DOI:
10.1039/C4RA05848H
(Paper)
RSC Adv., 2014,
4, 54109-54114
Continuous flow reactive distillation process for biodiesel production using waste egg shells as heterogeneous catalysts
Received
17th June 2014
, Accepted 9th October 2014
First published on 9th October 2014
Abstract
A laboratory scale continuous flow jacketed reactive distillation (RD) unit was developed to evaluate the performance of calcium oxide (CaO) derived from egg shells as a heterogeneous catalyst for transesterification of waste frying oil. The effects of reactant flow rate, methanol to oil ratio and catalyst bed height on methyl ester conversion were investigated. All the experimental investigations were carried out at atmospheric pressure and the reboiler temperature was maintained at 65 °C throughout the process. The results indicated that the system reached the steady state at 7 h. Egg shell based CaO showed high catalytic activity in a continuous flow jacketed reactive distillation system and a maximum methyl ester conversion of 93.48% was obtained at a reactant flow rate of 0.2 mL min−1, methanol–oil ratio of 4
:
1 and catalyst bed height of 150 mm.
Introduction
High feedstock cost is one of the major barriers to the expansion and application of biodiesel for commercial viability. Proper utilization of waste frying oil (WFO) originating from restaurants and household disposal as feedstock for biodiesel preparation can reduce the production costs. However WFO contains significant amounts of free fatty acids (FFA) which are highly sensitive to homogeneous alkaline catalysts such as sodium hydroxide (NaOH) and potassium hydroxide (KOH) leading to the formation of soap. Homogeneous base catalysts have several drawbacks such as difficulty in the recovery of catalyst from reactant product mixture, generation of excess wastewater and establishment of a continuous process.1,2 Usage of heterogeneous catalysts in the preparation of biodiesel can significantly decrease the purification steps and production cost.3,4 Another possible way to reduce the cost is to use waste materials (shells) as solid catalyst in biodiesel production. Waste shells of egg,5,6 clam,7 capiz,8 cockle,9 and Turbonilla striatula10 were calcined to obtain calcium oxide and used effectively as a heterogeneous catalyst in batch experiments for biodiesel production. Wei et al.5 utilized egg shells for transesterification of soybean oil and Niju et al.6 utilized egg shells for transesterification of waste frying oil. However there is no report on use of this catalyst in continuous processes for biodiesel production. Continuous production of biodiesel using resinous catalyst with high methanol to oil molar ratio in a packed bed reactor had been reported.4,11 These processes employed excess alcohol which must be recovered in additional separation process such as low pressure distillation which leads to increase in energy consumption and process time. In order to integrate the chemical reaction and product purification, a novel configuration which provides a more effective and efficient system is needed. Reactive distillation (RD) is the ideal candidate technology for continuous production of biodiesel which integrates the function of chemical reactions and separations simultaneously in a single unit. The excess/unreacted methanol was completely vaporized by the reboiler and the vapors were recirculated in the RD column and utilized in the reactive zone. Hence the methanol to oil ratio is reduced significantly and the additional methanol recovery unit used to separate biodiesel from methanol is avoided in the RD system. Prasertsit et al.12 performed transesterification of palm oil with methanol in the presence of potassium methoxide as homogeneous catalyst in reactive distillation column. Kiss et al.13 performed reactive distillation using metal oxides such as niobic acid, sulfated zirconia, sulfated titania and sulfated tin oxide and tested its applicability for biodiesel production and a very high reboiler temperature of 200 °C has been reported for the required process operation.
The aim of this work is to explore the applicability of a laboratory scale continuous flow jacketed reactive distillation (RD) process using egg shell based CaO as a heterogeneous base catalyst for transesterification of waste frying oil which helps to reduce the reboiler temperature for product purification. The effect of variables on transesterification process was also investigated.
Materials and methods
Materials
Waste frying oil (WFO) was used as a source for transesterification process. Non-oil component and solid content of the WFO were separated using a Whatman no. 41 filter paper of 125 mm diameter and pore size of 20 μm and the obtained oil was pretreated with activated carbon for the reduction of free fatty acids. The physiochemical properties of WFO were measured and presented in Table 1. Egg shells were washed, dried, reduced to small pieces and then calcined in a muffle furnace under static air conditions at 900 °C for 2.5 h in order to transform the calcium species in the shell into active CaO catalyst. CaO pieces (2–4 mm particle size) were stored in a closed vessel to avoid the reaction with CO2 and moisture in the air. Anhydrous methanol of analytical grade, purchased from Merck Limited, Mumbai, India was used in the transesterification reaction.
