DOI:
10.1039/C4RA06636G
(Paper)
RSC Adv., 2014,
4, 39197-39203
Synthesis of tin superhydrophobic surfaces on zinc substrates
Received
4th July 2014
, Accepted 14th August 2014
First published on 14th August 2014
Abstract
A superhydrophobic surface with a water contact angle of 159 ± 2° and a sliding angle of 2 ± 2° on a zinc substrate is reported in this article. The process is composed of etching, replacement deposition and annealing treatment. The surface morphologies, chemical compositions and hydrophobicity of the as-prepared surfaces were investigated using a scanning electron microscope, powder X-ray diffraction analysis, X-ray photoelectron spectroscopy and contact angle measurements. The superhydrophobicity of the fabricated surface results from composite structures which consist of densely packed nanoscale particles composed of tin on microscale cavities. The optimal conditions and the formation mechanism of the superhydrophobic surfaces were also studied. Moreover, the potentiodynamic polarization shows that the as-prepared superhydrophobic surface has excellent corrosion resistance, indicating promising industrial applications.
1. Introduction
Superhydrophobic surfaces with a contact angle greater than 150° and a sliding angle less than 10° have attracted tremendous attention because of their great potential in industrial uses of self-cleaning,1,2 anti-bacterial,3,4 anti-icing,5 anti-corrosion,6,7 and oil–water separation.8 As is known, superhydrophobic surfaces have superhydrophobicity owing to their micro- and nano-structures and their unique chemical composition.9–12 According to the two aspects, researchers have successfully fabricated superhydrophobic surfaces by means of plasma etching,13–15 lithographic methods,16 chemical vapor deposition,17,18 electrochemical deposition,19–21 electrospinning,22,23 layer-by-layer processing scheme,24 laser pulses patterning,25–29 sol–gel process,30–34 etc. But most of these methods need strict conditions and organic substances to enhance superhydrophobicity. Therefore, researches tried to use simple methods without using organic substances modification to get superhydrophobic surfaces.
Chemical deposition through replacement reaction is a simple and basic method for the synthesis of nanostructured materials,35–38 especially in the synthesis of metal nanostructures owing to its technological applicability and easy control of morphology. What's more, fabricating superhydrophobic surfaces on metal substrates is of great importance on account of significant status of metal in the applications for the modern industry.39 Some researchers have prepared superhydrophobic surfaces on metal substrates via this electroless galvanic deposition as well as the modification of organic substances.40,41
Zinc (Zn) is one of the important metals in industry owing to its wide use in many applications such as a material to fabricate batteries, indicating it is essential to construct super-hydrophobic surfaces on zinc substrates. Qian et al.42 constructed superhydrophobic surfaces by simple chemical etching and Liu et al.43,44 also obtained superhydrophobic surfaces on zinc substrates by a simple immersion. However, organic substances were both used in the processes.
We are interested in fabricating superhydrophobic surfaces on metals via chemical deposition without organic substances modification. Here, we fabricated stable tin superhydrophobic surfaces via immersing zinc substrates into SnCl2 suspensions followed by anneal. Wettability, morphology, chemical compositions, corrosion resistance, influence factors and theoretical growth mechanism of the superhydrophobic surface were investigated.
2. Experimental
Preparation of superhydrophobic surfaces
All the chemicals were analytic grade reagents and purchased from Beijing Chemical Company, which need not further purification. Zinc substrates were obtained from Beijing Cuibolin Non-Ferrous Technology Developing Co. Ltd.
Zinc substrates (1.0 cm × 1.0 cm × 0.1 cm, Beijing, 99.999%) were ultrasonic cleaned in ethanol and deionized water respectively to remove the greasy dirt, then etched in 1 mol L−1 hydrochloric acid (HCl) solution for 5 min and rinsed with deionized water to remove the zinc oxide layer. The zinc substrates were horizontally immersed in 0.01 mol L−1 SnCl2 suspensions for 40 min at room temperature, then rinsed with deionized water. Dried in the air, the samples were put into Petri dish covered with filter paper, and annealed at 180 °C for 1 h in the oven.
