DOI:
10.1039/C4RA06023G
(Paper)
RSC Adv., 2014,
4, 35415-35421
Silicate-based multifunctional nanostructured materials with magnetite and Prussian blue: application to cesium uptake
Received
20th June 2014
, Accepted 31st July 2014
First published on 1st August 2014
Abstract
Multifunctional nanostructured materials with superparamagnetic and adsorbent properties towards cesium ions were developed by a simple procedure consisting of the one-pot synthesis of magnetite and Prussian blue nanoparticles (NPs) in the presence of a porous inorganic solid that acts as a support or nanoplatform simultaneously assembling both types of NPs. Sepiolite, a hydrated magnesium silicate with fibrous morphology, microporosity, large specific surface area and bearing hydroxyl groups in its external surface, was here chosen as the nanoplatform supporting the in situ synthesized NPs, although this methodology was also successfully proved using other inorganic solids like silica gel. The efficacy of the resulting materials in the adsorption of cesium ions from aqueous media, even in the presence of a high concentration of sodium chloride, would allow their application in the removal of radioactive cesium-137 with the advantage of an easy and quick recovery of the pollutant-loaded adsorbents by means of a magnet.
Introduction
The pollution of soil and water by radioactive species like cesium-137 produced during nuclear fission and accidentally liberated in nuclear disasters (e.g., Chernobyl, Fukushima) or in routine operations in nuclear power plants, represents a serious environmental problem. In fact, these isotopes can easily pass to living beings and become a severe health risk for humans. Prussian blue (PB) and analogous hexacyanoferrate compounds are well-known adsorbents for the selective removal of cesium ions,1–3 even from living organisms in the case of the non-toxic PB.3–5 These compounds are prepared by precipitation of iron or other transition metal ions with hexacyanoferrate anions,6,7 being frequently synthesized on diverse types of supports in order to overcome the problems related to the fragility and the small particle size of the resulting crystals. Among these supports, ion-exchange resins,8,9 activated carbon,10 mesoporous silica11–13 and silicates14–16 have been commonly employed. Similarly, iron oxide particles have been used as support of hexacyanoferrates in order to profit from their magnetic properties in the separation process.17–19 Among them, iron oxide nanoparticles (NPs) provided with superparamagnetic properties, i.e. showing magnetic behavior only in the presence of an external magnetic field, resulted very useful as they allowed the easy recovery of the pollutant-loaded adsorbents with the help of electromagnets. This makes the separation process easier and less time consuming than column separation, filtration or centrifugation processes. Thus, magnetite NPs coated with nickel hexacyanoferrate were applied to the selective recovery of cesium ions showing fast exchange kinetics and being effective in the presence of high concentration of sodium nitrate.17 Prussian blue modified magnetite NPs were also reported as effective adsorbents in the uptake of cesium ions in the presence of NaCl,19 showing a maximum amount of adsorbed cesium ions of 16 g kg−1 of adsorbent. Nano-aggregates of hexacyanoferrate-loaded magnetite formed by mixing nickel-modified NPs with NPs previously modified with hexacyanoferrate anions were also applied in the removal of cesium ions by means of a magnetic separator column.18 In other cases, the magnetite NPs were dispersed within a polymer together with the PB as cesium adsorbent.20,21
In the present work, an alternative approach is proposed, in which PB and magnetite NPs are bonded to an inorganic solid provided with high surface area to produce superparamagnetic materials for selective Cs+ removal. These silicate-based multifunctional nanoplatforms are prepared through a recently patented one-pot synthesis with a sequential addition of reagents,22 in which the magnetite NPs are synthesized in the presence of an inorganic support of large specific surface area, followed by precipitation of the PB crystals in the same reactor. This method is completely accomplished in aqueous media, avoiding the use of organic solvents and oleic acid that were previously employed in the modification of analogous porous solids with ferrofluids to produce superparamagnetic adsorbents.23,24 The synthesis of PB was carried out following the protocol reported by Neff25 in which PB films are spontaneously formed from a solution of the reddish ferric ferricyanide complex on conducting and semiconducting substrates like platinum foil,25 and gold26 or magnetite NPs.27 The proposed mechanism involved the reduction of Fe3+ to Fe2+ and the subsequent precipitation of the PB as ferrous ferricyanide, with the conducting and semiconducting substrates most likely acting as catalysts.28
Sepiolite, a hydrated magnesium silicate with fibrous morphology, was used in this work as support of the magnetite and PB NPs. This silicate of theoretical unit cell formula Si12O30Mg8(OH,F)4(H2O)4·8H2O is characterized for its large specific surface area, ca. 320 m2 g−1, its microporosity, which results from an exceptional structure consisting in an alternation of blocks and tunnels that grow up in the fiber direction, and for the presence of silanol groups (Si–OH) located at the external edges of the blocks, which can interact with organic and inorganic species or can be used for the covalent grafting of functional groups.29,30 The high specific surface area and the microporosity of the inorganic support can favor the immobilization of the synthesized NPs, as recently reported for the preparation of magnetic zeolite,31 together with the presence of hydroxyl groups on the sepiolite surface. In comparison to those systems with the adsorbent particles entrapped within a polymer, the accessibility of the cesium ions to the adsorbent in these porous sepiolite-based platforms can be considerably increased as they offer an elevated active surface (Fig. 1). Other inorganic solids like silica gel were also tested as support of PB and magnetite for comparison. The efficiency of these multifunctional nanostructured materials in the uptake of cesium ions from aqueous media was evaluated, profiting from the easy recovery of the pollutant-loaded platforms by means of a magnet.
