DOI:
10.1039/C4RA06892K
(Review Article)
RSC Adv., 2014,
4, 45504-45516
Two-photon polymerization of hydrogels – versatile solutions to fabricate well-defined 3D structures
Received
9th July 2014
, Accepted 2nd September 2014
First published on 2nd September 2014
Abstract
Hydrogels are cross-linked water-containing polymer networks that are formed by physical, ionic or covalent interactions. In recent years, they have attracted significant attention because of their unique physical properties, which make them promising materials for numerous applications in food and cosmetic processing, as well as in drug delivery and tissue engineering. Hydrogels are highly water-swellable materials, which can considerably increase in volume without losing cohesion, are biocompatible and possess excellent tissue-like physical properties, which can mimic in vivo conditions. When combined with highly precise manufacturing technologies, such as two-photon polymerization (2PP), well-defined three-dimensional structures can be obtained. These structures can become scaffolds for selective cell-entrapping, cell/drug delivery, sensing and prosthetic implants in regenerative medicine. 2PP has been distinguished from other rapid prototyping methods because it is a non-invasive and efficient approach for hydrogel cross-linking. This review discusses the 2PP-based fabrication of 3D hydrogel structures and their potential applications in biotechnology. A brief overview regarding the 2PP methodology and hydrogel properties relevant to biomedical applications is given together with a review of the most important recent achievements in the field.
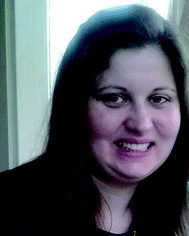 Adina I. Ciuciu | Adina I. Ciuciu completed her PhD in 2014 at the University of Bologna, with specialization in ‘Novel two photon absorbers: evaluation of photophysical properties in view of biomedical applications’. During her studies she was awarded a Marie Curie ITN fellowship (TOPBIO) and was enrolled as an early stage researcher at ISOF-CNR in Bologna. Her previous research focused on the functionalization of metallic surfaces of materials used for bioimplants. Currently, her research interests deal with photoinduced processes, two-photon absorption and biomaterials. |
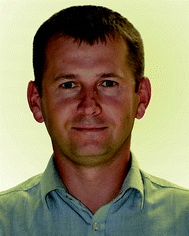 Piotr J. Cywiński | Piotr J. Cywinski is a senior researcher in the Nanopolyphotonics group at the Fraunhofer Institute for Applied Polymer Research. In 2003, he obtained his degree in Physics and in 2006 he obtained a PhD in Polymer Physics from the Lodz University of Technology. He moved to Germany in 2006 and was first a postdoctoral researcher at the Friedrich-Schiller University Jena and then at the University of Potsdam. Currently, he is pursuing research on linear and non-linear optical effects and their application in biomedicine. |
1. Introduction
1.1 Hydrogel fundamentals
Hydrogels are defined as water-swollen three-dimensional networks capable of imbibing water from an aqueous environment. In the entire volume, common hydrogels possess only 0.5–20 wt% of dry polymer mass.1 The remaining part is water and therefore hydrogels can provide a high resemblance to the extracellular matrix and good biocompatibility with living organisms. Moreover, the property of being soft while offering tissue-like elasticity minimizes irritation and damage to surrounding tissues. Nevertheless, the well-designed polymeric chemical structure and architecture is essential for the preparation of hydrogels. Depending on their origin, the polymeric compositions are classified into natural polymer hydrogels (e.g. collagen, dextran, fibrin, chitosan), synthetic polymer hydrogels (e.g. poly(ethylene glycol) derivatives) and their combination (e.g. collagen-acrylate, alginate-acrylate). While natural hydrogels can degrade easily on exposure to water-soluble enzymes, the degradation of synthetic hydrogels can occur via hydrolysis, solubilisation, mechanical erosion or can be adjusted by increasing the cross-linking densities.2 The properties of hydrogels are sought in a variety of applications, particularly in the biomedical field. The classification, preparation methods, main properties and applications of hydrogels are shown in Fig. 1. The potential of hydrogels to become useful biomaterials was first demonstrated in the 1960s when Wichterle and Lim cross-linked hydroxyethyl methacrylate (HEMA).3 Since then, numerous improvements in structure and properties of hydrogels4–6 have led to their use in cell encapsulation,7,8 wound healing9,10 and drug release.11–14 The extraordinary properties of hydrogels and associated significant experimental achievements have attracted the attention of scientific community to these materials. Hydrogel-related research resulted in more than 4000 publications in only the last four years. The previously published reviews emphasized the importance, applicability and progress of hydrogels in biology and medicine, predicting a bright future for them in science and technology.15–25
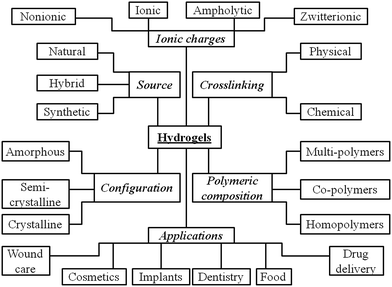 |
| Fig. 1 Simplified overview of hydrogel types, preparation methods, properties and applications. | |
1.2 Extracellular matrix
The extracellular matrix (ECM) is the space between cells and typically comprises fibrous proteins such as collagen, fibronectin, elastin and laminin, and proteoglycans (Fig. 2). Migratory cells innervate the ECM in response to receptor binding and the ECM retains biologically active molecules, such as growth factors, to regulate the cellular processes. A scheme presenting the ECM is provided in Fig. 2.26–28 When various mutations occur in ECM components, mild to severe diseases can affect the host organism. For example, tissue ageing, which is associated with unrepaired accumulation of naturally occurring DNA damage, is characterized by the decrease in cadherin, occludin or catenin and by the apparition of gaps between the cells.29,30 Under extreme conditions, the resultant failure of the wound healing process can promote chronic vascular remodelling and induce a tumor phenotype.31
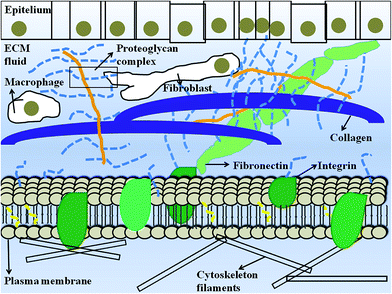 |
| Fig. 2 Schematic representation of ECM. Adapted with permission from Alberts et al.28 | |
Because of the important role that ECM plays, significant interest has emerged in developing new methods for constructing suitable materials that can mimic ECM. Novel materials should exhibit ECM properties, such as the sequestration, adhesion and proliferation of cells. Implicitly, the engineered structures require biocompatibility, mechanical strength and, when necessary, biodegradability. Hydrogels fulfill most of the aforementioned properties, and therefore, are excellent candidates for biomedical use, particularly for tissue engineering32–34 and drug release systems.35–38 Because soft tissue engineering targets volume restoration, limitations, such as cell survival, migration or exhaustion, and various mechanical challenges (e.g. scaffold collapse) must be considered. Hydrogels are considered to be ‘the first biomaterials developed for the human use’,39 and appear to address these issues in part.
In recent years, fabricating high resolution three-dimensional (3D) structures in hydrogels has been one of the major challenges. For this purpose, two-photon polymerization (2PP) has attracted considerable attention mainly due to the accuracy of fabricating structures with a spatial resolution down to 9 nm.40,41 The next section will describe this field in more details.
