DOI:
10.1039/C4RA06648K
(Paper)
RSC Adv., 2014,
4, 46208-46213
Facile synthesis of nickel hydroxide–graphene nanocomposites for insulin detection with enhanced electro-oxidation properties
Received
4th July 2014
, Accepted 29th August 2014
First published on 1st September 2014
Abstract
This study describes a facile and effective one-pot route to synthesize structurally uniform and electrochemically active nickel hydroxide–graphene nanocomposites (Ni(OH)2–GN) and investigates the electrocatalytic activity toward the oxidation of insulin. Graphene here was used to tether Ni2+ precursor onto surfaces and eventually grow Ni(OH)2 nanoparticles to form hybrid materials. The synthetic Ni(OH)2–GN nanocomposite has a uniform surface distribution, which was characterized with scanning electron microscopy (SEM). Moreover, the composition of synthetic Ni(OH)2–GN nanocomposite was characterized by X-ray photoelectron spectroscopy (XPS) and Fourier transform infrared spectroscopy (FT-IR spectra). The Ni(OH)2–GN were electrochemically treated in 0.1 M NaOH solution through cyclic voltammograms, and then gradually transited into nickel oxyhydroxide–graphene nanocomposites (NiOOH–GN), which demonstrated high catalytic activity and improved stability to insulin oxidation. The steady-state current response increases linearly with insulin concentration from 800 nM to 6400 nM with a fast response time of less than 2 s and a detection limit of 200 nM. The excellent performance of insulin sensor, including long term stability, can be ascribed to the synergistic effects of the large surface area (resulting in high loading ability), dispersing ability and conductivity of graphene and the large surface-to-volume ratio and electrocatalytic activity of Ni(OH)2 nanoparticles.
Introduction
Insulin is an important polypeptide hormone that is used to regulate blood glucose by signaling when high levels of blood glucose are present in the body.1–5 It is reported that the prevalence of diabetes for all age groups worldwide is estimated at 2.8% for 2000 and may be rising to 4.4% in 2030.6 In addition, insulin serves as a predictor for diabetes of insulinoma and trauma.7,8 As a result, the detection of insulin is of great importance for clinical diagnostics, medical and physiological studies on diseases. The methods for detection of insulin mainly include bioassays, immunoassays and chromatography.4 These current technologies for the detection of insulin are slow, somewhat complex, time-consuming and they require special instruments as well as derivatization materials.
Zhang et al. studied the insulin electro-oxidation at electrode and found that there was an implied electrode process with an H+/e− ratio equal to 1, which could be induced by the oxidation process that involves redox-active tyrosine residues in the insulin molecule.2 The oxidation of the amino acid tyrosine involves its hydroxyl group and results in a release of a proton, i.e., C–OH → C
O + H+ + e−. Direct detection of insulin by electrochemical means such as on glassy carbon surface offers an excellent opportunity for the development of fast, sensitive and inherently simple systems. This direct electrochemical oxidation approach, however, falls in its drawbacks such as slow oxidation kinetics and surface fouling, which are overcome by complex surface modification9–11 and/or the use of mediators. Recently, all sorts of materials, such as silica gel,9 cobalt oxide nanoparticles11 and carbon nanotube,2,10 were also used as an electrode modifier for the oxidation of insulin. Although the modified electrodes have been successfully employed for monitoring insulin, they usually present several disadvantages, such as reduced stability of mediators and electrocatalysts, and progressive fouling of electrode surface during insulin oxidation, or both, resulting in low sensitivity and poor reproducibility.12–14 As a result, electrochemical catalysts able to accelerate electron transfer have been urgently hunted and used for the electro-oxidation and determination of insulin.