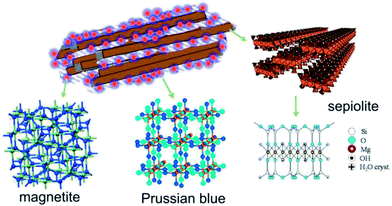 |
| Fig. 1 Schematic representation of the developed silicate-based multifunctional nanostructured materials. | |
Experimental section
Starting materials and reagents
Sepiolite used in this work is commercialized by TOLSA, S.A. as Pangel® S9. FeCl3·6H2O (99%), FeSO4·7H2O (99%), NaCl (98%) and HCl (ACS reagent, 37%) were supplied by Sigma-Aldrich, while K3Fe(CN)6 was purchased from Carlo Erba, and ammonium hydroxide solution (purum, ∼28% NH3 in H2O) from Fluka. Silica gel Merck 60 (particle size 0.015–0.040 mm) and CsCl were provided by Merck. Bidistilled water with resistivity of 18.2 MΩ cm was obtained with a Maxima Ultrapure Water system from Elga.
Synthesis procedure
The superparamagnetic material based on sepiolite incorporating magnetite nanoparticles and Prussian blue was prepared by the one-pot method patented by Ruiz-Hitzky et al.:22 a mixture of 2.43 g of FeCl3·6H2O and 1.67 g of FeSO4·7H2O was dissolved in 20 mL of bidistilled water and heated at 90 °C under mechanical stirring at 160 rpm using an overhead stirrer supplied with a homemade glass propeller. Then, an aqueous suspension (20 mL) containing 1.0 g of sepiolite was added to the mixture of the iron salts, followed by the addition of 6 mL of 25% NH4OH that turns the color of the suspension into black. After 5 min, the PB was formed by adding 80 mL of a solution containing 0.1 M FeCl3·6H2O and 0.1 M HCl to the previous mixture, followed by the addition of 80 mL of 0.1 M K3Fe(CN)6 also prepared in 0.1 M HCl. The dispersion was kept under mechanical stirring at 90 °C for 3 h, and then the solid was recovered by means of an iron–neodymium magnet, and washed with bidistilled water until obtaining a colorless supernatant. The recovered solid was allowed to dry at room temperature for 24 h, and finally grinded in an agate mortar. The sample was denoted as Sep/NPPB. For comparison, a sample without PB was prepared and labeled as Sep/NP.
Analogous materials were prepared following the same procedure but using 0.5 g of sepiolite, being this sample denoted as Sep-2/NPPB, or alternatively replacing the sepiolite by 1.0 g of silica gel Merck 60, which gave a sample that was called IM60NPPB. For comparison, the sample Sep/NP was used also as support of PB formed in a second step by adding 80 mL of 0.1 M FeCl3·6H2O and 80 mL of 0.1 M K3Fe(CN)6, both prepared in 0.1 M HCl, to a suspension containing 2 g of Sep/NP, and keeping this mixture under mechanical stirring at room temperature for 30 min. This sample was labeled as Sep/NPPB-2steps.