2. Two-photon polymerization (2PP)
2.1. Photopolymerization mechanism
Tissue or organ transplantation is one of the most challenging regenerative medicine processes faced by researchers. From a biological perspective, cell cultures seeded on fabricated structures can face difficulties in adhesion, proliferation or cell–cell interactions due to unnatural support. For these particular requirements, scaffold dimensions must be resolved at a sub-micrometer scale. An exciting route to fabricate well-defined low-dimensional 3D structures is offered by rapid prototyping techniques that provide precise control over the porosity and/or the mechanical properties of the substrate. Among these methods, only a few could be used with hydrogels because they require mild conditions.1,42,43 The most commonly used preparation methods are laser-based, nozzle-based and printer-based systems.42 Despite the fact that these techniques are suited for 3D microstructure fabrication, they are also affected by a series of limitations such as scaffold shrinkage, low resolution, and the lack of full control over the properties of the material and the medium.42 A more robust approach to prepare hydrogels is to cross-link them using light-induced polymerization. Photopolymerization is a versatile process, affording high-efficiency room-temperature material processing with well-defined spatial and temporal control. The first step in photopolymerization is initiation in which a light-sensitive compound (a photoinitiator) is required to produce active species (radicals or cations) upon irradiation with UV, vis or IR light. The photoinitiator can dissociate to form primary radicals or can react with a second species via hydrogen abstraction, forming secondary radicals. Regarding the mechanism involved in photolysis, photoinitiation includes radical polymerization (through photocleavage and hydrogen abstraction) and cationic polymerization.44–46 A comparison of the photopolymerization mechanisms is shown in Fig. 3.
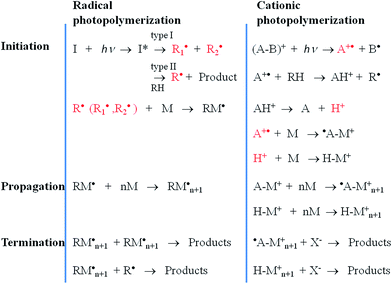 |
| Fig. 3 General mechanisms of radical and cationic photopolymerizations. | |
Photocleavage-based radical photopolymerization involves the formation of active species under light irradiation by the cleavage of chemical bonds (e.g. C–C, C
O, C–S, C–Cl). The photoinitiators used can be classified according to J.P. Fouassier47 as Norrish type I and II. Type I photoinitiators can be photo-fragmented into radicals upon absorption of light, whereas type II photoinitiators require the presence of a co-initiator such as an amine or an alcohol. In the case of Norrish type II systems, the radicals are generated at the co-initiator after transferring a hydrogen atom to the photoinitiator.
Radical photopolymerization by hydrogen abstraction involves aromatic ketones, which upon light exposure undergo hydrogen abstraction from a proton donor molecule to form a ketyl radical and a donor radical. Conventionally, the H-donor radical initiates the polymerization, while the ketyl radical undertakes radical coupling to the growing macromolecular chain.48–50
Cationic polymerization implies the use of a cationic initiator to transfer a charge to a monomer unit, which becomes reactive and interacts similarly with other units leading to polymer chain growth. The most commonly used cationic initiators are the Brønsted acids arenediazonium, diarylodonium and various sulfonium salts.51 However, in tissue engineering, cationic initiators are avoided because they generate protonic acids that affect cell cultures due to their strong acidic character.36
2.2. 2PP Working principle and instrumentation
Among all the methods involved in the fabrication of 3D scaffolds using the rapid prototyping technique, 2PP can be distinguished by its confined spatial resolution and mild processing conditions. It is a new method that has been broadly adopted for structuring medical devices at a small scale.52–54 2PP is a liquid-based rapid prototyping method that utilizes high power near-infrared (NIR) or infrared (IR) femtosecond lasers (e.g. a femtosecond pulsed titanium:sapphire (TiSa) laser). Fig. 4 illustrates the instrumental set-up typically used for 2PP. Unlike typical UV or vis-based photopolymerization where initiators produce radicals upon one photon absorption, initiators in 2PP generate radicals after absorbing two photons. If sufficient light power is provided at the focal point, free radical polymerization can be initiated. In this process, the probability of absorbing two photons (i.e. the two photon absorption (2PA) cross-section, σ2) by a specific molecule is quadratically dependent on the incident light intensity (eqn (1)),55,56 and therefore high intensity excitation lasers are required. The 2PA cross-sections are quantified in Göppert-Mayer units, 1 GM = 10−50 cm4 s per photon. The units were named after Maria Göppert-Mayer who predicted the two-photon absorption phenomenon in her doctoral dissertation. In the equation: |
 | (1) |
where
is the number of photons absorbed per unit time in the 2PA process, N represents the density of absorbing species, and F is the photon flux defined as follows:where I is the light intensity and hν is the photon energy.
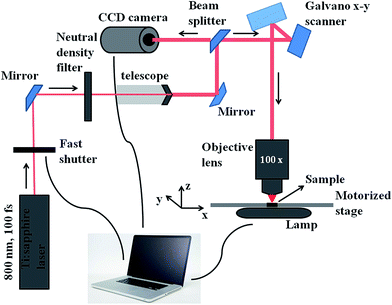 |
| Fig. 4 An instrumental setup typically used for 2PP processing. | |
In 2PP, excitation with a laser beam that is tightly focused in the focal volume allows the precise spatial confinement of matter. This accurate laser positioning permits the formation of well-defined three-dimensional microstructures. After the 2PP process, the non-polymerized material is washed away, leaving behind the polymerized structures. Importantly, because 2PP can be monitored using white light microscopy, any processing malfunction can be corrected on the fly. Three-dimensional characterization, such as geometry and surface roughness, of the fabricated microstructures is usually visualised by scanning electron microscopy (SEM).
2.3. Materials and applications
The main advantage of 2PP is in its distinctive spatial light confinement, leading to high 3D resolution that is unattainable for other rapid prototyping techniques. In general, the excellent reproducibility and quality of 3D scaffolds make 2PP a unique fabrication method. From a medical viewpoint, the permitted excitation made with a femtosecond laser is in the so-called ‘biological window’ (between 700 and 1050 nm), where tissues have a greater transparency.57,58 Despite the fact that 2PP was used practically to fabricate 3D structures around a living organism,59 the 2PP method would theoretically permit the 3D structuring of a material present inside biological tissues. In these conditions, the patient would benefit from the absence of scars as no surgical intervention occurred, and the in vivo fabricated structure would interact immediately with the surrounding environment, leading to fast recovery.
In the 2PP process, the photoinitiator and monomer types are both equally important. In principle, the photoinitiator molecule should possess a high initiation efficiency (i.e. high radical yield), good biocompatibility, acceptable hydrophilicity and low cytotoxicity. Even though a series of 2PA active chromophores were reported for bioimaging purposes, they were found to be unsuitable as photoinitiators.60,61 This was mainly due to the fact that fluorescence and radical generation are competitive processes of the excited state deactivation. The lack of suitable photoinitiators led to efforts in designing and synthesizing new compounds. From a medical perspective, in addition to the desired high yield of radical generation, these photoinitiators should not affect the biological environment. The ideal structures should be highly hydrophilic and non-cytotoxic, and these aspects were seriously considered while developing the 2PP photoinitiators.
A considerable expansion in the number of monomers used for the 2PP fabrication process occurred from the year 2000 onwards. For example, 2PP experiments were performed on acrylates, epoxy-based photoresists and inorganic materials, which have gained regular usage in multiphoton processes.62–65 Among them, the acrylates are preferred due to their high polymerization yield, good mechanical properties and resistance to elevated temperatures and harsh solvents. Later, it was noticed that the properties of the final polymer can be modified through actions on the monomer, and 2PP structuring was realized on inorganic–organic hybrid polymers such as ORMOCER.66,67 This material showed improved features because of universal monomers that possess both organic polymer properties (e.g. toughness) and glass-like properties (e.g. thermal stability and transparency).