Graphene is a novel carbon material composed of a single layer of carbon atoms tightly packed into a two-dimensional honeycomb lattice structure.15–17 Recently, graphene has drawn tremendous attention in many fields, such as nanomaterials, nanotechnology, and sensors,18–20 due to its ultra-high specific surface area, and it can act as an ideal base for nanocomposites. Moreover, high electron conductivity, fast heterogeneous electron-transfer rate allows graphene to act as a robust atomic-scale scaffold for nanoparticles and form hybrid materials with improved properties.21,22 To date, graphene has been developed as a burgeoning support to disperse and stabilize metal, metal oxide, and semiconductor nanomaterials such as Ag, Au, Pt, and Ni(OH)2.23,24
Inorganic nanomaterials, such as Cu, Fe, Ni, and their oxides and sulfides, have attracted considerable attention as sensors and biosensors due to their low cost, high specific surface area, good electrocatalytic activity, and the possibility of promoting electron transfer rates.25–27 Recently, some forms of Ni and Ni(OH)2 nanomaterials have been utilized in the fabrication of biosensors because they are inexpensive, nontoxic and due to their readily stored properties.28–30 Unfortunately, van der Waals force between nanoparticles causes strong aggregation, which prevents effective catalytic contribution of each nanocatalyst.31,32 In addition, due to the semiconducting nature of Ni(OH)2, Ni(OH)2 are not favorable for applications in electrode materials for electrocatalysis. Thus, it is highly desirable to construct well-dispersed nanocatalysts on electronically conductive support to reduce ohmic resistance and improve the electrochemical activity. Dai's group reported a two-step method to grow Ni(OH)2 nanocrystals on graphene by hydrolysis of Ni(CH3COO)2 at 80 °C in a mixed N,N-dimethylformamide–H2O solvent, followed by dispersing the products in pure H2O for hydrothermal treatment at 180 °C, during which the small Ni(OH)2 particles adhere on graphene.33 Duan's group demonstrated a one-step hydrothermal strategy to prepare 3D graphene–Ni(OH)2 composite hydrogels by heating a homogeneous aqueous mixture of graphene oxide, Ni(NO3)2, ammonia and hydrazine at 180 °C for 2 h.34 Li's group synthesized a reduced graphene oxide–Ni(OH)2 nanocomposite in which Ni2+ was first adsorbed onto monodispersed single layer graphene oxide (GO) sheets with PVP as the surfactant and then react with hydrazine and an ammonia solution at 95 °C. Then, Ni(OH)2 nanoplates were formed and assembled onto the reduced GO nanosheets that had been reduced by hydrazine from GO.35 However, these synthesis process involved in the mentioned three studies still need improvement considering more simple and involving less processes and reagents. Moreover, most graphene–Ni(OH)2 composites are used as supercapacitor electrode materials for energy storage, but the use of these composites as electrocatalysts for protein oxidation is rarely reported.
This study describes a facile one-pot method to synthesize Ni(OH)2–GN nanocomposite sensitive for insulin electro-oxidation. In the Ni(OH)2–GN composite material, nanoparticles of Ni(OH)2 are uniformly and directly grown on highly conductive graphene. The graphene sheets overlap with each other to afford a three-dimensional conductive network for fast electron transfer between the active materials and the insulin. The as-prepared Ni(OH)2–GN nanocomposites possess high dispersibility and conductivity, and exhibit excellent catalytic performance to construct an insulin sensor. This sensor showed high stability and fast amperometric response toward insulin detection. In contrast, pure graphene, pure Ni(OH)2 nanoaggregates and Ni(OH)2 nanoaggregates physically mixed with graphene sheets showed inferior performance to insulin electrochemical oxidation compared to Ni(OH)2 nanoparticles directly synthesized on highly conductive graphene sheets.
Experimental
Apparatus and electrodes
Electrochemical measurements were performed with a computer-controlled electrochemical analyser (CHI 600E, Chenhua, China) and a computer-controlled advanced electrochemical system (Princeton Applied Research (PARSTAT 2273)) in a two-compartment electrochemical cell with a bare or modified GCE (3 mm in diameter) working electrode, a platinum wire counter electrode, and an (Ag/AgCl)/V (KCl-saturated) reference electrode. All of the electrochemistry experiments were performed at room temperature.
Reagents
NiCl2·6H2O and porcine insulin (5733, >27 USP units per mg) were obtained from Aladdin. Graphene was obtained from Beijing Daoking Co. (Note: graphene used in this study is from chemical reduction of graphene oxide and may still be lightly oxidized.) All other chemicals (analytical grade) were purchased from Beijing Chemical Reagent Company (Beijing, China) and used without further purification. Ultra-pure water was obtained with a Milli-Q plus water purification system (Millipore Co. Ltd., USA, 18 MΩ cm).
