acid (2.0 g) and then sonicated for 3 h. This step converted the hydroxyl (–OH) and epoxy groups of GO to carboxylic groups (–COOH). Diluted HCl was added to the suspension containing GO–COOH to achieve the pH neutral. Finally the obtained GO–COOH was collected by centrifugation and washed with distilled water, dried.
Immobilization of FePc(SO2NH2)4 to carboxylated graphene oxide44
Prior to attachment GO–COOH was treated with thionyl chloride for converting it into acid chloride groups. GO–COCl and FePc(SO2NH2)4 complex were added in a round bottomed flask containing DMF and 1 ml triethylamine was added. The resulting suspension was refluxed under N2 atmosphere for 24 h. After being cooled at room temperature, the graphene oxide immobilized iron phthalocyanine (GO–FePc) was separated by centrifugation and washed with ethanol for five times. ICP-AES analysis of the sample provided Fe 2.23 wt% and elemental analysis (CHNS) gave C% 48.97, H% 2.546, N% 4.674, and S% 3.975 respectively.
Typical experimental procedure for oxidation of thiols to disulfides
In a 25 ml round bottomed flask containing distilled water (10 ml) was added GO–FePc catalyst (1 mol%) and thiol (2 mmol). The reaction vessel was sealed and illuminated with 20 watt LED light (Model no. HP-FL-20W-F-Hope LED Opto-Electric Co. Ltd. λ > 400 nm) under oxygen atmosphere. The reaction vessel was 4 cm far away from the light source and intensity at the surface was found to be 70 W m−2 as measured by intensity meter. Samples were collected after every half an hour interval with the help of a syringe. The samples were extracted with diethyl ether before injecting to the GC. Identity of the products was determined by GC-MS.
Acknowledgements
We kindly acknowledge Director, CSIR-IIP for his kind permission to publish these results. PK is thankful to CSIR, New Delhi for his research fellowship under Emeritus Scientist Scheme. Analytical division of the Institute is kindly acknowledged for providing support in analysis of samples. CSIR, New Delhi is kindly acknowledged for financial assistance under XII year projects.
Notes and references
- T. P. Yoon, M. A. Ischay and J. Du, Nat. Chem., 2010, 527–532 CrossRef CAS PubMed.
- J. M. R. Narayanam and C. R. J. Stephenson, Chem. Soc. Rev., 2011, 40, 102–113 RSC.
- C. K. Prier, D. A. Rankic and D. W. C. MacMillan, Chem. Rev., 2013, 113, 5322–5363 CrossRef CAS PubMed.
- J. W. Tucker and C. R. J. Stephenson, J. Org. Chem., 2012, 77, 1617–1622 CrossRef CAS PubMed.
- M. Zhang, C. Chen, W. Ma and J. Zhao, Angew. Chem., 2008, 120, 9876–9879 CrossRef.
- M. Mahyari and A. Shaabani, Appl. Catal., A, 2014, 469, 524–531 CrossRef CAS PubMed.
- M. Pirouzmand, M. M. Amini and N. Safari, J. Colloid Interface Sci., 2008, 319, 199–205 CrossRef CAS PubMed.
- X. Tao, W. Ma, J. Li, Y. Huang, J. Zhao and J. C. Yu, Chem. Commun., 2003, 80–81 RSC.
- B. Basu, S. Satapathy and A. K. Bhatnagar, Catal. Rev.: Sci. Eng., 1993, 35, 571–609 CAS.
- R. J. Cremlyn, An Introduction to Organosulfur Chemistry, Wiley & Sons, New York, 1996 Search PubMed.
- H. Golchoubian and F. Hosseinpoor, Catal. Commun., 2007, 8, 697–700 CrossRef CAS PubMed.
- L. Menini, M. C. Pereira, A. C. Ferreira, J. D. Fabris and E. V. Gusevskaya, Appl. Catal., A, 2011, 392, 151–157 CrossRef CAS PubMed.
- S. Thurow, V. A. Pereira, D. M. Martinez, D. Alves, G. Perin, R. G. Jacob and E. J. Lenardao, Tetrahedron Lett., 2011, 52, 640–643 CrossRef CAS PubMed.
- T. V. Rao, K. N. Rao, S. L. Jain and B. Sain, Synth. Commun., 2002, 32, 1151–1157 CrossRef CAS PubMed.
- S. M. S. Chauhan, A. Kumar and K. A. Srinivas, Chem. Commun., 2003, 2348–2349 RSC.
- I. Chatti, A. Ghorbel, P. Grange and J. M. Colin, Catal. Today, 2002, 75, 113–117 CrossRef CAS.
- V. N. Nemykin, A. E. Polshyna, S. A. Borisenkova and V. V. Strelko, J. Mol. Catal. A: Chem., 2007, 264, 103–109 CrossRef CAS PubMed.
- H. Liu and E. Min, Green Chem., 2006, 8, 657–662 RSC.