In addition to biomedicine, the range of applications for the 3D structures fabricated by 2PP has been increasing progressively and the necessity for new materials whose properties could be tailored according to potential applications represent a demanding task (Fig. 5). Therefore, in addition to undoped microstructures characterized by limited applicability, novel doped resin formulations were reported. These formulations contained advanced materials (i.e. magnetic nanoparticles, conjugated polymers or bio-related materials) and were capable of conferring new functionalities. A few examples of doped resins together with their potential applications are shown in Fig. 5.68
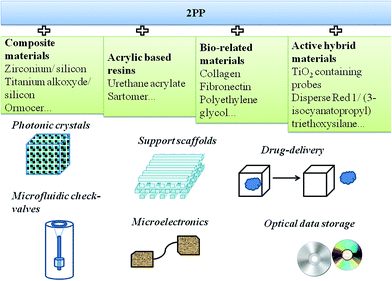 |
| Fig. 5 Materials and applications associated with 2PP process. | |
3. Two photon polymerization of hydrogels
3.1. Cross-linking of natural hydrogels
In their work on the cross-linking of natural hydrogels, Campagnola et al.69 used 2PP and naturally biocompatible macromolecules to create simple multidimensional structures from bovine serum albumin (BSA) or fibrinogen. The hydrogel cross-linking process involved transferring a hydrogen from the protein to the photoexcited photoinitiator Rose Bengal. The process likely occurs at the ketone of the Rose Bengal because the triplet state ketones abstract the protons and are reduced to alcohols, leading to the inability of the photoinitiator to cross-link. This effect showed a strong dependence on the Rose Bengal concentration and it was concluded that the photopolymerization mechanism does not regenerate the dye. In the same work, the group demonstrated the ability of the cross-linked BSA hydrogel to trap and subsequently release dextran, confirming the efficiency of the system as a drug delivery carrier. 2PP hydrogel formation was found to be particularly good for promoting cell adhesion because almost all the cell types require a 3D environment to mimic physiological tissue structures.70,71 Experiments executed to study the adhesion of human dermal fibroblasts and the enzymatic degradation of the cross-linked matrices confirmed the biocompatibility and biodegradability of these hydrogels.72,73 A few years later, the same group presented 2PP-induced collagen cross-linking using the same photoinitiator to form stable sub-micron structures in fast processing timescales.72,73 Because Rose Bengal displayed a low solubility at acidic pH, where collagens have the highest solubility, another photoinitiator that is soluble at acidic pH was considered. Consequently, they reported a newly synthesized benzophenone dimer that could act as a photoinitiator for both BSA and collagen cross-linking.73,74 However, cytotoxicity was not tested for the fabricated structures. In Campagnola's group, other proteins, such as fibrinogen, fibronectin and concanavalin A, were also processed using Rose Bengal as the photoinitiator. Notably, the hydrogels prepared based on the abovementioned proteins maintained their bioactivity after the 2PP process.75
In another example, Shear et al.76 used flavin adenine dinucleotide as photoinitiator to fabricate interactive BSA or avidin-based microstructures in the presence of developing neurons. They reported that fabricated protein microchambers are an appropriate environment for the capture and growth of motile cells, such as Escherichia coli, particularly due to the ability to exchange nutrients and waste through the structure walls via diffusion.77 The same E. coli was successfully trapped inside 3D protein structures (BSA, avidin, and poly(methyl methacrylate) (PMMA) microparticles). The structures were prepared with controlled densities, thickness gradients and swelling characteristics in response to pH variations.78 These studies provided significant input for the future design of smart protein-based materials.
3.2. Cross-linking of hybrid and artificial hydrogels
If the first decade of the last twenty years was dominated by the use of natural proteins to prepare hydrogels, modified and synthetic hydrogels became of interest in the second decade. The synthetic hydrogels provide improved properties (i.e. mechanical strength) in comparison to their natural counterparts, thereby reducing the difficulty in handling. Parallel to the need for novel hydrogel materials, another problem arose regarding the photoinitiator cytotoxicity. Ideally, both a hydrogel and photoinitiator should be fully biocompatible and noncytotoxic, which is critical when working with living cells. Thus, the groups approaching 2PP for hydrogel preparation were also working on the development of new photoinitiators with improved biocompatibility and higher 2PA cross-sections than commercial photoinitiators. The 2PP-based hydrogel fabrication in aqueous solution would allow working with cell cultures, whereas elevated 2PA cross-sections would allow lower laser powers and implicitly shorter irradiation times. A list of common photoinitiators, their 2PA cross-sections and the respective cross-linked hydrogels are presented in Table 1. The cell type tested on the 2PP fabricated structures and the intended applications are also listed.
Table 1 Photoinitiators with the corresponding cross-linked hydrogels, 2PA cross-sections (σ2), the cell type tested and potential applications
Photoinitiator |
σ2/GM |
Hydrogel type |
Cell type tested |
Application intended |
Ref. |
Name |
Structure |
WSPI:1,4-bis(4-(N,N-bis(6-(N,N,N-trimethylammonium)hexyl)amino)-styryl)-2,5-dimethoxybenzene tetraiodide; G2CK: sodium 2,2′-((((1E,1′E)-(5-methyl-2-oxocyclohexane-1,3-diylidene)bis(methanylylidene))bis(4,1-phenylene))bis(methylazanediyl))diacetate; P2CK: sodium 3,3′-((((1E,1′E)-(2-oxocyclopentane-1,3-diylidene)bis(methanylylidene))bis(4,1-phenylene))bis(methylazanediyl))dipropanoate. |
Rose Bengal |
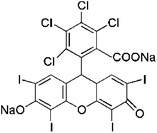 |
10 |
BSA, fibrinogen, collagen, fibronectin, concanavalin A |
Human dermal fibroblasts |
Drug delivery, scaffolds |
69, 72–74 |
Benzophenone dimer |
 |
— |
BSA, collagen |
— |
Drug delivery, scaffolds |
72 and 74 |
Flavin adenine dinucleotide |
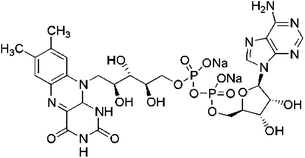 |
0.035 |
BSA, avidin, PMMA |
Cortical neurons, Escherichia coli |
Cell delivery |
76–78 |
Irgacure 369 |
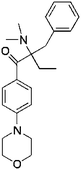 |
7 |
PEGDA |
Mouse fibroblasts |
Scaffolds |
79 |
Irgacure 651/AF240 |
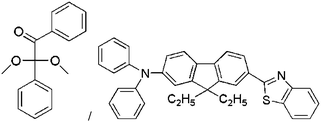 |
28 |
PEGDA/HEMA |
— |
Tissue engineering |
80 |
Irgacure 2959 |
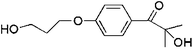 |
— |
GelMOD, HA |
Human adipose-derived stem cells, porcine mesenchymal stem cells, fibroblasts, osteoblasts |
Tissue engineering |
81–83 |
WSPIa |
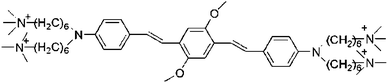 |
120 |
PEGDA, gelatin derivative |
C. elegans, osteosarcoma cells |
Tissue engineering |
84 and 85 |
G2CKa |
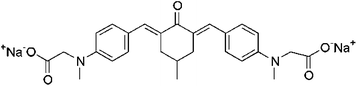 |
136 |
GelMOD |
Human osteosarcoma cells |
Stem cell research, drug delivery, tissue engineering |
85 and 86 |
P2CKa |
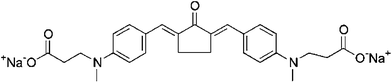 |
176 |
GelMOD |
Human osteosarcoma cells |
Stem cell research, drug delivery, tissue engineering |
85 and 86 |
West et al. took advantage of 2PP and its high resolution to cross-link poly(ethylene glycol) (PEG)-diacrylate (PEGDA) hydrogels.87 PEG derivatives were chosen because of their biocompatibility and resistance to protein adsorption and cell adhesion, while acrylate terminal groups were selected because of their enhanced radical generation efficiency in the presence of the selected photoinitiator.36,88–92 This study demonstrated that the 3D structures fabricated from PEGDA hydrogels are capable of guiding and simultaneously restricting fibroblast cell migration. Later, the same group successfully patterned the same hydrogel with collagenase-sensitive peptide chains, transforming a non-degradable and bioinert hydrogel into a degradable and cell-adhesive substrate.70
Anseth et al. prepared PEG-based hydrogels to encapsulate human mesenchymal stem cells.93,94 They demonstrated that stem cell morphology could be controlled at any time using UV, visible or two photon irradiation as well as through the degradation of the hydrogels.94 The same group proved the ability to control hydrogel physical and chemical properties by incorporating various functional groups, such as nitrobenzyl and peptide linkers, to control the degradation of the network.93
To shed more light on the modified PEG structures obtained under multiphoton irradiation, several research groups have recently presented results obtained from experiments executed in aqueous media in the presence of living cells/organisms. In a simple approach, low dimensional 3D structures were fabricated using commercially available photoinitiators such as organo-soluble Irgacure 369,79 Irgacure 651 and AF240 dissolved in water with a non-ionic surfactant (Pluronic F127).80 This demonstrated for the first time how a hydrophobic photoinitiator can be used directly for 2PP in aqueous solutions. Simple 2D structures from PEGDA and 2-hydroxiethyl methacrylate (HEMA) were fabricated in an aqueous medium. However, a large surfactant concentration was required, which increased the cytotoxicity of the structures when seeded with cells.