Characterization techniques
Fourier transform infrared spectroscopy (FTIR) was performed in a Bruker IFS 260 66v/S spectrophotometer. The samples were prepared as pellets in KBr and the spectra were recorded in the 4000–250 cm−1 wavenumber range with 2 cm−1 resolution. Powder X-ray diffraction (XRD) data were collected in a Bruker D8 T2T Advance diffractometer using a Cu-Kα source, with a scan step of 2° min−1 between 2–80° 2θ values. Transmission electron microscopy was performed with a LEO-910 microscope operating at 80 kV and with a JEOL 2000 FXII microscope working at 200 kV. Energy Dispersive X-ray (EDX) microanalysis was carried out in a FE-SEM equipment FEI-NOVA NanoSEM 230 coupled to a microanalysis system from EDAX. The particle samples were adhered on a carbon tap for direct observation without applying any conductive coating on their surface. VSM measurements were carried out at room temperature applying a variable magnetic field from −15
000 to 15
000 Oe in a vibrating sample magnetometer with an ADE magnetic system (model EV7). Measurements were carried out using powder samples.
Adsorption tests
The superparamagnetic samples were evaluated in the uptake of Cs+ ions. In order to optimize the adsorption time, a weighed amount of each sample (20 mg) was added to 20 mL of 2 mM CsCl solution and the mixture was kept in a shaker at room temperature and variable time. Then, the solid was separated from solution with the help of a magnet, and the amount of Cs+ ions in the supernatant was determined by inductively coupled plasma optical emission spectroscopy (ICP-OES), using the Perkin Elmer Optima 2100 DV ICP System.
In order to determine the maximum amount of Cs+ ions adsorbed by the superparamagnetic samples, weighed amounts of each sample (20 mg) were added to 20 mL of Cs+ solutions with concentration ranging from 0.1 to 10 mM. The mixture was kept in a shaker at room temperature for 3 h, and the amount of Cs+ ions remaining in the supernatant were determined by ICP-OES after separating the solid with a magnet. The possible interference of sodium ions was evaluated by carrying out the described adsorption procedure, but using a 2 mM Cs+ solution containing 0.3 M NaCl.
The adsorption capacity of the superparamagnetic materials was calculated using eqn (1), where q is the amount of cesium adsorbed per unit of adsorbent (mg g−1), C0 is the initial concentration of Cs+ ions (mg L−1), Ce is the Cs+ concentration at the adsorption equilibrium (mg L−1), V is the volume of the solution (L), and m is the weight of the adsorbent material (g).
|
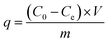 | (1) |
Results and discussion
The presence of magnetite nanoparticles together with Prussian blue crystals grown on the sepiolite substrate was confirmed by diverse techniques. TEM images of the samples are shown in Fig. 2. In contrast to the agglomeration and consequent loss of the superparamagnetic properties on individual NPs and the large inhomogenity in size observed in the NPPB system prepared in absence of sepiolite (Fig. 2a), the image of Sep/NP (Fig. 2b) shows the distribution on the sepiolite support of magnetite NPs of average diameter around 7.7 nm. The presence of the silicate fibers during the formation of magnetite seems to minimize the typical agglomeration of NPs found in the absence of surfactants, making possible the presence of individual nanoparticles or small groups of nanoparticles attached to the fibrous support of interest due to their potential superparamagnetic behaviour. The TEM image of the Sep/NPPB sample prepared in one step is shown in Fig. 2c, revealing that the particle size of magnetite in this case is slightly lower than in Sep/NP, showing an average diameter around 5.6 nm. This effect can be due to differences in the synthesis of Sep/NPPB sample, as the formation of the supported magnetite was only allowed for 5 minutes before addition of the reagents for PB precipitation. Fig. 2d shows a detail of one of the magnetite nanoparticles supported on the clay fiber, showing the crystallinity of this material, in which it is possible to appreciate a coverage on both the nanoparticle and the fibre that could be attributed to the deposited PB as small nanocrystals.
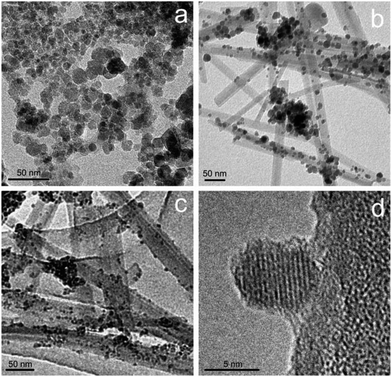 |
| Fig. 2 TEM images of the samples (a) NPPB, (b) Sep/NP, and (c and d) Sep/NPPB. | |
X-ray diffractograms (Fig. 3) showed the patterns of the pristine crystalline compounds as well as those observed after assembling the magnetite and PB nanoparticles to the porous support. In fact, the diffraction pattern of the sample Sep/NPPB (Fig. 3e) showed the reflections corresponding to the silicate and those of magnetite NPs together with two sharp peaks at 17.6 and 24.8 2θ° (0.50 and 0.36 nm, respectively) and a weak peak at 39.4 2θ° (0.22 nm) that can be clearly attributed to the PB nanocrystals assembled to the Sep/NP system. The reflection at 35.6 2θ° (0.25 nm) due to the supported PB cannot be easily identified as it is overlapped mainly by the most intense (311) reflection peak corresponding to magnetite. The presence of other iron oxide phases like maghemite cannot be discarded as the corresponding XRD patterns are very similar.