Characterization
The static water contact angle (CA) and sliding angle (SA) were measured with approximately 8 μL water droplets using an optical contact angle meter (FAT200, Dataphysics Inc, USA) at ambient temperature. The average value was obtained after measuring more than five different positions of one sample. All values of each sample are in a range of ±2°, as is error bars in graph. Surface morphologies of samples were studied using a scanning electron microscope (SEM, S-4800, Hitachi, Japan). The chemical compositions of the samples were investigated with a X-ray diffractometer (XRD, D8 ADVANCE, Bruker, Gemany) using Cu Kα radiation (40 kV, 40 mA, and λ = 0.15418 nm) between 30° and 80° with X'Celerator's scanning rate of 4° min−1 and the X-ray photoelectron spectrometer (XPS, Model PHI 5300, Physical Electronics, USA) using 250 W Mg Kα (λ = 0.9891 nm) X-ray as the excitation source. Electrochemical corrosion test was carried out by an electrochemical workstation (CHI760E, Shanghai Huachen Instrument Co. Ltd., China) at room temperature. The potentiodynamic anodic polarization curves were recorded at a sweep rate of 5 mV s−1 from −1.40 V to −0.8 V.
3. Results and discussion
Via chemical deposition on zinc substrates in SnCl2 suspensions at room temperature and followed anneal, we obtained the superhydrophobic surfaces.
Wettability
As shown in Fig. 1a, the pure zinc substrate has a water CA 69°, which decreases to 28° after etching with HCl aqueous solution, as shown in Fig. 1b, and then increases to 65° after reacting with SnCl2 suspensions (Fig. 1c), indicating hydrophibility. However, after anneal treatment, the sample becomes superhydrophobic with a water CA of 159° as presented in Fig. 1d. From the result, a conclusion could be reached that etching, replacement deposition and anneal treatment are important in the fabrication of superhydrophobic surfaces.
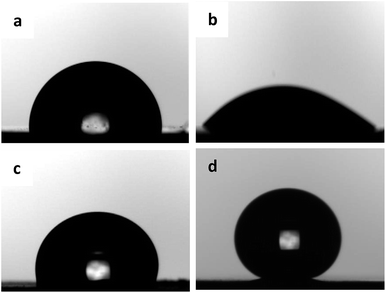 |
| Fig. 1 The shapes of 8.0 μL water droplet on the surface (a) untreated zinc, (b) etched in 1 mol L−1 HCl, (c) reacted in 0.01 mol L−1 SnCl2 suspensions for 40 min and (d) after annealed at 180 °C for 1 h. | |
An 8 μL droplet on the as-prepared superhydrophobic surface can readily move when tilted slightly (sliding angle 2 ± 2°), as shown in Fig. 2, which exhibits nonsticking behavior. While the other surfaces have high adhesion as the droplets never drop down even when the substrates turn upside down.
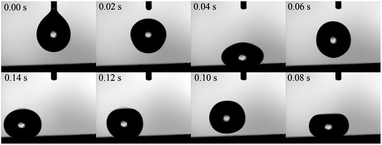 |
| Fig. 2 Successive snapshots of a 8.0 μL droplet on the superhydrophobic surface when tilted slightly (sliding angle 2 ± 2°). | |
The surface morphology
Surface morphologies of various samples were studied using a scanning electron microscope to better understand the wettability change.
Fig. 3a and b show zinc substrates before and after etching by HCl respectively. As can be seen in Fig. 3b, many holes similar to irregular hexagon-like honeycombs are obtained on the zinc substrate by etching in HCl. After reacting with SnCl2 suspensions, the surface morphology is composed of interesting composite structures which consist of densely packed nanoscale particles with an average diameter of 200–300 nm on the microscale cavities. Tiny particles with a higher density constructed the lower layer. The loose larger ones make up the upper layer, as shown in Fig. 3c and d. After anneal treatment, the shape of the hierarchical structures stays almost unchanged but the size of nanoscale particles increased with an average diameter of about 300–500 nm (Fig. 4e and f), That is, a novel hierarchical structure containing micro- and nanoscale structure is formed on the rough zinc substrate. Overall, this micro/nanoscale hierarchical structure would allow air to be trapped easily in porosity, and thus, water droplets can roll off from the surface with ease.