Sample characterization
SEM (Scanning Electron Microscopy) images were obtained using a Hitachi S-2600N scanning electron microscope. FT-IR (Fourier Transform Infrared Spectroscopy) spectra were obtained using a Bruker Vector 22 FTIR spectrometer in the frequency range of 4000–500 cm−1. XPS (X-ray Photoelectron Spectroscopy) measurements were carried out on a VG Microtech ESCA 2000 using a monochromic Al X-ray source.
Synthesis of Ni(OH)2–GN
20 mg of graphene powders was dispersed in 10 mL deionized water by ultra-sonication for 1 h while 32.5 mg of NiCl2·6H2O was dissolved in 5 mL of deionized water. After mixing these two solutions with 30 min of vigorous agitation, 0.1 M NaOH solution was introduced dropwise into the mixture to adjust the pH to 11. Then, the solution was heated at 50 °C for 30 min under magnetic stirring, followed by refluxing for 8 h. At last, green precipitate was obtained and rinsed with ethanol and deionized water several times. The resulting nanocomposites were dried under vacuum at 60 °C for 24 h. For the synthesis process of pure Ni(OH)2, the conditions are identical, except for the absence of graphene in the system.
Preparation of the insulin sensor
In an electrochemical experiment, a GC (glassy carbon) electrode was polished with 1.0, 0.3 and 0.05 μm alumina slurry to a mirror-like surface, followed by rinsing thoroughly with deionized water. Then, 10 μL of the Ni(OH)2–GN suspension (5 mg μL−1 in DMF) was dropped onto the GCE surface, which was exposed to air to evaporate the solvent. Then, the electrode was rinsed thoroughly with deionized water to remove the physically adsorbed materials to form the Ni(OH)2–GN/GC electrode for insulin sensing.
Results and discussion
Morphology and composition of Ni(OH)2–GN
Fig. 1 shows the SEM images of the blank ITO substrate (A), graphene sheet (B), as-formed Ni(OH)2 (C) and Ni(OH)2–GN (D). The ITO substrate in Fig. 1A is smooth and apparently has no nano-structured material. When graphene film is coated on the surface of ITO substrate in Fig. 1B, some sheets structures are found as the texture of single-atom thick substrate, indicating the presence of graphene sheet. Fig. 1C presents the prepared Ni(OH)2 nanoparticles without the presence of graphene, apparently, Ni(OH)2 nanoparticles have a large size, an average diameter of 50 nm and are much more prone to aggregation compared with Fig. 1D. In Ni(OH)2–GN (Fig. 1D), single-crystalline nanoparticle of Ni(OH)2 with diameter of 5 nm are uniformly immobilized on highly conductive graphene, demonstrating nickel ion conversion to nickel hydroxide on the graphene surface. The graphene sheets overlap with each other to afford a three-dimensional conductive network for fast electron transfer between the active materials, Ni(OH)2, which may improve the electro-catalytic efficiency.
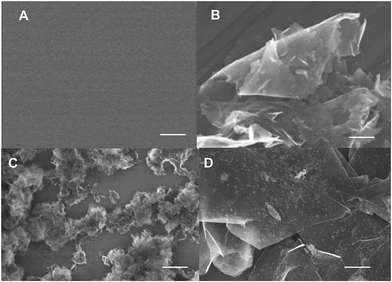 |
| Fig. 1 FE-SEM images of (A) ITO substrate, (B) graphene sheet, (C) as-formed Ni(OH)2 and (D) Ni(OH)2–GN. All scale bars are 400 nm. | |
In order to prove the presence of Ni(OH)2 in the graphene nanocomposites, the XPS spectra were investigated, which are shown in Fig. 2A. XPS was used to obtain semiquantitative data on the chemical compositions of obtained samples. Comparing the representative XPS survey spectra of Ni(OH)2–GN and GN, the existence of C and O is clearly observed for both samples while Ni peaks are only obviously appearing in Ni(OH)2–GN, confirming the successful incorporation of the Ni element into the nanocomposite in this study. Moreover, XPS spectra show that the peak characteristic of Ni 2p appears for Ni 2p3/2 at 856.75 eV, which is consistent with the presence of Ni(OH)2.36 Carbon content was calculated to be 91.4% and the oxygen content was calculated to be 7.8% in graphene–Ni(OH)2 nanocomposite, whereas carbon and oxygen contents in graphene were 91.7% and 8.3%, respectively. The decrease of relative content of carbon and oxygen after graphene is incorporated with Ni(OH)2 particles demonstrates that Ni element has been successfully grown on graphene surface. The IR spectrums obtained for Ni(OH)2–GN and graphene sample are shown in Fig. 2B. There are obvious peaks at 3643 cm−1, 3442 cm−1, 1631 cm−1, 1442 cm−1, 1117 cm−1, which are separately assigned to the stretching and bending vibrations of free O–H and certain O–H of H-bonded, C
C stretching carbonyl-conjugated, –CH2 and C–O stretching. The spectrum of the Ni(OH)2–GN sample obtained is similar to that graphene except for the new peak corresponding to O–H stretching observed at 3643 cm−1, which is associated with the OH vibrations, confirming the presence of Ni(OH)2 in the composite.37 This suggests that Ni(OH)2 nanoparticles are successfully immobilized onto the graphene sheets and without changing the chemistry environment of graphene.