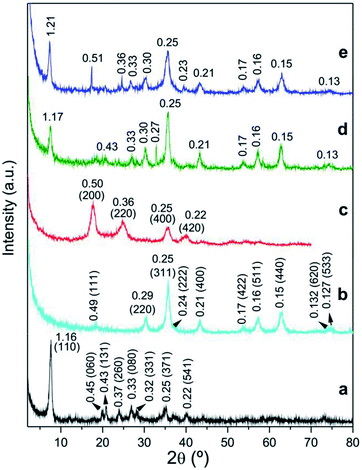 |
| Fig. 3 XRD patterns of (a) sepiolite, (b) magnetite, (c) PB, (d) Sep/NP, and (e) Sep/NPPB. | |
FTIR clearly confirmed the incorporation of the PB particles in the one-pot synthesis of Sep/NPPB, as the spectrum of this sample shows an intense band at 2073 cm−1 assigned to the C
N stretching vibration in the hexacyanoferrate units (Fig. 4). Two bands at 596 and 473 cm−1, which could be attributed to Fe–C
N–Fe bending vibration modes in PB,6 can be also observed in the spectrum of Sep/NPPB, appearing partially overlapped with those bands around 600–460 cm−1 attributed to the Fe–O stretching vibration in magnetite. In the spectrum of pristine PB, the sharp band at 500 cm−1 showed a shoulder at 514 cm−1, as already observed in the spectra of both soluble and insoluble PB prepared in the presence of HCl.6 The spectra of Sep/NP and Sep/NPPB show also the intense bands centered at 1025 cm−1 due to the Si–O–Si vibration modes in the crystalline structure of sepiolite, as well as the antisymmetric and symmetric O–H stretching vibrations around 3600–3000 cm−1 and the H–O–H bending vibration mode at 1606 cm−1 that confirm the presence of associated water. The ammonium ions used in the synthesis of magnetite nanoparticles remain adsorbed on sepiolite, as suggested by the sharp band observed at around 1410 cm−1 in the spectra of both samples Sep/NP and Sep/NPPB.
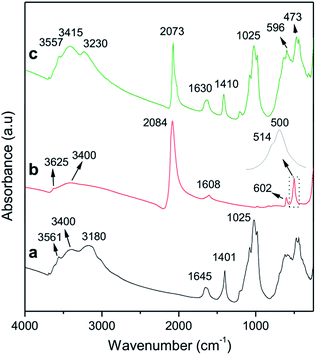 |
| Fig. 4 FTIR spectra in the 4000–250 cm−1 range of the samples (a) Sep/NP, (b) PB, and (c) Sep/NPPB. | |
The magnetic properties of the samples were evaluated by VSM. Fig. 5 shows a comparative study of the prepared samples in order to determine their behavior in the presence of an external magnetic field. The presence of superparamagnetic particles in Sep/NPPB can be advantageous for the easy recovery of the adsorbents after the Cs+ uptake from aqueous solution by means of the application of an external magnetic field. Thus, the Sep/NPPB materials should exhibit the superparamagnetic character of the magnetite nanoparticles showing a high value of saturation magnetization (Ms) when the field is applied, and a value of coercive field (Hc) equal to zero when removing the external field. All the magnetization curves corresponding to NP, NPPB, Sep/NP and Sep/NPPB materials (Fig. 5) show the value Hc = 0, clearly indicating the superparamagnetic behavior of each material in good relationship with the small particle size of the magnetite NPs. The saturation magnetization oscillates between 49.5 emu g−1 for the NPs of pure magnetite (Fig. 5a) to 27.3 emu g−1 for the Sep/NPPB system (Fig. 5c) due to the variable content of NPs in each system, but in all cases the superparamagnetic behavior of these materials would allow their easy recovery with the help of a magnet after the uptake of cesium ions. The lower value obtained in the saturation magnetization of magnetite NPs with respect to that reported for pure magnetite could be ascribed to the presence of other iron oxide phases such as maghemite.