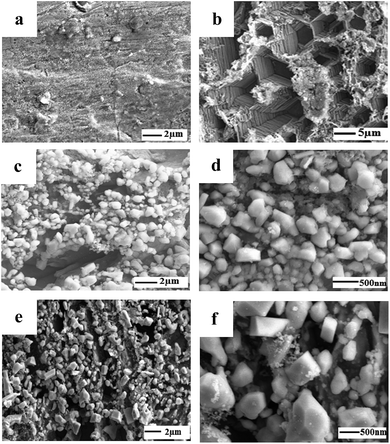 |
| Fig. 3 SEM images of samples: (a) untreated zinc, (b) zinc after etching by HCl aqueous solution, (c) reacted in the SnCl2 suspensions before anneal, (d) enlarged view of a microstructure in (c), (e) reacted in the SnCl2 suspensions after anneal, (f) enlarged view of a microstructure in (e). | |
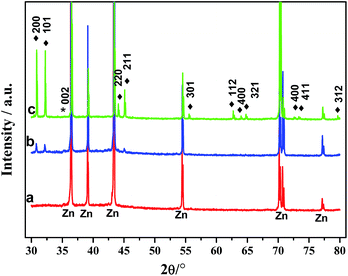 |
| Fig. 4 XRD patterns of the samples: (a) the untreated zinc, (b) treatment with SnCl2 suspensions, and (c) after anneal. | |
The surface chemical compositions
It is well known that the surface wettability is governed by its chemical compositions besides the surface morphology. Both the XRD and XPS measurements are used to investigate the chemical compositions on surfaces.
Fig. 4 shows XRD patterns of samples. Several strong peaks which are derived from the zinc substrate can be seen obviously in Fig. 4a. After reacting in SnCl2 suspensions for 40 min several peaks appear, as can be observed from the Fig. 4b. The peaks of crystalline Sn labeled with “♦” and crystalline ZnO labeled with “*” existed slightly on the surface. After anneal treatment, several strong diffraction peaks in oriented (200), (101), (220), (211) crystal face from the crystalline Sn were observed in Fig. 4c, whereas other Sn peaks are either relatively weak or not detected. The stronger peaks indicate the preferential growth of the tin nanostructures over a large substrate area during anneal process. All the peaks coincide with that of the standardized PDF card of crystal zinc (JCPDS Card no. 04-0831), ZnO (JCPDS Card no. 36-1451) and Sn (JCPDS card no. 04-0673).
To further confirm compositions on the Zn surface, Fig. 5 displays the XPS spectra of samples. The curves labeled with (A) and (B) respectively are the zinc substrates coated Sn before and after anneal treatment. Fig. 5a shows total XPS of samples, which reveals that Sn, Zn, Cl and O exist on the surfaces before and after anneal. Weak peaks at about 200.2 eV indicate the existence of chlorine element before and after anneal. Fig. 5b shows the XPS of tin element. From the deconvolution of the Sn 3d5/2 line of the spectrum before anneal, two peaks were obtained at 485.7 eV corresponding to zerovalent Sn and at 487.2 eV (Sn(OH)Cl) due to the hydrolysis of SnCl2.45 However, from the deconvolution of the Sn 3d5/2 line of the spectrum after anneal, three peaks were obtained at 485.6, 486.4 and 487.0 eV, corresponding to different types of species. The component at lower binding energy corresponds to zerovalent Sn. According to the literature, the line corresponding to Sn 3d5/2 for SnO has a binding energy at 486.5–486.8 eV, while the Sn 3d5/2 line for SnO2 has a binding energy at 486.5 eV,45 therefore, it is not possible to discriminate the oxidation state of Sn corresponding to the signal positioned at 486.4 eV. In consequence, the second peak will be assigned to Sn(II)/Sn(IV) oxides, while the one at 487.0 eV could be probably due to SnCl2 produced during anneal process.46 In Fig. 5c the peaks of curve (A) located at 1020.6 eV and 1044.1 eV are attributed to the Zn 2p3/2 and Zn 2p1/2, respectively. After anneal treatment, those of the curve (B) shift to higher binding energy at 1022.3 eV (Zn 2p3) and 1045.3 eV (Zn 2p1), respectively. The peak of O 1s shifts from 529.7 eV (curve A) to 531.6 eV (curve B) in Fig. 5d, combining the XPS of Zn, indicating that some ZnO have formed through anneal treatment.