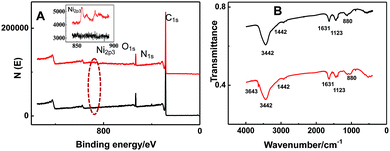 |
| Fig. 2 (A) Representative XPS survey spectra of graphene–Ni(OH)2 nanocomposite (red line) and graphene (black line), inset is the zoom of XPS scans over Ni2p3 peak in the dotted-line marked region. (B) FT-IR spectra of graphene–Ni(OH)2 (red line) and graphene sheet (black line). | |
Electrochemical characterization of the Ni(OH)2–GN/GC electrode
The electron transfer properties of the electrode after different surface modifications were characterized by electrochemical impedance spectroscopy (EIS) (Fig. 3). In general, the linear portion of the EIS represents the diffusion-limited process, and the semicircle portion corresponds to the electron transfer-limited process. The charge transfer resistance (Rct) at the electrode surface is equal to the semicircle diameter. A small well-defined semicircle with Rct of 268 Ω at higher frequencies was obtained for the bare GCE, which indicated a small interface electron transfer resistance. When Ni(OH)2–GN was immobilized on the GC electrode surface, the high frequency part in EIS was nearly a straight line, and the Rct value decreased to 108 Ω, which suggested that Ni(OH)2–GN can improve the conductivity and electron transfer process. These results also demonstrated that Ni(OH)2–GN was successfully immobilized on the GCE surface.
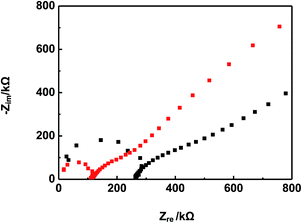 |
| Fig. 3 EIS obtained at the different electrodes: bare GC (black line) and Ni(OH)2–GN (red line). | |
Electrochemical treatment on Ni(OH)2–GN to form NiOOH–GN
The NiOOH–GN layer was generated using cyclic voltammetry on Ni(OH)2–GN modified electrode in the potential range from +0.25 to +0.6 V in pH 13 solution, as shown in Fig. 4. The oxidation and reduction process of Ni(OH)2 was studied in three different peaks in cyclic voltammetry. Basically, the electrocatalytic oxidation mechanism of the Ni(OH)2 nanocrystal modified electrode can be explained by the following equations.38
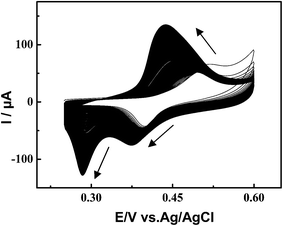 |
| Fig. 4 Cyclic voltammograms of graphene–NiOOH film growth on a carbon composite electrode in 0.1 M NaOH solution. The potential was continuously cycled at a scan rate of 100 mV s−1 between 0.25 V and 0.7 V vs. Ag/AgCl reference electrode. | |
Firstly, at potential of 0.42 V, Ni(OH)2–GN was oxidized at the electrode surface to NiOOH–GN. Then, NiOOH–GN was reduced back to Ni(OH)2–GN at 0.37 V, which would be electrochemically reoxidized in the anodic scan. With the increase in the cycle number, Ni(II) has been transferred to Ni(III), and Ni(III) is reverted to Ni(II) at a less positive potential. Moreover, the peak observed at 0.27 V during the cathodic process originates from the reduction of molecular oxygen, which was produced and adsorbed on the electrode surface during the anodic scan. As a characteristic of conductive film formation, the cathodic and anodic waves grew with the number of scans up to the 300th cycle, and then a current plateau and stable voltammetric response was obtained.