A limited selection of water soluble photoinitiators prompted researchers to pursue studies to design and synthesize novel classes of photoreactive dyes. In a different approach, Rose Bengal-triethanolamine was tested.64,95 However, this photoinitiator suffered from low 2PA cross-section therefore required high laser intensity and long exposure times. Several groups85,96–98 proved that an efficient way to produce water soluble photoinitiators is to create conjugated molecules. Quaternary ammonium cations or carboxylic sodium salts combined with an effective six-member ring in a series of benzilidene ketone-based water soluble photoinitiators increased the 2PA cross-sections up to 200 GM (see Table 1).
Ovsianikov et al. were also actively involved in the study on hydrogel cross-linking using modified naturally occurring macromolecules as monomers. They used modified gelatin-GelMOD and hyaluronic acid (HA) together with newly synthesized water-soluble photoinitiators. Irgacure 2959 was selected to form a support for a culture of human adipose-derived stem cells.82 This photoinitiator was chosen due to its reasonable hydrophilicity and reduced cytotoxicity. Not only did the final hydrogel structures preserve the enzymatic degradation of gelatin after the 2PP process, but cell addition and proliferation also occurred with satisfactory rates. In a further report, they used the same monomer and initiator to reproduce the CAD-software model with high precision (Fig. 6).83 The tests showed that the computer model was replicated precisely in both shape and porosity (with a pitch as small as 1.5 μm), opening a new door for geometrical tissue design and its formation in 3D. In the same study, the fabricated scaffolds provided good conditions for the attaching porcine mesenchymal stem cells and their subsequent proliferation. Upon applying an osteoinductive medium, the cells showed calcium deposition on the 3D scaffold. The abovementioned results show that hydrogel structures engineered accurately via CAD and 2PP can lead to novel perspectives for studying tissue formation in 3D.
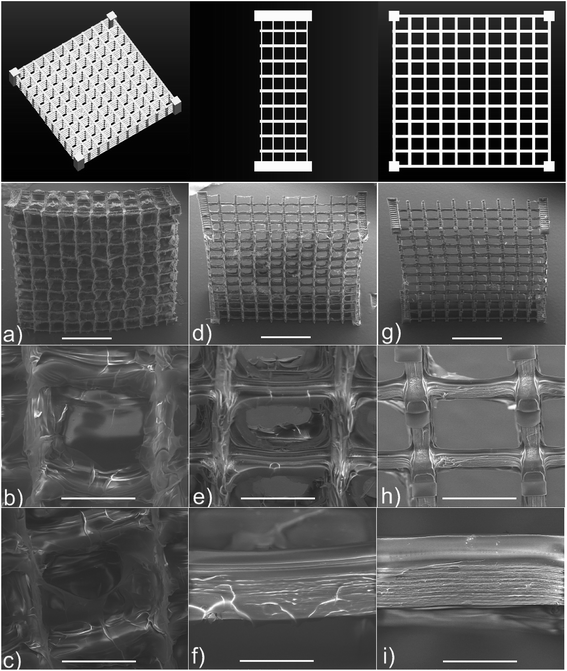 |
| Fig. 6 Snapshot of the CAD model and SEM images of gelatin methacrylamides scaffolds fabricated by 2PP: (a–c) untreated; (d–f) treated for 1 h and (g–i) treated for 2 h with 100 collagenase digestion unit per mL solution. Scale bar represents 1 mm for (a, d and g), 200 μm for (b, c, e and h) and 50 μm for (f and i). Reprinted (adapted) with permission from ref. 83. Copyright 2011 American Chemical Society. | |
The most recent achievements from the Liska and Stampfl research group are related to the microfabrication of hydrogels in the presence of living organisms.85 CAD scaffolds prepared using the PEGDA hydrogel in formulations with up to 80% water content were fabricated around living C. elegans. This nematode was a test organism chosen to demonstrate the biocompatibility of the scaffold. Because of the opacity of the tissues in the UV range, the in vivo use of the photoresponsive hydrogels is limited. This issue was overcome by the use of NIR lasers, as most of the living cells are transparent in this domain.99,100 Therefore, it was successfully shown that due to tissue transparency in the NIR region the organism did not suffer damage even under high power laser exposure.97 In a similar manner, GelMOD was processed in formulations with up to 80% water in the presence of living cells.86 In this case, the benzylidenecycloketones (G2CK and P2CK) were used as photoinitiators (Table 1) that allowed the fabrication of well-defined microstructures. Human osteosarcoma cells (MG63) incorporated in the cross-linked GelMOD hydrogel were affected during the 2PP fabrication process, whereas the ones found in the immediate proximity or within the 2PP structures survived the fabrication process. In this case, the cell damage occurred because reactive species were produced during 2PP. The authors also showed that the fabricated scaffolds were stable in the cell culture for at least three weeks, and in the third week the cells trapped in the structures were able to proliferate, occupying most of the available space.
The same group also adopted the newest strategy in the field of tissue engineering; namely, the assembly of 3D hydrogels via two-photon excited thiol–ene chemistry.84 They reported the synthesis of a vinyl ester derivative of gelatin (GH-VE) and its copolymerization with reduced BSA derivatives in the presence of a water-soluble photoinitiator, WSPI. Well-defined and stable microstructures were fabricated with high 2PP writing speed (ca. 50 mm s−1) at low laser power (as low as 20 mW). This writing speed was particularly important with respect to the previously reported 10 mm s−1 for PEGDA structures,101 and the results were not only due to the improved fabrication setup, but also due to the novel 2PP thiol–ene chemistry. The osteosarcoma cells seeded inside the GH-VE/BSA hydrogels with various macromer ratios showed a preference for the structure with higher gelatin (GH-VE) content. These results are particularly important in the view of information regarding cell–ECM interactions.