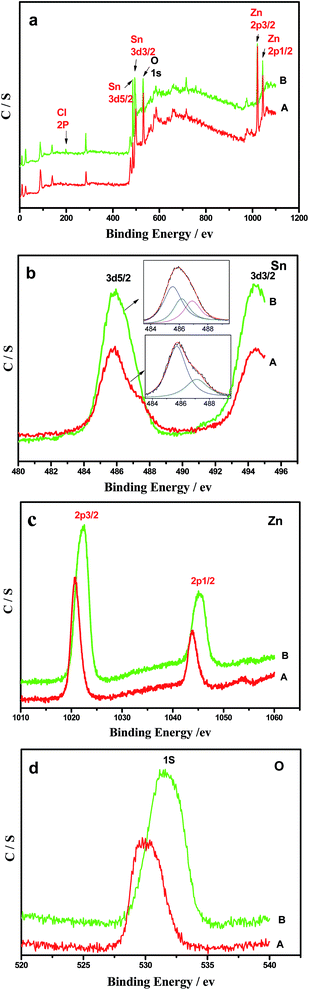 |
| Fig. 5 XPS spectra of the samples: (A) before anneal; (B) after anneal. | |
Stability and corrosion resistance
To test the environmental stability of the superhydrophobic surfaces, they were maintained at ambient temperature for six months and the static water CA of the samples didn't change a lot, indicating the novel composite structure is very stable in air.
Electrochemical corrosion test was carried out in a three electrode cell. A platinum plate and saturated calomel electrode (SCE) were used as the counter and reference electrode, respectively. The samples were used as a working electrode and measured in 3.0 wt% NaCl solution. Fig. 6 shows the potentiodynamic polarization curves of the bare Zn substrate and superhydrophobic surface. The corrosion potential (Ecorr), corrosion current density (icorr) and corrosion rate are given in Table 1 using the Tafel extrapolation from the potentiodynamic polarization curves.47 As expected, the superhydrophobic surface has much better corrosion resistance than the bare Zn substrate due to its lower corrosion current. The corrosion current density of the superhydrophobic surface is approximately 9.29% that of the bare Zn. The superhydrophobic surface exhibits very low corrosion rate, a surprising 13-fold decrease from the bare Zinc. We can conclude that the as-prepared superhydrophobic surface possesses a good anticorrosion behaviour for the bare zinc substrate, which removes a major barrier to its practical applications.
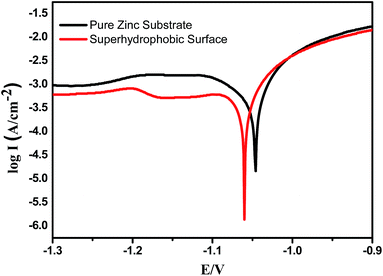 |
| Fig. 6 Potentiodynamic polarization curves measured in 3.0 wt% NaCl solution for the bare Zn substrate and superhydrophobic surface. | |
Table 1 Corrosion potential (Ecorr), corrosion current (icorr) and corrosion rate of the bare Zn and superhydrophobic surface
Sample |
Ecorr (V) |
Icorr (A cm−2) |
Corrosion rate (mm a−1) |
Bare Zn substrate |
−1.05 |
1.41 × 10−5 |
1.65 × 10−4 |
Superhydrophobic surface |
−1.06 |
1.31 × 10−6 |
1.28 × 10−5 |
Influence factors of wetting behaviours
Several influence factors should be considered to prepare the superhydrophobic surfaces, such as reaction time, concentration of SnCl2 suspensions, anneal time and anneal temperature. The effect of different reaction time on the wetting behaviour was shown in Fig. 7. It firstly increases from 69° to 149° with the increase of reaction time and reaches the maximum value of 159° at 40 min, and finally decreases to 147°. The result indicates the reaction time has a great effect on wettability of the samples.