Electrocatalytic oxidation of insulin at the NiOOH–GN modified GCE
In order to investigate the electrocatalytic activity of the formed NiOOH–GN modified electrodes, cyclic voltammograms were obtained in the presence and absence of graphene and NiOOH–GN modified electrodes. Fig. 5A shows the recorded cyclic voltammograms of electrode modified with only graphene in the absence and presence of insulin in 0.1 M NaOH solution. There is no obvious anodic or reduction peak in the presence of 80 μM insulin in the potential range from 0.25 to 0.60 V. While in Fig. 5B, the recorded cyclic voltammograms of electrode modified with NiOOH–GN shows a dramatic enhancement of anodic peak current with the addition of 80 μM insulin, indicating a strong catalytic effect of NiOOH–GN to insulin oxidation. The enhancement peak current for insulin oxidation is achieved with the modified electrodes. The effect of adding insulin to the system was investigated in the range of 0–80 μM (Fig. 6). A linear dependence of the catalytic oxidation currents versus concentration of insulin can be fitted in the equation (Ip (μA) = 5.27 + 0.079Cinsulin (μM), R = 0.993). Ultra-high specific surface area, high electron conductivity and fast heterogeneous electron-transfer rate allow graphene to act as a robust atomic-scale scaffold for nanoparticles to form hybrid materials with improved properties. In addition, NiOOH nanoparticles with high catalytic activity to insulin oxidation make the NiOOH–GN composites exhibit superior catalytic activity to insulin oxidation.
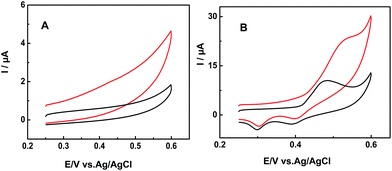 |
| Fig. 5 (A) Voltammetric response of graphene modified GCE placed in 0.1 M NaOH solution and 80 μM insulin 0.1 M NaOH solution, scan rate of 100 mV s−1. (B) Voltammetric response of graphene–NiOOH modified GCE placed in 0.1 M NaOH solution and 80 μM insulin 0.1 M NaOH solution, scan rate of 100 mV s−1. | |
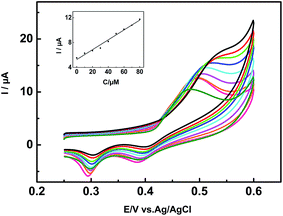 |
| Fig. 6 Voltammetric response of graphene–NiOOH modified GCE placed in 80 μM insulin 0.1 M NaOH solution at a scan rate of 50 mV s−1 to additions of insulin, oxidation currents from smaller to bigger 0, 10, 20, 30, 40, 50, 60, 70 and 80 μM. Inset: plot of insulin peak current versus insulin concentration. | |
Amperometric detection of insulin at NiOOH–GN modified GCE
Fig. 7 shows the amperometric response of NiOOH–GN on successive addition of insulin into 0.1 M NaOH solution (pH 13) at an applied potential of 0.51 V. When insulin was added to the stirring NaOH solution, Ni(OH)2–GN responded rapidly and achieved the maximum steady-state current in less 3 s. Catalytic currents showed linear response from 800 nM to 6400 nM (Ip (μA) = 0.08 + 0.05Cinsulin (μM), R = 0.98) with a detection limit of 200 nM. On the other hand, the NiOOH–GN nanocomposite results in higher stability for amperometric measurements of insulin. Fig. 7B displays the stability of the response to 7 μM insulin during an experiment. The response remains relatively stable throughout the entire 1 hour. Moreover, pure nickel hydroxide particles were synthesized using the same method as the Ni(OH)2–GN nanocomposite. When the synthetic nickel hydroxide particles were modified onto GC electrode, they were easier to drop off from electrode surface and dissolve in the alkaline solution owing to its high hydrophilicity. In the presence of graphene, the nickel hydroxide particles become smaller and uniform, tightly interact with graphene due to van der Waals force, and make the electrocatalytic surface more stable and efficient. In an attempt to consider the Ni(OH)2–GN/GC electrode for practical applications, we tested an artificial physiological matrix and spiked samples with different insulin concentrations, i.e. 1 μM, 5 μM, 20 μM on the Ni(OH)2–GN modified biosensor. The recovery and relative standard deviation values are acceptable with a value of 92.5–105.3%. As a result, the proposed sensor might be promising for the determination of insulin in clinical tests.