Table 1 Physicochemical properties of waste frying oil used in the present study
Properties |
Measured values |
Density at 25 °C (kg m−3) |
914 |
Kinematic viscosity at 40 °C (mm2 s−1) |
29.54 |
Acid value (mg of KOH per g of oil) |
2.24 |
Catalyst characterization
The CaO formation on calcination of the egg shell was confirmed by powder X-ray diffraction (XRD) using a Rigaku (Model: DMAX 2200/Ultima+) diffractometer equipped with Cu Kα radiation. Scanning Electron Microscopy (SEM) and Energy Dispersive Atomic X-ray Spectrometry (EDAX) were performed to confirm the morphology and composition of the catalyst using High Resolution Scanning Electron Microscope (Model: F E I Quanta FEG 200) equipped with EDAX. The surface area of egg shell based CaO was determined by BET analysis using ASAP 2020 surface area analyzer (Micromeritics).
Continuous transesterification in a reactive distillation set up
The continuous transesterification of WFO with methanol was performed in a jacketed reactive distillation (RD) system using egg shell based CaO as a heterogeneous catalyst. The most important advantages of RD system using heterogeneous catalyst is not only the recirculation of methanol in the reactive zone but also the simplicity of product separation. The scheme of the reactive distillation system is shown in Fig. 1. The system consists of a packed bed distillation column made up of glass (25 cm length × 2 cm inner diameter) equipped with a hot water jacket to maintain a constant temperature of 65 °C inside the packed column. The column was packed with glass beads of 1–2 mm diameter up to a certain height and the remaining portion of the column is loaded with egg shell based CaO of 2–4 mm particle size. The lower end of the column was connected to the middle neck of a 1 liter three-necked round bottomed flask which was used as a reboiler. The top of the column was connected with a water-cooled condenser to recover methanol and the unreacted reactants which was refluxed back to the system. Reboiler temperature was maintained at 65 °C throughout the process and the heat required for the reboiler is supplied by a constant temperature water bath. Methanol and oil were mixed thoroughly at a particular ratio in a feedstock tank using a magnetic stirrer and then fed to the upper part of the catalytic section of the column continuously through a peristaltic pump. Most of the methanol was being utilized in the reaction section itself (the region packed with the catalyst) and the unreacted methanol or excess methanol if any slowly flow down towards the end of the column. This methanol was completely vaporized by the reboiler and the vapors were recirculated in the RD column and utilized in the reactive zone. All the catalysts are retained in the column itself and hence the products collected in the reboiler include fatty acid methyl esters (FAMEs) and glycerol which clearly indicates that the reverse transesterification of FAME and glycerol is negligible. The product mixture was withdrawn from the reboiler using a peristaltic pump and sent to a glycerol-ester separator continuously. FAME was separated and characterized.
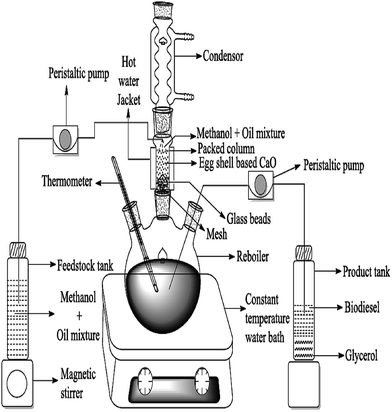 |
| Fig. 1 Scheme of the continuous transesterification reactive distillation system. | |
Analysis of the samples
The composition of the methyl esters was characterized using Perkin Elmer GC clarus 500 system and Gas Chromatograph interfaced to a mass spectrometer (GC-MS) equipped with Elite 5 fused silica capillary column composed of 5% diphenyl/95% dimethyl polysiloxane (30 × 0.25 mm × 0.25 μm df). The conversion of oil to FAME was determined by 1H Nuclear Magnetic Resonance Spectroscopy (1H NMR). Samples prepared in CDCl3 was recorded on a Bruker Avance III 500 MHz (AV 500) spectrometer. The relevant signals chosen for integration were those of methoxy group in the methyl esters at 3.7 ppm and the protons on the carbon next to the glyceryl moiety (α-CH2) at 2.3 ppm. eqn (1) given by Knothe14 has been employed to find the conversion. |
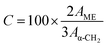 | (1) |
where C = percentage conversion of triglycerides to methyl esters, AME = integration value of the methoxy protons of the methyl ester, Aα-CH2 = integration value of the α-methylene protons.