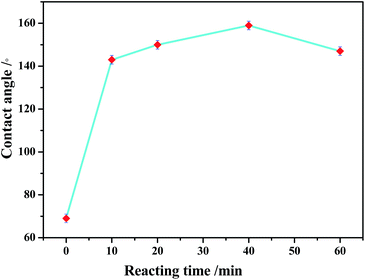 |
| Fig. 7 Water CAs of the as-prepared surfaces at different reaction time while immersed in 0.01 mol L−1 SnCl2 suspensions, followed by anneal at 180 °C for 1 h. | |
Fig. 8 shows SEM images of surfaces prepared at different reaction time, while other conditions kept constant. We can find that the density and sizes of particles increase evidently as the reaction time are varied. When reaction time is 10 min, densely packed particles diameter of about 50–100 nm grow on the edge of the hexagonal cavities, although the microscale cavities have no consistency about the shapes or sizes (Fig. 8a). When reaction time was 20 min, more particles about 100–200 nm formed on a large area of the zinc substrate which stuff part of the hexagonal cavities (Fig. 8b). Compared with Fig. 8b and c, the shape of composite structures stays almost unchanged. However, more particles with larger size are aggregated, exhibiting higher surface roughness when the reaction time reaches to 40 min, as observed in Fig. 8c. When the reaction time increased to 60 min, densely packed particles on zinc substrates have an average diameter approximately 1 μm, no significant nanoparticles could be observed on the surface. The surface approximately does not have uniform porous structures and interspaces. Thus, the air trapping within such rough surface would be less, and the roughness of the zinc substrate has changed clearly (Fig. 8d).
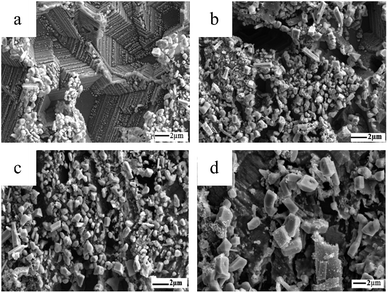 |
| Fig. 8 SEM images of the prepared samples obtained at different reaction time: (a) 10 min, (b) 20 min, (c) 40 min, and (d) 60 min while immersed in 0.01 mol L−1 SnCl2 suspensions, then annealed at 180 °C for 1 h. | |
Superhydrophobicity of the samples also depend on the concentration of SnCl2 suspensions, anneal time and anneal temperature in addition to the effect of reaction time. Fig. 9a shows the water CAs of the samples with different concentrations of SnCl2 suspensions. When the concentration increased from 0 mol L−1 to 0.025 mol L−1, the average water CA increases from 69° to 159°, and then decreases to 129°. The optimal concentration is 0.01 mol L−1. Fig. 9b shows water CAs varied under different anneal temperature and zinc substrates annealed at 180 °C is most superhydrophobic. Fig. 9c shows water CAs changes with different anneal time. When annealed from 0 h to 2.5 h, the average water CA increases from 65° to 159°, and then decreases to 129°. The optimal anneal time is 1 h.
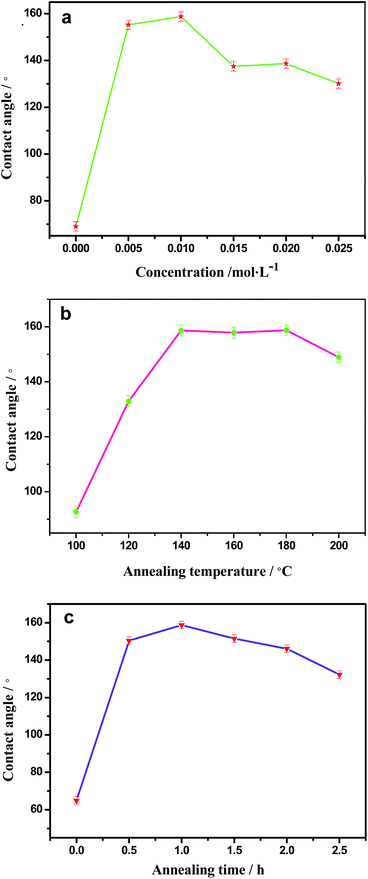 |
| Fig. 9 Water CAs of the as-prepared surfaces: (a) with different SnCl2 concentration while immersed for 40 min, followed by anneal at 180 °C for 1 h, (b) different anneal temperature while immersed in 0.01 mol L−1 SnCl2 suspensions for 40 min, followed by anneal for 1 h, (c) different anneal time while immersed in 0.01 mol L−1 SnCl2 suspensions for 40 min, followed by anneal at 180 °C. | |
The results above indicate that the optimal superhydrophobic surface with a water CA as high as of 159° and a SA as low as about 2° will be obtained by immersing the zinc substrate in 0.01 mol L−1 SnCl2 suspensions for 40 min and annealing at 180 °C for 1 h.