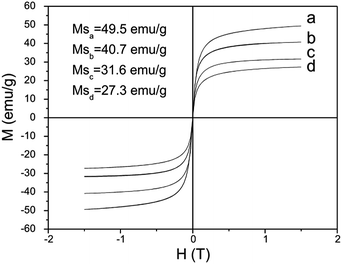 |
| Fig. 5 VSM characterization of the samples (a) magnetite NPs, (b) NPPB, (c) Sep/NP and (d) Sep/NPPB. | |
Cesium removal
The multifunctional materials were evaluated as adsorbents for the removal of cesium ions from aqueous solution. A comparison of the adsorption ability of the synthesized materials is shown in Fig. 6. The assembly of both PB and magnetite nanoparticles in the sample Sep/NPPB contributed to a remarkable adsorption property, with the addition of the superparamagnetic character that allows an easy recovery of the adsorbent. The presence of the solid support seems to be important, as the system NPPB prepared in the absence of sepiolite showed a lower retention value than Sep/NPPB, even when it has a slightly higher amount of deposited PB (about 30% in weight) in contrast to the 20% determined in Sep/NPPB by EDX measurements. Similarly, a sample prepared with a lower amount of this sepiolite platform (Sep-2/NPPB) shows lower retention values, around 25 mg Cs+ per g, in spite of having a higher content in PB, also close to 30%. In those cases the accessibility of Cs+ ions to the PB nanocrystals may be reduced as the NPPB system becomes agglomerated due to the less available platform surface for their optimal distribution (Sep-2/NPPB) or to the absence of the clay support (NPPB). In addition, the silicate support could contribute to the cesium uptake, as pristine sepiolite affords a retention of 4.4 mg Cs+ per g of clay. The one-pot synthetic procedure seems to be also a key factor in the final properties, since a similar material prepared in two steps (i.e. depositing the PB nanoparticles on previously prepared Sep/NP) yielded a lower capacity to adsorb cesium ions, around 40% below the capacity of the sample prepared by the one-pot method, and being even lower than that of a sepiolite-based material containing half the amount of sepiolite than the sample Sep/NPPB. Other porous inorganic solids like silica gel acting as support of magnetite and PB in place of sepiolite and prepared in a one-step method can also afford good performance in cesium removal, being able to adsorb around 26 mg g−1 of Cs+, which is a value close to those obtained with the sepiolite-based samples.
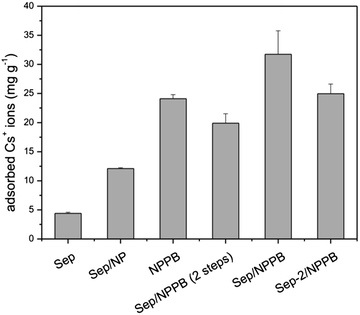 |
| Fig. 6 Comparison of several materials in the adsorption of cesium ions. About 20 mg of each material were added to 20 mL of 2 mM CsCl solution and kept in contact for 24 h. | |
The uptake of Cs+ ions was also evaluated in the presence of Na+ ions, which can be an important interference in the removal of cesium from sea water. The adsorption process was carried out using a 2 mM CsCl solution implemented with 0.3 M NaCl. In both the sepiolite-based material Sep/NPPB and the silica gel-based sample, the amount of adsorbed Cs+ was around 32% lower than that obtained in the absence of the interfering ion. This is a good result considering that neat iron hexacyanoferrate shows a similar level of interference under the same conditions, with a decrease in the Cs+ uptake from 80 mg g−1 to 60 mg g−1, i.e. 25%.
The study was then focused on Sep/NPPB, as this sample showed the best performance in the retention of cesium ions. The adsorption kinetics was evaluated by using a 2 mM CsCl solution, following the procedure described in the Experimental Section. Fig. 7 shows a fast adsorption of Cs+ ions by Sep/NPPB within the first 15 minutes of contact with the cesium solution, which reveals a similar behavior than other reported systems of metal-hexacyanoferrates loaded on magnetite17 or on mesoporous silica.11 The adsorbed amount of Cs+ ions reached a constant value after ca. 30 min of contact with the cesium solution. The best fit of the kinetic data was achieved with a pseudo-second order model.32 The linear form of this model is described by the following equation:
|
t/qt = 1/(k2qe2) + (1/qe) × t
| (2) |
where
qe and
qt (mg g
−1) represent the adsorbed amount at equilibrium and at a given time, respectively, and
k2 is the rate constant of pseudo-second order sorption (g mg
−1 min
−1). The fitting of the experimental data to the pseudo-second order model yielded a good correlation coefficient (
r = 0.9998), and allowed to determine the constants
qe = 41.5 mg g
−1 and
k2 = 0.012 g mg
−1 min
−1. From these values, an initial sorption rate of 21.3 mg g
−1 min
−1 was calculated using the equation
h =
k2qe2.