- V. I. Iliev, A. I. Ileva and L. D. Dimitrov, Appl. Catal., A, 1995, 126, 3333–3340 CrossRef.
- T. Stuchinskaya, N. Kundo, L. Gogina, U. Schubert, A. Lorenz and V. Maizlish, J. Mol. Catal. A: Chem., 1999, 140, 235–240 CrossRef CAS.
- J. K. Joseph, S. L. Jain and B. Sain, Ind. Eng. Chem. Res., 2010, 49, 6674–6677 CrossRef CAS.
- J. Zhu, Y. Li, Y. Chen, J. Wang, B. Zhang, J. Zhang and W. J. Blau, Carbon, 2011, 49, 1900–1905 CrossRef CAS PubMed.
- M. Zhang, C. Chen, W. Ma and J. Zhao, Angew. Chem., 2008, 120, 9876–9879 CrossRef.
- B. Xiao, X. Wang, H. Huang, M. Zhu, P. Yang, Y. Wang and Y. Du, J. Phys. Chem. C, 2013, 117, 21303 CAS.
- S. Min and G. Lu, J. Phys. Chem. C, 2011, 115, 13938–13945 CAS.
- Z. Mou, Y. Dong, S. Li, Y. Du, X. Wang, P. Yang and S. Wang, Int. J. Hydrogen Energy, 2011, 36, 8885 CrossRef CAS PubMed.
- W. S. Hummers and R. E. Offeman, J. Am. Chem. Soc., 1958, 80, 1339 CrossRef CAS.
- J. Rouquerol, D. Avnir, C. W. Fairbridge, D. H. Everett, J. M. Haynes, N. Pernicone, J. D. F. Ramsay, K. S. W. Sing and K. K. Unger, IUPAC Recommendations, Pure Appl. Chem., 1994, 66, 1739 CrossRef CAS.
- S. Bose, T. Kuila, A. K. Mishra, N. H. Kim and J. H. Lee, J. Mater. Chem., 2012, 22, 9696–9703 RSC.
- Y. Zhang, X. Sun, L. Pan, H. Li, Z. Sun, C. Sun and B. K. Tay, J. Alloys Compd., 2009, 480, L17–L19 CrossRef CAS PubMed.
- T. Kuila, A. K. Mishra, P. Khanra, N. H. Kim and J. H. Lee, Nanoscale, 2013, 5, 52–71 RSC.
- N. Karousis, A. S. D. Sandanayaka, T. Hasobe, S. P. Economopoulos, E. Sarantopoulou and N. Tagmatarchis, J. Mater. Chem., 2011, 21, 109 RSC.
- X. Zhang, Y. Feng, S. Tang and W. Feng, Carbon, 2010, 48, 211–216 CrossRef CAS PubMed.
- L. Xiaolin, Z. Guangyu, B. Xuedong, S. Xiaoming, W. Xinran, W. Enge and D. Hongjie, Nat. Nanotechnol., 2008, 3, 538 CrossRef PubMed.
- T. Zhang, D. Zhang and M. A. Shen, J. Mater. Sci. Lett., 2009, 63, 2051–2054 CrossRef CAS PubMed.
- X. Zhang, Y. Feng, P. Lv, Y. Shen and W. Feng, Langmuir, 2010, 26, 18508–18511 CrossRef CAS PubMed.
- J. Zhu, Y. Li, Y. Chen, J. Wang, B. Zhang, J. Zhang and W. J. Blau, Carbon, 2011, 49, 1900–1905 CrossRef CAS PubMed.
- V. H. Pham, T. V. Cuong, S. H. Hur, E. W. Shin, J. S. Kim, J. S. Chung and E. J. Kim, Carbon, 2010, 48, 1945 CrossRef CAS PubMed.
- T. J. Wallace, A. Schriescheim, H. Hurwitz and M. B. Glaser, Ind. Eng. Chem. Prod. Res. Dev., 1964, 3, 237 CrossRef CAS.
- D. E. Jiang, G. Pan, B. Zhao, G. Ran, Y. Xie and E. Min, Appl. Catal., A, 2000, 201, 169–176 CrossRef CAS.
- M. Zhu, Z. Li, B. Xiao, Y. Lu, Y. Du, P. Yang and X. Wang, ACS Appl. Mater. Interfaces, 2013, 5, 1732–1740 CAS.
- G. Das, B. Sain, S. Kumar, M. O. Garg and G. Muralidhar, Catal. Today, 2009, 141, 152–156 CrossRef CAS PubMed.
- X. Sun, Z. Liu, K. Welsher, J. T. Robinson, A. Goodwin, S. Zaric and H. Dai, Nano Res., 2008, 1, 203–212 CrossRef CAS PubMed.
- Y. Yu, M. Zhou, W. Shen, H. Zhang, Q. Cao and H. Cui, Carbon, 2012, 50, 2539–2545 CrossRef CAS PubMed.
|
This journal is © The Royal Society of Chemistry 2014 |
Click here to see how this site uses Cookies. View our privacy policy here.