Theoretical growth mechanism for superhydrophobic surface
The formation mechanism of this superhydrophobic surface can be explained by the following discussions: first, by etching in HCl solution many holes similar to hexagon-like cavities are obtained on the zinc substrate, which was reported in a previous literature.48 Secondly, Sn2+ in the suspensions are deoxidize to Sn atom by Zn atom on the zinc substrate. It is well known that there is some Sn(OH)Cl precipitation in SnCl2 suspensions due to the hydrolysis of SnCl2 and some Sn(OH)Cl may adhere to the zinc substrate. There is an equilibrium precipitation and dissolution of Sn(OH)Cl in water and the replacement reaction can enhance the dissolution of Sn(OH)Cl. The chemical displacement continuously occurs on the zinc substrate. With the increase of reaction time and concentration of SnCl2, composite structures of densely packed nanoscale particles of tin are gradually formed on microscale cavities on the surface. The size of Sn particles is larger with longer growth time and higher concentration of SnCl2. However, it is inevitable that particles break away from the surface easily when the reaction time is long enough or the concentration of SnCl2 is very high, resulting in a roughness change, as shown in SEM images of Fig. 8d. Thirdly, when annealed, the tin particles grow larger and have preferential growth in certain orientations,49,50 leading a decrease in surface energy.51 Meanwhile, Sn(OH)Cl is decomposed to SnCl2, SnO and gaseous water during the anneal process and SnO2 also can be achieved, as shown in XPS images of Fig. 5b. However, peaks of Sn(OH)Cl, SnCl2, SnO and SnO2 are not very strong in the XRD spectra, maybe they exist in the amorphous state. The anneal treatment, as an industrial conventional method, changes the compositions of metal surfaces. The main reactions in the system are shown in the following equations: (Scheme 1).
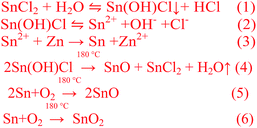 |
| Scheme 1 The main reactions in the formation of superhydrophobic surface. | |
Until now, two models are commonly employed to account for the wetting behaviour: the Wenzel and Cassie models.52,53 According to the Wenzel model, the liquids completely fill the valleys of this surface where they come into contact. In the Cassie model, liquids are assumed to only contact the solid through the top of the asperities and air pockets are assumed to be trapped underneath the liquid, which allows the liquid to roll off easily if tilted slightly. The as-prepared superhydrophobic surface with high water contact angle of 159° and a low SA of 2° can be explained in terms of the Cassie–Baxter equation between the water CA on a smooth surface (θ) and a rough surface (θ*).
In this equation, f1 is the fraction of the solid surface in contact with water and f2 is the ratio of air in contact with water respectively, and f1 + f2 = 1. For superhydrophobic samples with θ* = 159° and θ = 69°, the resulting values of f1 and f2 are about 0.0490 and 0.9510 respectively, indicating the air occupies the large fraction of the superhydrophobic surface, so that the water drop can slide quickly when the surface slightly tilted.
4. Conclusions
In this work, a superhydrophobic surface with a contact angle around 159° and a sliding angle around 2° is successfully constructed on the zinc substrate by a facile method. The superhydrophobicity of the constructed surface derives from its rough surface with a composite structures composed of densely packed nanoscale particles composed of tin on the microscale cavities. The as-prepared superhydrophobic surface has excellent corrosion resistance as well as stability. The fabrication process composed of etching, replacement deposition and anneal treatment offers an effective strategy and promising industrial applications for fabricating superhydrophobic surfaces on various metallic materials.
Acknowledgements
We gratefully thank the National Natural Science Foundation of China (no. 21271027) for this work.
Notes and references
- X. Li, D. Reinhoudt and M. Crego-Calama, Chem. Soc. Rev., 2007, 36, 1350 RSC.