31
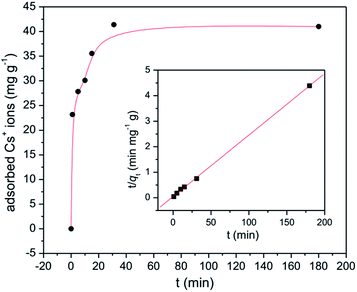 |
| Fig. 7 Adsorption kinetics of the removal of Cs+ ions by Sep/NPPB. The inset shows the fitting of the data to the pseudo-second order kinetic model. | |
The adsorption values obtained at 295 K by varying the starting concentration of cesium ions were fitted to the Langmuir model (Fig. 8), yielding a maximum adsorption capacity close to 102 mg g−1 and an adsorption constant of 0.0024 L g−1. The Gibbs free energy of adsorption could be determined from the thermodynamic equilibrium constant (K0), which is calculated from the limiting value of the ratio Cs/Ce (K0) by extrapolating a plot of Cs/Ce against Cs to Cs = 0, being Cs the concentration of adsorbed cesium (mmol g−1), and Ce the Cs+ concentration in equilibrium solution (mmol mL−1).33 The resulting free energy was estimated to be −14.5 kJ mol−1, typical of a physisorption process.
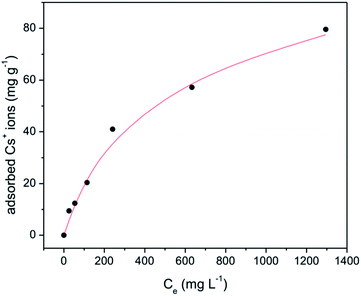 |
| Fig. 8 Adsorption isotherm on Sep/NPPB of Cs+ ions from aqueous solution at 295 K. | |
For comparison, Table 1 summarizes the adsorption capacity values of related materials found in referenced works. The multifunctional materials here developed showed a higher adsorption capacity than that obtained for the PB–magnetite system reported by Sasaki and Tanaka (16.2 mg g−1),19 but still lower than that of the nickel hexacyanoferrate–magnetite system, which was reported to adsorb around 1000 mg g−1 of cesium ions.17 However, this last material has the disadvantage of involving hexacyanoferrates with toxic metals rather than the harmless PB. Considering those systems in which the metal-hexacyanoferrate is grown on other supports, the efficiency of the Sep/NPPB material in the cesium removal seems to be also superior than that of systems based on ion-exchange resins,34 activated carbon,10 latex nanoparticles,35 or microporous silica.13 In contrast, other reported systems involving nickel-hexacyanoferrate/chabazite16 and mesoporous silica as support of nickel12 and copper11 hexacyanoferrates afforded an adsorption capacity of about twice that obtained with the Sep/NPPB material. However, all these systems have the disadvantages of lacking of magnetic character and of involving toxic metals, while PB has been reported as a non-hazardous compound being applied in decorporation of radiocesium.4
Table 1 Adsorption capacity values of other reported systems based on metal-hexacyanoferrate grown on different supports
Adsorbent |
Support |
Adsorption capacity (mg g−1) |
Reference |
FeIII hexacyanoferrate (PB) |
Magnetite |
16.2 |
Sasaki and Tanaka, 2012 (ref. 19) |
NiII hexacyanoferrate |
Magnetite |
1000 |
Ambashta et al., 2006 (ref. 17) |
CuII hexacyanoferrate |
Ion exchange resin |
12.4–16.1 |
Singh and Ramaswamy, 1991 (ref. 34) |
NiII hexacyanoferrate |
Chabazite |
191–249 |
Mimura et al., 1999 (ref. 16) |
CuII hexacyanoferrate |
Activated carbon |
61.1 |
Wang et al., 2009 (ref. 10) |
CuII, NiII, and CoII hexacyanoferrates |
Latex nanoparticles |
2.6–5.5 |
Avramenko et al., 2011 (ref. 35) |
NiII hexacyanoferrate |
Mesoporous silica |
225 |
Chang et al., 2008 (ref. 12) |
CuII hexacyanoferrate |
Mesoporous silica |
179 |
Lin et al., 2001 (ref. 11) |
CuII hexacyanoferrate |
Mesoporous silica |
17.1–21.7 |
Sangvanich et al., 2010 (ref. 13) |
After cesium uptake, the sample Sep/NPPB was evaluated by means of FESEM-EDX, which provided the composition of the sample (Fig. 9). From these results, the existence of 27.0% of sepiolite, 44.1% of magnetite, and 18.3% of PB can be estimated in Sep/NPPB together with a 7.14% of adsorbed cesium ions and about 3.4% of water. The mapping of a given area of Sep/NPPB showed a homogeneous distribution of all the evaluated elements along the adsorbent, pointing out to the homogeneity of the magnetite and PB nanoparticles on the sepiolite support. Consequently, the expected uniform dispersion of Cs+ ions on this area of the adsorbent was also clearly observed. The EDX analysis confirmed the adsorption of 7.14% wt of Cs+ ions, which was in agreement with the value determined for this sample by means of ICP-OES (7.37% wt).