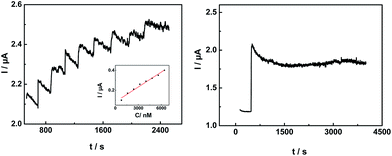 |
| Fig. 7 (A) Amperometric response of graphene–NiOOH/GCE on successive injection of insulin into 0.1 M NaOH solution. Applied potential: 0.51 V. Inset: plot of insulin peak current versus insulin concentration. (B) Stability of the amperometric response to 7 μM insulin during 4000 s. | |
Conclusions
In this work, we demonstrate a facile method for in situ growth of Ni(OH)2 nanoparticle on the surface of graphene sheets and a successful example of the detection of insulin based on as-prepared Ni(OH)2–GN modified GC electrode. The prepared electrochemical sensor exhibits much better catalytic performance compared with pure graphene, with a detection limit of 200 nM and a linear range between 0.8 μM and 6.4 μM. The proposed method was also successfully applied for the determination of insulin in human blood serum sample. High sensitivity, fast response and ease of preparation make this sensor promising for applications in the detection of some other proteins and amino acids.
Acknowledgements
This work was financially supported by the National Natural Science Foundation (21375088), the Scientific Research Project of Beijing Educational Committee (KM201410028006), the Scientific Research Base Development Program of the Beijing Municipal Commission of Education and the 2013 Program of Scientific Research Foundation for the Returned Overseas Chinese Scholars of Beijing Municipality.
References
- J. Y. Gerasimov, C. S. Schaefer, W. Yang, R. L. Grout and R. Lai, Biosens. Bioelectron., 2013, 42, 62–68 CrossRef CAS PubMed.
- M. Zhang, C. Mullens and W. Gorski, Anal. Chem., 2005, 77, 6396–6401 CrossRef CAS PubMed.
- Y. Wang and J. Li, Anal. Chim. Acta, 2009, 650, 49–53 CrossRef CAS PubMed.
- B. Rafiee and A. R. Fakhari, Biosens. Bioelectron., 2013, 46, 130–135 CrossRef CAS PubMed.
- B. B. Prasad, R. Madhuri, M. P. Tiwari and P. S. Sharma, Electrochim. Acta, 2010, 55, 9146–9156 CrossRef CAS PubMed.
- S. Wild, G. Roglic, A. Green, R. Sicree and H. King, Diabetes Care, 2004, 27, 1047–1053 CrossRef.
- A. Salimi, A. Noorbakhash, E. Sharifi and A. Semnani, Biosens. Bioelectron., 2008, 24, 792–798 CrossRef CAS PubMed.
- A. Salimi, L. Mohamadi, R. Hallaj and S. Soltanian, Electrochem. Commun., 2009, 11, 1116–1119 CrossRef CAS PubMed.
- M. Jaafariasl, E. Shams and M. K. Amini, Electrochim. Acta, 2011, 56, 4390–4395 CrossRef CAS PubMed.
- J. Wang, T. Tangkuaram, S. Loyprasert, T. Vazquez-Alvarez, W. Veerasai, P. Kanatharana and P. Thavarungkul, Anal. Chim. Acta, 2007, 581, 1–6 CrossRef CAS PubMed.
- A. Salimi and R. Hallaj, J. Solid State Electrochem., 2012, 16, 1239–1246 CrossRef CAS PubMed.
- L. Mercolini, A. Musenga, B. Saladini, F. Bigucci, B. Luppi, V. Zecchi and M. A. Raggi, J. Pharm. Biomed. Anal., 2008, 48, 1303–1309 CrossRef CAS PubMed.
- A. Arvinte, A. C. Westermann, A. M. Sesay and V. Virtanen, Sens. Actuators, B, 2010, 150, 756–763 CrossRef CAS PubMed.