- Y.-L. Zhang, H. Xia, E. Kim and H.-B. Sun, Soft Matter, 2012, 8, 11217 RSC.
- L. Shen, B. Wang, J. Wang, J. Fu, C. Picart and J. Ji, ACS Appl. Mater. Interfaces, 2012, 4, 4476 CAS.
- X. Zhang, L. Wang and E. Levänen, RSC Adv., 2013, 3, 12003 RSC.
- A. Otten and S. Herminghaus, Langmuir, 2004, 20, 2405 CrossRef CAS.
- P. M. Barkhudarov, P. B. Shah, E. B. Watkins, D. A. Doshi, C. J. Brinker and J. Majewski, Corros. Sci., 2008, 50, 897 CrossRef CAS PubMed.
- F. Su and K. Yao, ACS Appl. Mater. Interfaces, 2014, 6, 8762 CAS.
- J. Li, L. Shi, Y. Chen, Y. Zhang, Z. Guo, B. Su and W. Liu, J. Mater. Chem., 2012, 22, 9774 RSC.
- T. Kako, A. Nakajima, H. Irie, Z. Kato, K. Uematsu, T. Watanabe and K. Hashimoto, J. Mater. Sci., 2004, 39, 547 CrossRef CAS.
- D. Quéré, Rep. Prog. Phys., 2005, 68, 2495 CrossRef.
- M. Zielecka and E. Bujnowska, Prog. Org. Coat., 2006, 55, 160 CrossRef CAS PubMed.
- H. J. Ensikat, P. Ditsche-Kuru, C. Neinhuis and W. Barthlott, Beilstein J. Nanotechnol., 2011, 2, 152 CrossRef CAS PubMed.
- M. Lejeune, L. M. Lacroix, F. Brétagnol, A. Valsesia, P. Colpo and F. Rossi, Langmuir, 2006, 22, 3057 CrossRef CAS PubMed.
- R. Jafari, S. Asadollahi and M. Farzaneh, Plasma Chem. Plasma Process., 2013, 33, 177 CrossRef CAS.
- N. M. Oliveira, R. L. Reis and J. F. Mano, ACS Appl. Mater. Interfaces, 2013, 5, 4202 CAS.
- S. Tian, X. Xia, W. Sun, W. Li, J. Li and C. Gu, Nanotechnology, 2011, 22, 395301 CrossRef PubMed.
- K. K. S. Lau, J. Bico, K. B. K. Teo, M. Chhowalla, G. A. J. Amaratunga, W. I. Milne, G. H. McKinley and K. K. Gleason, Nano Lett., 2003, 3, 1701 CrossRef CAS.
- L. Huang, S. P. Lau, H. Y. Yang, E. S. P. Leong, S. F. Yu and S. Prawer, J. Phys. Chem. B, 2005, 109, 7746 CrossRef CAS PubMed.
- Z. Chen, L. Hao, M. Duan and C. Chen, Appl. Phys. A: Mater. Sci. Process., 2013, 111, 581 CrossRef CAS.
- M. Wolfs, T. Darmanin and F. Guittard, Macromolecules, 2011, 44, 9286 CrossRef CAS.
- M. Nicolas, F. Guittard and S. Géribaldi, Langmuir, 2006, 22, 3081 CrossRef CAS PubMed.
- D. Han and A. J. Steckl, Langmuir, 2009, 16, 9454 CrossRef PubMed.
- A. Singh, L. Steely and H. R. Allcock, Langmuir, 2005, 21, 11604 CrossRef CAS PubMed.
- J. Bravo, L. Zhai, Z. Wu, R. E. Cohen and M. F. Rubner, Langmuir, 2007, 23, 7293 CrossRef CAS PubMed.
- J. Yong, F. Chen, Q. Yang, D. Zhang, G. Du, J. Si, F. Yun and X. Hou, J. Phys. Chem. C, 2013, 117, 24907 CAS.
- X. Gao, X. Yao and L. Jiang, Langmuir, 2007, 23, 4886 CrossRef CAS PubMed.
- A. K. Gnanappa, D. P. Papageorgiou, E. Gogolides, A. Tserepi, A. G. Papathanasiou and A. G. Boudouvis, Plasma Processes Polym., 2012, 9, 304 CrossRef CAS PubMed.