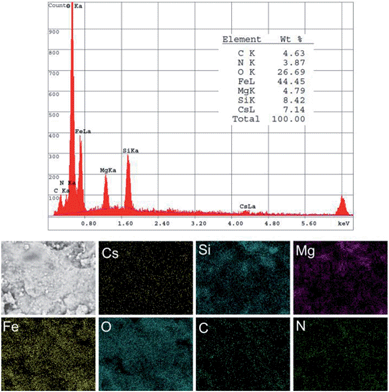 |
| Fig. 9 EDX analysis and mapping of an area of Sep/NPPB after adsorption of cesium ions, showing the distribution of each element. The FESEM image of the analyzed area is included together with the maps of the elements. | |
Conclusions
The preparation, characterization, and application of multifunctional nanostructured materials provided with superparamagnetic and adsorbent properties were here reported. Magnetite nanoparticles were successfully grown in the presence of the sepiolite silicate as porous support, following a procedure that avoids the use of organic solvents and oleic acid. Then, precipitation of Prussian blue, a non-toxic iron hexacyanoferrate, was carried out in the same reactor. The presence of both magnetic and adsorbent particles was confirmed by several characterization techniques like XRD, FTIR, and EDX, and the magnetic properties were determined by VSM measurements. These multifunctional materials were proven to be effective adsorbents for the removal of cesium ions from aqueous solution, even in the presence of sodium ions, with the advantage of allowing an easy recovery of the cesium-loaded materials with the help of a magnet.
Acknowledgements
This work was supported by the CICYT, Spain (project MAT2012-31759). The authors thank Mr A. Valera and Mr C. Sebastián for the FESEM-EDX studies and to Mr J. González Casablanca for the TEM images.
Notes and references
- J. Benes and M. Kyrs, Anal. Chim. Acta, 1963, 29, 564 CrossRef CAS
. - C. Loos-Neskovic, S. Ayrault, V. Badillo, B. Jimenez, E. Garnier, M. Fedoroff, D. J. Jones and B. Merinov, J. Solid State Chem., 2004, 177, 1817 CrossRef CAS PubMed
. - P. J. Faustino, Y. Yang, J. J. Progar, C. R. Brownell, N. Sadrieh, J. C. May, E. Leutzinger, D. A. Place, E. P. Duffy, F. Houn, S. A. Loewke, V. J. Mecozzi, C. D. Ellison, M. A. Khan, A. S. Hussain and R. C. Lyon, J. Pharm. Biomed. Anal., 2008, 47, 114 CrossRef CAS PubMed
. - Use of Prussian blue (Ferric Hexacyanoferrate) for Decorporation of Radiocaesium, http://www.hpa.org.uk/webc/HPAwebFile/HPAweb_C/1274091555595, accessed on December 2013 Search PubMed.