One of the latest works in the field has been published very recently by B. Chichkov et al.81 They functionalized a hyaluronic acid natural hydrogel with glycidyl methacrylate groups to increase its mechanical strength. Consequently, 3D microstructures with well-defined pore sizes were successfully fabricated by the 2PP technique by using an Irgacure 2959 photoinitiator. Their biocompatibility was tested with fibroblasts and osteoblasts. Slight cytotoxic effects were observed most likely due to the photoinitiator concentration. However, the study confirmed the potential use of these materials as biomatrices for guided bone formation.
Among the groups using the 2PP technique for cross-linking hydrogels, the M. Kellomäki group distinguished itself by a different approach in the fabrication method. They utilized a low-cost picosecond Nd:Yag laser for 2PP fabrication, instead of the expensive femtosecond Ti:Sapphire laser.102–105 Hybrid polymer-ceramic materials, such as Ormocomp105 or biocompatible proteins (e.g. avidin, BSA),104 were employed, and structures as small as 1 μm were obtained. A comparison of the results obtained using both lasers indicated comparable surface features and resolutions, despite the very low scanning speed of the Nd:Yag apparatus.104 In one of their recent publications, they used methacryloylated and acryloylated poly(α-amino acid)s to recreate the microarchitectures of the extracellular matrix.106 They compared these materials to commonly used poly(ethylene glycol) diacrylates and found that both methacryloylated and acryloylated poly(α-amino acid) hydrogels are suitable for 2PP with a wider processing window than PEG-based acrylates. Cell culture studies were also performed in this group. For example, human embrionic stem cell (hESC)-derived neuronal cells cultured on PEGDA or a poly(∑-caprolactone)-based oligomer (PCL-o) were able to attach on both material surfaces, but did not migrate.103 Only the cells placed inside the structures survived, whereas the ones found outside the scaffolds were dead. After a subsequent material functionalization, PEGDA and PCL-o could be used as guidance structures in neural cell culture applications. However, the most important achievement of this study is the potential use of a low-cost and affordable laser, such as Nd:Yag, for the fabrication of microstructures for cell-based applications.
4. Applications
The current literature gives an in-depth overview regarding possible cross-linked hydrogels and their applications in medicine, which span from tissue engineering to biosensors or drug delivery systems.81,97,107–109 Despite the fact that most of the methods used to date were different from 2PP, the applications perfectly match the objectives of hydrogels combined with the 2PP technique.
4.1. Tissue engineering
In tissue engineering, organ failure or damage is addressed by implanting another artificial comparable organ with a similar functionality. The solution for developing clinically relevant tissues in vitro is to restore the three-dimensional distribution of cells in the novel scaffold material while maintaining their viability and function. Regarding photopolymerizable hydrogels in tissue engineering, a review by Nguyen et al. covered their potential uses as: (a) barriers following tissue injury to ensure better healing, (b) localized drug delivery systems, (c) cell cages in order to ensure cell transplantation and (d) scaffold materials for regeneration of soft tissues.36 Several other comprehensive papers describe the properties and the applicability of hydrogels for tissue regeneration.42,110–112
For the 2PP technique, studies on the complex cellular migratory response are in progress and depend on different factors such as the concentration of biomolecules, laser scan speed and intensity, or other bioactive factors. Commonly, to allow the cytotoxicity and proliferation tests, the cells are seeded in the fabricated scaffold or incorporated in the monomer material (before laser fabrication). However, a few successful results are reported in the literature regarding 2PP fabrication of biomatrices for guided cell growth81,82,84,107 with promising perspectives.
4.2. Biosensors
In biosensors, hydrogels undergo swelling or shrinkage as function of external stimuli such as pH, temperature, solvent, electric and magnetic fields, light, etc. Such a response was predicted theoretically by Dusek and Patterson in 1968113 and proved experimentally ten years later.114,115 In the case of hydrogels, the swelling feature is particularly important for their mechanical properties and solute transport activity.116
Within this context, particular attention was given to the photoresponsive hydrogels, which under light irradiation can show changes in their physical and/or chemical properties such as elasticity, shape, viscosity or swelling. In such designs, rigorous requirements are necessary to choose the appropriate photoresponsive groups and scaffolds. Various methods were developed to introduce photo-responsiveness to hydrogels as described in a recent review by Tomatsu et al.99 They mentioned several possibilities such as (a) modification with photoresponsive groups, (b) modification with supramolecularly interacting groups responsive to photoirradiation, or (c) supramolecular hydrogel formation of photoresponsive low-molecular weight-gelators.
There are plenty reports in the literature describing the exploitation of hydrogels structures as biosensors,108 but to our knowledge only one paper reports a UV photoresponsive cantilever fabricated by 2PP.117 Despite the fact that the photo-induced command was slow and irreversible, the authors claimed to have achieved the 3D fabrication of novel components based on photoactive polymers, at the same time enlarging the horizon of 2PP applications. Another stimuli-responsive hydrogel was reported by Shear's group who fabricated a pH responsive two-photon fabricated PEGDA hydrogel.78
4.3. Drug delivery systems
Due to their biocompatibility and biodegradability, hydrogels are suitable materials for transporting cells and drugs. In general, they are not rejected by the human body and they can deliver and release material loaded beforehand when necessary. However, it is very often difficult to load the cells and drugs inside the hydrogels because of their poor mechanical strength. Several ways to handle this problem, such as cell immobilization or a pachyman-based hydrogel approach, are reviewed in a current paper by K. Deligkaris et al.108 Regarding multiphoton 2PP processing, Campagnola et al.69 reported the incorporation into and subsequent controlled release of fluorescently labelled polysaccharides from 3D BSA structures.
5. Conclusions, challenges and future perspectives
Well-defined 3D hydrogel structures with precise control over shape and pore dimensions were very difficult to achieve for many years. In the last decade, several research groups were engaged in multiphoton processing methods, as well as in the development of new photoinitiators with enhanced water solubility and high 2PA cross-sections. Various natural, hybrid and artificial hydrogels were cross-linked using 2PP, and well-defined and stable microstructures were obtained in most cases. The hydrogel biocompatibility was tested and, in general, satisfactory results were obtained regarding cytotoxicity, adhesion and migration of cells. When cell viability was affected, negative factors were identified (i.e. reactive species generated during the 2PP process). Currently, efforts are being made to eliminate these adversities. In 2PP, the exact reproducibility of the CAD drawings by the fabricated 3D structures is of particular importance in the evaluation for ceramics or hydrogels.54,82,118 The data obtained from computer tomography and the correlation to CAD software can lead to improvements in the precision of the 2PP fabrication process, and indirectly to high-quality structures. Overall, using 2PP as a processing technique and hydrogels as materials resulted in improved performance of scaffolds with potential application in the biomedical field.
Although the use of hydrogels in biomedical applications is preferred due to the abovementioned reasons, there are also some limitations to be considered and addressed. First, the design of a perfect photoinitiator remains a serious challenge. Then, poor mechanical strength leads to difficulties in hydrogel handling while loading cells or drugs. The full control over the hydrogel biodegradability also remains a problem to address. Another challenge is hydrogel sterilization before applying the fabricated 3D structures in vivo. The methods commonly used for sterilization, such as gamma irradiation or steam sterilization, significantly affect the hydrogel properties. As an alternative, a recently reported dense carbon dioxide-based technique can be applied for hydrogel sterilization.119 Nevertheless, other “hydrogel-friendly” sterilization procedures have to be developed.