- M. Tang, M. H. Hong, Y. S. Choo, Z. Tang and D. H. C. Chua, Appl. Phys. A: Mater. Sci. Process., 2010, 101, 503 CrossRef CAS.
- V. Zorba, E. Stratakis, M. Barberoglou, E. Spanakis, P. Tzanetakis and C. Fotakis, Appl. Phys. A: Mater. Sci. Process., 2008, 93, 819 CrossRef CAS.
- N. J. Shirtcliffe, G. McHale, M. I. Newton and C. C. Perry, Langmuir, 2003, 19, 5626 CrossRef CAS.
- H. Budunoglu, A. Yildirim, M. O. Guler and M. Bayindir, ACS Appl. Mater. Interfaces, 2011, 3, 539 CAS.
- X. Zhang, F. Zheng, L. Ye, P. Xiong, L. Yan, W. Yang and B. Jiang, RSC Adv., 2014, 4, 9838 RSC.
- Y. Peng, K. Lo and Y. Juang, Langmuir, 2010, 26, 5167 CrossRef CAS PubMed.
- K. A. Manjumol, L. Mini, A. P. Mohamed, U. S. Hareesh and K. G. K. Warrier, RSC Adv., 2013, 3, 18062 RSC.
- H. M. Shang, Y. Wang, K. Takahashi and G. Z. Cao, J. Mater. Sci., 2005, 40, 3587 CrossRef CAS PubMed.
- J. Fang, H. You, P. Kong, Y. Yi, X. Song and B. Ding, Cryst. Growth Des., 2007, 7, 864 CAS.
- Y. Sun and Y. Xia, J. Am. Chem. Soc., 2004, 126, 3892 CrossRef CAS PubMed.
- J. Fang, X. Ma, H. Cai, X. Song and B. Ding, Nanotechnology, 2006, 17, 5841 CrossRef CAS.
- W. Xu, X. Shi and S. Lu, Mater. Chem. Phys., 2011, 129, 1042 CrossRef CAS PubMed.
- I. A. Larmour, S. E. J. Bell and G. C. Saunders, Angew. Chem., Int. Ed., 2007, 46, 1710 CrossRef CAS PubMed.
- Q. M. Pan and M. Wang, ACS Appl. Mater. Interfaces, 2009, 1, 420 CAS.
- B. Qian and Z. Shen, Langmuir, 2005, 21, 9007 CrossRef CAS PubMed.
- H. Liu, S. Szunerits, W. Xu and R. Boukherroub, ACS Appl. Mater. Interfaces, 2009, 6, 1150 Search PubMed.
- H. Liu, S. Szunerits, M. Pisarek, W. Xu and R. Boukherroub, ACS Appl. Mater. Interfaces, 2009, 9, 2086 Search PubMed.
- C. D. Wagner, W. M. Riggs, L. E. Davis and J. F. Moulder, Handbook of X-ray Photoelectron Spectroscopy, 1993 Search PubMed.
- S. A. Bocanegraa, A. Guerrero-Ruizb, S. R. de Miguela and O. A. Scelza, Appl. Catal., A, 2004, 277, 11 CrossRef PubMed.
- E. McCafferty, Corros. Sci., 2005, 47, 3202 CrossRef CAS PubMed.
- X. Shi, S. Lu and W. Xu, Mater. Chem. Phys., 2012, 134, 657 CrossRef CAS PubMed.
- J. P. Enríquez and X. Mathew, J. Mater. Sci.: Mater. Electron., 2005, 16, 617 CrossRef PubMed.
- J. G. Son, K. W. Gotrik and C. A. Ross, ACS Macro Lett., 2012, 1, 1279 CrossRef CAS.
- K. H. Westmacott, R. E. Smallman and P. S. Dobson, Met. Sci. J., 1968, 2, 177 CrossRef CAS PubMed.
- R. N. Wenzel, Ind. Eng. Chem., 1936, 28, 988 CrossRef CAS.
- A. B. D. Cassie and S. Baxter, Trans. Faraday Soc., 1944, 40, 546 RSC.
|
This journal is © The Royal Society of Chemistry 2014 |