- S. Regonda, R. Tian, J. Gao, S. Greene, J. Ding and W. Hu, Biosens. Bioelectron., 2013, 45, 245–251 CrossRef CAS PubMed.
- M. Quintana, E. Vazquez and M. Prato, Acc. Chem. Res., 2013, 46, 138–148 CrossRef CAS PubMed.
- F. Long, A. Zhu, H. Shi and H. Wang, Anal. Chem., 2014, 86, 2862–2866 CrossRef CAS PubMed.
- X. Wang, J. Wang, H. Cheng, P. Yu, J. Ye and L. Mao, Langmuir, 2011, 27, 11180–11186 CrossRef CAS PubMed.
- J. Zhu, M. Chen, Q. He, L. Shao, S. Wei and Z. Guo, RSC Adv., 2013, 3, 22790–22824 RSC.
- H. Zhou, X. Wang, P. Yu, X. Chen and L. Mao, Analyst, 2012, 137, 305–308 RSC.
- E. Asadian, S. Shahrokhian, A. I. zad and E. Jokar, Sens. Actuators, B, 2014, 196, 582–588 CrossRef CAS PubMed.
- C. Chung, Y.-K. Kim, D. Shin, S.-R. Ryoo, B. Hong and D.-H. Min, Acc. Chem. Res., 2013, 46, 2211–2224 CrossRef CAS PubMed.
- H. Wang, J. T. Robinson, G. Diankov and H. Dai, J. Am. Chem. Soc., 2010, 132, 3270–3271 CrossRef CAS PubMed.
- J. Bai and X. Jiang, Anal. Chem., 2013, 85, 8095–8101 CrossRef CAS PubMed.
- X. Zhu, Y. Zhong, H. Zhai, Z. Yan and D. Li, Electrochim. Acta, 2014, 132, 364–369 CrossRef CAS PubMed.
- X. Niu, Y. Li, J. Tang, Y. Hu, H. Zhao and M. Lan, Biosens. Bioelectron., 2014, 51, 22–28 CrossRef CAS PubMed.
- P. Si, Y. Huang, T. Wang and J. Ma, RSC Adv., 2013, 3, 3487–3502 RSC.
- W. Cheng, J. Sue, W. Chen, J. Chang and J. Zen, Anal. Chem., 2010, 82, 1157–1161 CrossRef CAS PubMed.
- Z. Fan, B. Liu, Z. Li, L. Ma, J. Wang and S. Yang, RSC Adv., 2014, 4, 23319–23326 RSC.
- Y. Miao, L. Ouyang, S. Zhou, L. Xu, Z. Yang, M. Xiao and R. Ouyang, Biosens. Bioelectron., 2014, 53, 428–439 CrossRef CAS PubMed.
- T. E. M. Nancy and V. A. Kumary, Electrochim. Acta, 2014, 133, 233–240 CrossRef CAS PubMed.
- P. R. Dalmasso, M. L. Pedano and G. A. Rivas, Sens. Actuators, B, 2012, 173, 732–736 CrossRef CAS PubMed.
- A. Ciszewski, K. Sron, L. Stepniak and G. Milczarek, Electrochim. Acta, 2014, 134, 355–362 CrossRef CAS PubMed.
- H. Wang, H. S. Casalongue, Y. Liang and H. Dai, J. Am. Chem. Soc., 2010, 132, 7472–7477 CrossRef CAS PubMed.
- Y. Xu, X. Huang, Z. Lin, X. Zhong, Y. Huang and X. Duan, Nano Res., 2013, 6, 65–76 CrossRef CAS.
- Y. Zhang, F. Xu, Y. Sun, Y. Shi, Z. Wen and Z. Li, J. Mater. Chem., 2011, 21, 16949–16954 RSC.
- B. V. Crist, Handbook of Monochromatic XPS Spectra-Vol. 1 – The Elements and Native Oxides, XPS International, Inc., 1999 Search PubMed.
- P. Jeevanandam, Y. Koltypin and A. Gedanken, Nano Lett., 2001, 1, 263–268 CrossRef CAS.
- Z. Chen, J. Nai, H. Ma and Z. Li, Electrochim. Acta, 2014, 116, 258–262 CrossRef CAS PubMed.
|
This journal is © The Royal Society of Chemistry 2014 |
Click here to see how this site uses Cookies. View our privacy policy here.