There are clear indications that the abovementioned issues can be overcome and novel hydrogel-based 3D scaffolds can be created by 2PP and can be used for various exciting applications. Further hydrogel development with improved functionalities, such as transmission of specific spatially- and temporally-controlled signals, will have a significant impact on tissue engineering. Another promising field is the selective entrapment and release of a target object (e.g. cell or microorganism) combined with the simultaneous signalling of both events. In addition, good control over hydrogel structures and their ECM-like biodegradability represents a challenging task for synthetic chemists. At the same time, the use of 2PP to fabricate low dimensional 3D hydrogel structures has opened the door toward an accurate control over porosity as well as over the incorporation and distribution of various loadings within a hydrogel. Due to elevated operational costs, 2PP cannot generate many reproducible structures at the moment. However, a new setup allowing an enhanced writing speed while maintaining the process efficiency and high structural definition would open new frontiers for commercially available, cost-effective, low dimensional devices.
In summary, the cross-linking of hydrogels using the 2PP technique expands the area of novel tools for the biomedical field with major improvements ranging from the cellular to macro levels. Their use in regenerative processes could shortly become indispensable.
References
- R. Landers, U. Hubner, R. Schmelzeisen and R. Mulhaupt, Biomaterials, 2002, 23, 4437–4447 CrossRef CAS.
- D. Eglin, D. Mortisen and M. Alini, Soft Matter, 2009, 5, 938–947 RSC.
- O. Wichterle and D. Lim, Nature, 1960, 185, 117 CrossRef.
- H. Omidian, D. Mastropietro and U. Kandalam, J. Bioact. Compat. Polym., 2014, 29, 66–80 CrossRef PubMed.
- X. J. Liu, Y. Chen, Q. L. Huang, W. He, Q. L. Feng and B. Yu, Carbohydr. Polym., 2014, 110, 62–69 CrossRef CAS PubMed.
- Z. Hu and G. Chen, Adv. Mater., 2014, 26, 5950–5956 CrossRef CAS PubMed.
- X. Liu, Z. Lei, F. Y. Liu, D. J. Liu and Z. X. Wang, Biosens. Bioelectron., 2014, 58, 92–100 CrossRef CAS PubMed.
- K. Kato, K. Inukai, K. Fujikura and T. Kasuga, New J. Chem., 2014, 38, 3591–3599 RSC.
- Z. H. Zhou, J. H. Chen, C. Peng, T. L. Huang, H. Zhou, B. L. Ou, J. Chen, Q. Q. Liu, S. L. He, D. F. Cao, H. H. Huang and L. J. Xiang, J. Macromol. Sci., Part A: Pure Appl.Chem., 2014, 51, 318–325 CrossRef CAS.
- Z. J. Fan, B. Liu, J. Q. Wang, S. Y. Zhang, Q. Q. Lin, P. W. Gong, L. M. Ma and S. R. Yang, Adv. Funct. Mater., 2014, 24, 3933–3943 CrossRef CAS.
- T. Shirakura, T. J. Kelson, A. Ray, A. E. Malyarenko and R. Kopelman, ACS Macro Lett., 2014, 3, 602–606 CrossRef CAS.
- P. Paradiso, R. Galante, L. Santos, A. P. A. de Matos, R. Colaco, A. P. Serro and B. Saramago, J. Biomed. Mater. Res., Part B, 2014, 102, 1170–1180 CrossRef CAS PubMed.
- R. Oun, J. A. Plumb and N. J. Wheate, J. Inorg. Biochem., 2014, 134, 100–105 CrossRef CAS PubMed.
- T. S. Anirudhan and A. M. Mohan, RSC Adv., 2014, 4, 12109–12118 RSC.
- K. Deligkaris, T. S. Tadele, W. Olthuis and A. van den Berg, Sens. Actuators, B, 2010, 147, 765–774 CrossRef CAS PubMed.
- C. Goncalves, P. Pereira and M. Gama, Materials, 2010, 3, 1420–1460 CrossRef CAS PubMed.
- A. M. Kasko and D. Y. Wong, Future Med. Chem., 2010, 2, 1669–1680 CrossRef CAS PubMed.
- N. S. Satarkar, D. Biswal and J. Z. Hilt, Soft Matter, 2010, 6, 2364–2371 RSC.
- J. M. Zhu and R. E. Marchant, Expert Rev. Med. Devices, 2011, 8, 607–626 CrossRef CAS PubMed.
- T. K. Giri, D. Thakur, A. Alexander, K.J. Ajazuddin, H. Badwaik and D. K. Tripathi, Curr. Drug Delivery, 2012, 9, 539–555 CrossRef CAS.
- J. Kopecek and J. Y. Yang, Angew. Chem., Int. Ed. Engl., 2012, 51, 7396–7417 CrossRef CAS PubMed.
- T. T. Lau and D. A. Wang, Nanomedicine, 2013, 8, 655–668 CrossRef CAS PubMed.
- B. J. Tighe, Eye Contact Lens, 2013, 39, 4–12 Search PubMed.
- E. M. White, J. Yatvin, J. B. Grubbs, J. A. Bilbrey and J. Locklin, J. Polym. Sci., Part B: Polym. Phys., 2013, 51, 1084–1099 CrossRef CAS.
- J. Lam, N. F. Truong and T. Segura, Acta Biomater., 2014, 10, 1571–1580 CrossRef CAS PubMed.
- H. Jarvelainen, A. Sainio, M. Koulu, T. N. Wight and R. Penttinen, Pharmacol. Rev., 2009, 61, 198–223 CrossRef CAS PubMed.
- L. Schaefer and R. M. Schaefer, Cell Tissue Res., 2010, 339, 237–246 CrossRef CAS PubMed.
- B. Alberts, A. Johnson, J. Lewis, M. Raff, K. Roberts and P. Walter, Molecular Biology of the Cell, Garland Science, New York, 2002 Search PubMed.
- A. D. Akintola, Z. L. Crislip, J. M. Catania, G. Chen, W. E. Zimmer, R. C. Burghardt and A. R. Parrish, Am. J. Physiol. Renal Physiol., 2008, 294, F170–F176 CrossRef CAS PubMed.
- J. L. Bolognia, Am. J. Med., 1995, 98, 99S–103S CrossRef CAS.
- O. De Wever, P. Demetter, M. Mareel and M. Bracke, Int. J. Cancer, 2008, 123, 2229–2238 CrossRef CAS PubMed.
- A. Atala and R. Lanza, Methods of Tissue Engineering, Academia Press, USA, 2006 Search PubMed.
- J. L. Drury and D. J. Mooney, Biomaterials, 2003, 24, 4337–4351 CrossRef CAS.
- A. Khademhosseini and R. Langer, Biomaterials, 2007, 28, 5087–5092 CrossRef CAS PubMed.
- A. K. Bajpai, S. K. Shukla, S. Bhanu and S. Kankane, Prog. Polym. Sci., 2008, 33, 1088–1118 CrossRef CAS PubMed.
- K. T. Nguyen and J. L. West, Biomaterials, 2002, 23, 4307–4314 CrossRef CAS.
- J. K. Oh, R. Drumright, D. J. Siegwart and K. Matyjaszewski, Prog. Polym. Sci., 2008, 33, 448–477 CrossRef CAS PubMed.
- K. Vulic and M. S. Shoichet, J. Am. Chem. Soc., 2012, 134, 882–885 CrossRef CAS PubMed.
- J. Kopecek, Biomaterials, 2007, 28, 5185–5192 CrossRef CAS PubMed.
- M. Gu, Presented at CLEO/Europe-IQEC, 2013 Search PubMed.