Results and discussion
Catalyst characterisation
Crystalline structure of the catalyst was confirmed by XRD analysis and Fig. 2 depicts the XRD pattern of the egg shell based CaO. The peaks appeared at 2θ = 32.36°, 37.50°, 54.00°, 64.30°, 67.48° were the characteristics of CaO and the peaks appeared at 2θ = 18.16°, 34.34° were the characteristics of calcium hydroxide. From the BET analysis, the surface area of the egg shell based CaO was determined as 3.72 m2 g−1. Fig. 3 shows the SEM image of the egg shell based CaO. It expressed regular micro morphology of rod like particles with size ranges from 1.29 to 2.0 μm of width was observed. The elemental composition of the egg shell based CaO was performed by Energy Dispersive Atomic X-ray Fluorescence Spectrometer, which clearly indicates the presence of oxygen (45.42%, w/w) and calcium (54.58%, w/w).
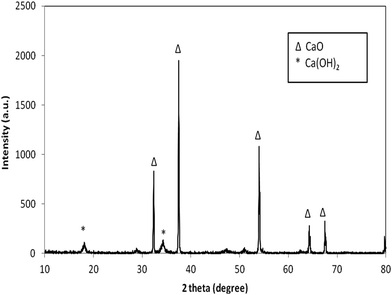 |
| Fig. 2 XRD of the egg shell based CaO catalyst. | |
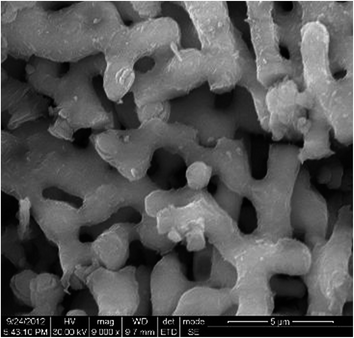 |
| Fig. 3 SEM image of the egg shell based CaO catalyst. | |
Effect of variables on methyl ester conversion
The effects of variables such as flow rate, methanol to oil ratio and catalyst bed height were investigated. The results are discussed in detail.
Steady state determination
The samples collected from the reboiler periodically was checked by 1H Nuclear Magnetic Resonance Spectroscopy (1H NMR) to confirm the presence of methyl ester. It was observed from the Fig. 4 that the system has reached steady state at 7 h. Thereafter the methyl ester conversion almost remained the same which ensure that steady state has attained.
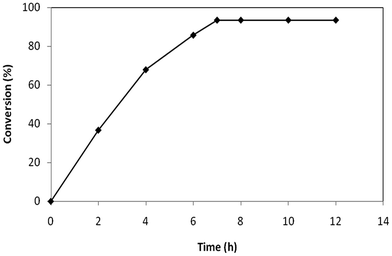 |
| Fig. 4 Effect of time on methyl ester conversion (flow rate = 0.2 mL min−1, height of the catalyst bed = 150 mm, methanol–oil ratio = 4 : 1). | |
Effect of flow rate
Reactant flow rate is one of the most important parameters in continuous flow RD system. Methyl ester conversion was increased from 84.26 to 93.48% with increased flow rate from 0.1 to 0.2 mL min−1 as shown in Fig. 5. Further increase in the feed flow rate resulted in lowering the methyl ester conversion. Conversion significantly decreased from 93.48 to 41.62% as the flowrate increased from 0.2 to 0.4 mL min−1. Therefore a maximum methyl ester conversion of 93.48% was achieved at a flow rate of 0.2 mL min−1. These results are in accordance with the findings of other researchers too.15,16 Liquid film formed on the catalyst surface at low feed flowrate improved the mass transfer resistance.15 An increase in the feed flowrate reduces the mass transfer limitation and results in higher methyl ester conversion.16 However, further increase in the flow rate or at very high flow rates, the residence time of the reactants in the catalyst column is decreased which results in a lower conversion.15
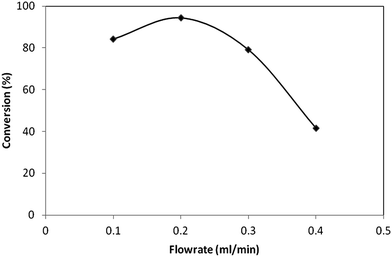 |
| Fig. 5 Effect of flow rate on methyl ester conversion (time = 7 h, height of the catalyst bed = 150 mm, methanol–oil ratio = 4 : 1). | |
Effect of catalyst bed height
Height of the catalyst bed is closely related to the residence time in continuous transesterification.17 Methyl ester conversion highly depends on the amount of catalyst loaded. The conversion was increased rapidly from 64.25 to 93.48% with increased catalyst bed height from 75 mm to 150 mm. It clearly indicates that a high catalyst bed height provides a longer reaction time and more active sites to promote the reaction between waste frying oil and methanol at a given flow rate.4
Effect of methanol to oil ratio
Methanol to oil molar ratio is one of the key parameters in a transesterification reaction.