- E. F. Unsworth, J. Pearce, C. H. McMurray, B. W. Moss, F. J. Gordon and D. Rice, Sci. Total Environ., 1989, 85, 339 CrossRef CAS
. - R. E. Wilde, S. N. Ghosh and B. J. Marshall, Inorg. Chem., 1970, 9, 2512 CrossRef CAS
. - J. B. Ayers and W. H. Waggoner, J. Inorg. Nucl. Chem., 1971, 33, 721 CrossRef CAS
. - K. Watari, K. Imai and M. Izawa, J. Nucl. Sci. Technol., 1967, 4, 190 CrossRef CAS
. - H.-J. Won, J.-K. Moon, C.-H. Jung and W.-Y. Chung, Nucl. Eng. Technol., 2008, 40, 489 CrossRef CAS
. - L. Wang, M. Feng, C. Liu, Y. Zhao, S. Li, H. Wang, L. Yan, G. Tian and S. Li, Sep. Sci. Technol., 2009, 44, 4023 CrossRef CAS
. - Y. H. Lin, G. E. Fryxell, H. Wu and M. Engelhard, Environ. Sci. Technol., 2001, 35, 3962 CrossRef CAS
. - C.-Y. Chang, L.-K. Chau, W.-P. Hu, C.-Y. Wang and J.-H. Liao, Microporous Mesoporous Mater., 2008, 109, 505 CrossRef CAS PubMed
. - T. Sangvanich, V. Sukwarotwat, R. J. Wiacek, R. M. Grudzien, G. E. Fryxell, R. S. Addleman, C. Timchalk and W. Yantasee, J. Hazard. Mater., 2010, 182, 225 CrossRef CAS PubMed
. - D. Gournis, C. Papachristodoulou, E. Maccallini, P. Rudolf, M. A. Karakassides, D. T. Karamanis, M.-H. Sage, T. T. M. Palstra, J.-F. Colomer, K. D. Papavasileiou, V. S. Melissas and N. H. Gangas, J. Colloid Interface Sci., 2010, 348, 393 CrossRef CAS PubMed
. - H. Mimura, M. Kimura, K. Akiba and Y. Onodera, J. Nucl. Sci. Technol., 1999, 36, 307 CrossRef CAS
. - H. Mimura, M. Kimura, K. Akiba and Y. Onodera, Sep. Sci. Technol., 1999, 34, 17 CrossRef CAS PubMed
. - R. D. Ambashta, D. S. Deshingkar, P. K. Wattal and D. Bahadur, J. Radioanal. Nucl. Chem., 2006, 270, 585 CrossRef CAS
. - R. D. Ambashta, P. K. Wattal, S. Singh and D. Bahadur, J. Magn. Magn. Mater., 2003, 267, 335 CrossRef CAS
. - T. Sasaki and S. Tanaka, Chem. Lett., 2012, 41, 32 CrossRef CAS
. - D. Bradbury, G. R. Elder and A. T. S. A. Hendawi, US Pat., EP0666577B1, WO95/21011, 1995
. - D. Bradbury, P. M. Tucker and G. R. C. Elder, US Pat., EP0522856B1, 1993
. - E. Ruiz-Hitzky, P. Aranda, M. Darder and Y. González-Alfaro, Spain Pat., P201330062, 2013
. - E. Ruiz-Hitzky, P. Aranda and Y. González-Alfaro, Spain Pat., P201030333, 2010
; PCT ES2011/070145, 2011
. - Y. González-Alfaro, P. Aranda, F. M. Fernandes, B. Wicklein, M. Darder and E. Ruiz-Hitzky, Adv. Mater., 2011, 23, 5224 CrossRef PubMed
. - V. D. Neff, J. Electrochem. Soc., 1978, 125, 886 CrossRef CAS PubMed
. - J.-D. Qiu, H.-Z. Peng, R.-P. Liang, J. Li and X.-H. Xia, Langmuir, 2007, 23, 2133 CrossRef CAS PubMed
. - G. Zhao, J. J. Feng, Q. L. Zhang, S. P. Li and H. Y. Chen, Chem. Mater., 2005, 17, 3154 CrossRef CAS
. - D. Ellis, M. Eckhoff and V. D. Neff, J. Phys. Chem., 1981, 85, 1225 CrossRef CAS
. - E. Ruiz-Hitzky, J. Mater. Chem., 2001, 11, 86 RSC
. - Developments in Palygorskite-Sepiolite Research. A new Outlook on these Nanomaterials, ed.E. Galán and A. Singer, Elsevier B.V., Oxford, UK, 2011 Search PubMed
. - H. Faghihian, M. Moayed, A. Firooz and M. Iravani, J. Colloid Interface Sci., 2013, 393, 445 CrossRef CAS PubMed
. - Y. S. Ho and G. McKay, Process Biochem., 1999, 34, 451 CrossRef CAS
. - H. K. Boparai, M. Joseph and D. M. O'Carroll, J. Hazard. Mater., 2011, 186, 458 CrossRef CAS PubMed
. - I. J. Singh and M. Ramaswamy, Removal of cesium from low level waste solutions by copper hexacyanoferrate loaded resins, Report BARC/1991/E/022, 1991 Search PubMed
. - V. Avramenko, S. Bratskaya, V. Zheleznov, I. Sheveleva, O. Voitenko and V. Sergienko, J. Hazard. Mater., 2011, 186, 1343 CrossRef CAS PubMed
.
|
This journal is © The Royal Society of Chemistry 2014 |