- J. Torgersen, X.-H. Qin, Z. Li, A. Ovsianikov, R. Liska and J. Stampfl, Adv. Funct. Mater., 2013, 23, 4542–4554 CrossRef CAS.
- T. Billiet, M. Vandenhaute, J. Schelfhout, S. Van Vlierberghe and P. Dubruel, Biomaterials, 2012, 33, 6020–6041 CrossRef CAS PubMed.
- Y. Fuchs, O. Soppera and K. Haupt, Anal. Chim. Acta, 2012, 717, 7–20 CrossRef CAS PubMed.
- A. B. Scranton and C. N. Bowman, Photopolymerization Fundamentals and Applications, ACS Publishers, New Orleans, 1996 Search PubMed.
- C. Decker, J. Coat. Technol., 1987, 59, 97–106 CAS.
- J. Fouassier, Photoinitiation photopolymerization and photocuring, Hanser Publishers, New York, 1995 Search PubMed.
- J. P. Fouassier, Photoinitiation, Photopolymerization, and Photocuring: Fundamentals and Applications, Hanser, Munich, 1995 Search PubMed.
- K. Haraguchi and T. Takehisa, Adv. Mater., 2002, 14, 1120–1124 CrossRef CAS.
- I. L. Kim, R. L. Mauck and J. A. Burdick, Biomaterials, 2011, 32, 8771–8782 CrossRef CAS PubMed.
- J. J. Roberts and S. J. Bryant, Biomaterials, 2013, 34, 9969–9979 CrossRef CAS PubMed.
- J. V. Crivello, in Polymer Science: A Comprehensive Reference, ed. M. Moeller and K.
Matyjaszewski, Elsevier Science, 2012, vol. 4, pp. 919–955 Search PubMed.
- A. Doraiswamy, C. Jin, R. J. Narayan, P. Mageswaran, P. Mente, R. Modi, R. Auyeung, D. B. Chrisey, A. Ovsianikov and B. Chichkov, Acta Biomater., 2006, 2, 267–275 CrossRef CAS PubMed.
- S. D. Gittard, P. R. Miller, R. D. Boehm, A. Ovsianikov, B. N. Chichkov, J. Heiser, J. Gordon, N. A. Monteiro-Riviere and R. J. Narayan, Faraday Discuss., 2011, 149, 171–185 RSC.
- A. Ovsianikov, B. Chichkov, P. Mente, N. A. Monteiro-Riviere, A. Doraiswamy and R. J. Narayan, Int. J. Appl. Ceram. Technol., 2007, 4, 22–29 CrossRef CAS PubMed.
- M. Goppert-Mayer, Ann. Phys., 1931, 9, 273–295 CrossRef CAS.
- F. Terenziani, C. Katan, E. Badaeva, S. Tretiak and M. Blanchard-Desce, Adv. Mater., 2008, 20, 4641–4678 CrossRef CAS.
- R. R. Anderson and J. A. Parrish, J. Invest. Dermatol., 1981, 77, 13–19 CAS.
- C.-L. Tsai, J.-C. Chen and W.-J. Wang, J. Med. Biol. Eng., 2001, 21, 7–13 Search PubMed.
- J. Torgersen, A. Baudrimont, N. Pucher, N. Stadlmann, K. Cicha, C. Heller, R. Liska and B. Chichkov, In Vivo Writing using Two-Photon-Polymerization, 2010 Search PubMed.
- M. Taki, J. L. Wolford and T. V. O'Halloran, J. Am. Chem. Soc., 2004, 126, 712–713 CrossRef CAS PubMed.
- M. A. Albota, C. Xu and W. W. Webb, Appl. Opt., 1998, 37, 7352–7356 CrossRef CAS.
- T. Baldacchini, C. N. LaFratta, R. A. Farrer, M. C. Teich, B. E. A. Saleh, M. J. Naughton and J. T. Fourkas, J. Appl. Phys., 2004, 95, 6072–6076 CrossRef CAS PubMed.
- C.-H. Lee, T.-W. Chang, K.-L. Lee, J.-Y. Lin and J. Wang, Appl. Phys. A, 2004, 79, 2027–2031 CAS.
- M. Farsari, G. Filippidis, K. Sambani, T. S. Drakakis and C. Fotakis, J. Photochem. Photobiol., A, 2006, 181, 132–135 CrossRef CAS PubMed.
- A. Ovsianikov, S. Schlie, A. Ngezahayo, A. Haverich and B. N. Chichkov, J. Tissue Eng. Regener. Med., 2007, 1, 443–449 CrossRef CAS PubMed.
- J. Serbin, A. Egbert, A. Ostendorf, B. N. Chichkov, R. Houbertz, G. Domann, J. Schulz, C. Cronauer, L. Frοhlich and M. Popall, Opt. Lett., 2003, 28, 301–303 CrossRef CAS.
- R. Houbertz, S. Steenhusen, T. Stiche and G. Sext, Coherence and Ultrashort Pulse Laser Emission, InTech, 2010 Search PubMed.
- D. S. Correa, L. De Boni, A. J. G. Otuka, V. Tribuzi and C. R. Mendonça, Two-Photon Polymerization Fabrication of Doped Microstructures, InTechOpen, 2012 Search PubMed.
- J. D. Pitts, P. J. Campagnola, G. A. Epling and S. L. Goodman, Macromolecules, 2000, 33, 1514–1523 CrossRef CAS.
- S.-H. Lee, J. J. Moon and J. L. West, Biomaterials, 2008, 29, 2962–2968 CrossRef CAS PubMed.
- J. C. Hoffmann and J. L. West, Soft Matter, 2010, 6, 5056–5063 RSC.
- L. P. Cunningham, M. P. Veilleux and P. J. Campagnola, Opt. Express, 2006, 14, 8613–8621 CrossRef.
- J. D. Pitts, A. R. Howell, R. Taboada, I. Banerjee, J. Wang, S. L. Goodman and P. J. Campagnola, Photochem. Photobiol., 2002, 76, 135–144 CrossRef CAS.
- S. Basu, L. P. Cunningham, G. D. Pins, K. A. Bush, R. Taboada, A. R. Howell, J. Wang and P. J. Campagnola, Biomacromolecules, 2005, 6, 1465–1474 CrossRef CAS PubMed.
- S. Basu and P. J. Campagnola, J. Biomed. Mater. Res., Part A, 2004, 71A, 359–368 CrossRef CAS PubMed.
- B. Kaehr, R. Allen, D. J. Javier, J. Currie and J. B. Shear, Proc. Natl. Acad. Sci. U. S. A., 2004, 101, 16104–16108 CrossRef CAS PubMed.
- B. Kaehr and J. Shear, J. Am. Chem. Soc., 2007, 129, 1904–1905 CrossRef CAS PubMed.
- B. Kaehr and J. B. Shear, Proc. Natl. Acad. Sci. U. S. A., 2008, 105, 8850–8854 CrossRef CAS PubMed.
- A. Ovsianikov, M. Gruene, M. Pflaum, L. Koch, F. Maiorana, M. Wilhelmi, A. Haverich and B. Chichkov, Biofabrication, 2010, 2, 014104 CrossRef CAS PubMed.
- S. J. Jhaveri, J. D. McMullen, R. Sijbesma, L.-S. Tan, W. Zipfel and C. K. Ober, Chem. Mater., 2009, 21, 2003–2006 CrossRef CAS PubMed.
- O. Kufelt, A. El-Tamer, C. Sehring, S. Schlie-Wolter and B. N. Chichkov, Biomacromolecules, 2014, 15, 650–659 CrossRef CAS PubMed.
- A. Ovsianikov, A. Deiwick, S. Van Vlierberghe, M. Pflaum, M. Wilhelmi, P. Dubruel and B. Chichkov, Materials, 2011, 4, 288–299 CrossRef CAS PubMed.
- A. Ovsianikov, A. Deiwick, S. Van Vlierberghe, P. Dubruel, L. Moeller, G. Draeger and B. Chichkov, Biomacromolecules, 2011, 12, 851–858 CrossRef CAS PubMed.
- X.-H. Qin, J. Torgersen, R. Saf, S. Mühleder, N. Pucher, S. C. Ligon, W. Holnthoner, H. Redl, A. Ovsianikov, J. Stampfl and R. Liska, J. Polym. Sci., Part A: Polym. Chem., 2013, 51, 4799–4810 CrossRef CAS.
- Z. Li, J. Torgersen, A. Ajami, S. Muhleder, X. Qin, W. Husinsky, W. Holnthoner, A. Ovsianikov, J. Stampflbe and R. Liska, RSC Adv., 2013, 3, 15939–15946 RSC.
- A. Ovsianikov, S. Mühleder, J. Torgersen, Z. Li, X.-H. Qin, S. V. Vlierberghe, P. Dubruel, W. Holnthoner, H. Redl, R. Liska and J. Stampfl, Langmuir, 2014, 30, 3787–3794 CrossRef CAS PubMed.
- M. S. Hahn, J. S. Miller and J. L. West, Adv. Mater., 2006, 18, 2679–2684 CrossRef CAS.
- J. L. Hill-West, S. M. Chowdhury, R. C. Dunn and J. A. Hubbell, Fertil. Steril., 1994, 62, 630–634 CAS.
- J. L. Hill-West, S. M. Chowdhury, A. S. Sawhney, C. P. Pathak, R. C. Dunn and J. A. Hubbell, Obstet. Gynecol., 1994, 83, 59–64 CAS.
- J. L. West and J. A. Hubbell, Macromolecules, 1999, 32, 241–244 CrossRef CAS.
- B. K. Mann, A. S. Gobin, A. T. Tsai, R. H. Schmedlen and J. L. West, Biomaterials, 2001, 22, 3045–3051 CrossRef CAS.
- G. Dumanian, W. Dascombe, C. Hong, K. Labadie, K. Garrett, A. Sawhney, C. Pathak, J. Hubbell and P. Johnson, Plast. Reconstr. Surg., 1995, 95, 901–907 CAS.
- C. A. DeForest and K. S. Anseth, Nat. Chem., 2011, 3, 925–931 CrossRef CAS PubMed.
- A. M. Kloxin, A. M. Kasko, C. N. Salinas and K. S. Anseth, Science, 2009, 324, 59–63 CrossRef CAS PubMed.
- P. J. Campagnola, D. M. Delguidice, G. A. Epling, K. D. Hoffacker, A. R. Howell, J. D. Pitts and S. L. Goodman, Macromolecules, 2000, 33, 1511–1513 CrossRef CAS.
- X. Wan, Y. R. Zhao, J. Xue, F. Wu and X. Fang, J. Photochem. Photobiol., A, 2009, 202, 74–79 CrossRef CAS PubMed.
- J. Torgersen, A. Ovsianikov, V. Mironov, N. Pucher, X. Qin, Z. Li, K. Cicha, T. Machacek, R. Liska, V. Jantsch and J. Stampfl, J. Biomed. Opt., 2012, 17, 105008 CrossRef PubMed.
- Z. Li, N. Pucher, K. Cicha, J. Torgersen, S. C. Ligon, A. Ajami, W. Husinsky, A. Rosspeintner, E. Vauthey, S. Naumov, T. Scherzer, J. Stampfl and R. Liska, Macromolecules, 2013, 46, 352–361 CrossRef CAS.
- I. Tomatsu, K. Peng and A. Kros, Adv. Drug Delivery Rev., 2011, 63, 1257–1266 CrossRef CAS PubMed.
- P. Lenz, Photochem. Photobiol., 1995, 62, 333–338 CrossRef CAS PubMed.
- J. Torgersen, A. Ovsianikov, V. Mironov, N. Pucher, X. Qin, Z. Li, K. Cicha, T. Machacek, R. Liska, V. Jantsch and J. Stampfl, J. Biomed. Opt., 2012, 17 Search PubMed.
- A. M. Haaparanta, E. Jarvinen, I. F. Cengiz, V. Ella, H. T. Kokkonen, I. Kiviranta and M. Kellomaki, J. Mater. Sci.: Mater. Med., 2014, 25, 1129–1136 CrossRef CAS PubMed.
- J. E. Koskela, S. Turunen, L. Yla-Outinen, S. Narkilahti and M. Kellomaki, Polym. Adv. Technol., 2012, 23, 992–1001 CrossRef CAS.
- S. Turunen, et al., Biofabrication, 2011, 3, 045002 CrossRef CAS PubMed.
- E. Käpylä, et al., J. Micromech. Microeng., 2011, 21, 065033 CrossRef.
- E. Kapyla, T. Sedlacík, D. B. Aydogan, J. Viitanen, F. Rypacek and M. Kellomakia, Mater. Sci. Eng., C, 2014, 43, 280–289 CrossRef CAS PubMed.
- A. Ovsianikov, M. Malinauskas, S. Schlie, B. Chichkov, S. Gittard, R. Narayan, M. Loebler, K. Sternberg, K. P. Schmitz and A. Haverich, Acta Biomater., 2011, 7, 967–974 CrossRef CAS PubMed.
- K. Deligkaris, T. Shiferaw, W. Olthuis and A. van den Berg, Sens. Actuators, B, 2010, 147, 765–774 CrossRef CAS PubMed.
- M. T. Raimondi, S. M. Eaton, M. M. Nava, M. Laganà, G. Cerullo and R. Osellame, J. Appl. Biomater. Biomech., 2012, 10, 55–65 Search PubMed.
- B. Adnadjevic and J. Jovanovic, Int. J. Polym. Sci., 2011, 343062 Search PubMed.
- S. Khetan and J. A. Burdick, Soft Matter, 2011, 7, 830–838 RSC.
- E. C. Spivey, E. T. Ritschdorff, J. L. Connell, C. A. McLennon, C. E. Schmidt and J. B. Shear, Adv. Funct. Mater., 2013, 23, 333–339 CrossRef CAS.
- K. Dusek and D. Patterson, J. Polym. Sci., Part A-2, 1968, 6, 1209–1216 CrossRef CAS.
- T. Tanaka, Phys. Rev. Lett., 1978, 40, 820–823 CrossRef CAS.
- J. Hrouz, M. Ilavsky, K. Ulbrich and J. Kopecek, Eur. Polym. J., 1981, 17, 361–366 CrossRef CAS.
- J. L. Drury and D. J. Mooney, Biomaterials, 2003, 24, 4337–4351 CrossRef CAS.
- T. Watanabe, M. Akiyama, K. Totani, S. M. Kuebler, F. Stellacci, W. Wenseleers, K. Braun, S. R. Marder and J. W. Perry, Adv. Funct. Mater., 2002, 12, 611–614 CrossRef CAS.
- A. Ovsianikov, S. Schlie, A. Ngezahayo, A. Haverich and B. N. Chichkov, J. Tissue Eng. Regener. Med., 2007, 1, 443–449 CrossRef CAS PubMed.
- S. S. Karajanagi, R. Yoganathan, R. Mammucari, H. Park, J. Cox, S. M. Zeitels, R. Langer and N. R. Foster, Biotechnol. Bioeng., 2011, 108, 1716–1725 CrossRef CAS PubMed.
|
This journal is © The Royal Society of Chemistry 2014 |