Methanol to oil ratio was varied from 2
:
1 to 6
:
1 with the increment of 2
:
1. The methyl ester conversion was increased from 63.77 to 93.48% when the methanol to oil ratio was increased from 2
:
1 to 4
:
1 as shown in Fig. 6. This leads to reaction of methanol with the catalyst to form calcium methoxide during the early stage of reaction and subsequently formed calcium diglyceroxide with the glycerol produced by transesterification.18,19 Larger amount of methanol would facilitate the diffusion of reactants and the formation of calcium methoxide resulted in higher methyl ester content and a maximum conversion of 93.48% was obtained at a methanol to oil ratio of 4
:
1. Further increase in the methanol to oil ratio has no significant effect on methyl ester conversion. The unreacted methanol seems to be collected at the end of column towards the reboiler which is vaporized and recirculated through the catalyst bed. Moreover, the RD system operated with egg shell based CaO as solid catalyst reduced the methanol to oil ratio at 4
:
1 as compared to 9
:
1 in batch processes using egg shell based CaO5,6 and 6
:
1 in the conventional homogeneous catalyst processes.12 This helps to reduce the quantum of methanol about 55.55% compared to the batch processes and 33.33% compared to the conventional processes using homogeneous catalysts.
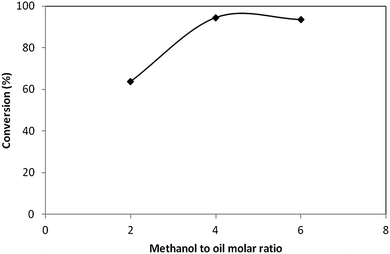 |
| Fig. 6 Effect of methanol to oil molar ratio on methyl ester conversion (time = 7 h, flow rate = 0.2 mL min−1, height of the catalyst bed = 150 mm). | |
Composition of methyl ester
The GC-MS analysis was performed to determine the chemical composition of the synthesized FAME. The gas chromatogram of the FAME is shown in Fig. 7. Interpretation on mass spectrum was conducted using the database of NIST. The spectrum of the unknown component was compared with the spectrum of the known components stored in the NIST library. The name, molecular weight and structure of the components were identified and reported in Table 2. In Fig. 7 the major components observed in chromatogram are tetradecanoic acid methyl ester, 7-hexadecenoic acid methyl ester, hexadecanoic acid methyl ester, 9,12-octadecadienoic acid (z,z) methyl ester, 9,12,15-octadecatrienoic acid (z,z,z) methyl ester, 9 octadecenoic acid (z) methyl ester, eicosanoic acid methyl ester, docosanoic acid methylester, tetracosanoic acid methyl ester at the retention time of 9.65, 11.51, 11.97, 14.14, 15.20, 16.72, 17.04, 19.63 and 22.20 minutes respectively.
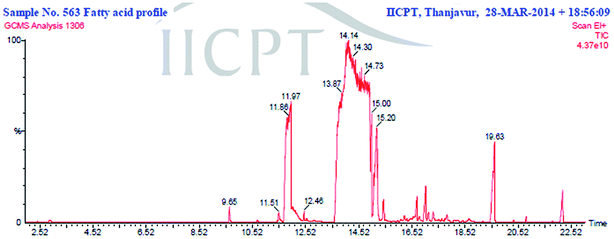 |
| Fig. 7 GC-MS spectrum of FAME. | |
Table 2 Chemical composition of the components in FAME
Retention time (min) |
Name of the compound |
Molecular formula |
Molecular weight |
9.65 |
Tetradecanoic acid methyl ester |
C15H30O2 |
242 |
11.51 |
7-Hexadecenoic acid methyl ester |
C17H32O2 |
268 |
11.97 |
Hexadecanoic acid methyl ester |
C17H34O2 |
270 |
14.14 |
9,12-Octadecadienoic acid (z,z) methyl ester |
C19H34O2 |
294 |
15.20 |
9,12,15-Octadecatrienoic acid (z,z,z) methyl ester |
C19H32O2 |
292 |
16.72 |
9 Octadecenoic acid (z) methyl ester |
C19H36O2 |
296 |
17.04 |
Eicosanoic acid methyl ester |
C21H42O2 |
326 |
19.63 |
Docosanoic acid methyl ester |
C23H46O2 |
354 |
22.20 |
Tetracosanoic acid methyl ester |
C25H50O2 |
382 |
Conversion of methyl ester formation
The conversion of oil to FAME was determined by 1H Nuclear Magnetic Resonance Spectroscopy (1H NMR). Fig. 8 represents the 1H NMR spectrum of the waste frying oil. The conversion of triglycerides to fatty acid methyl esters catalyzed by egg shell based CaO was analyzed using 1H NMR and it is shown in Fig. 9. The characteristic peaks of methoxy protons as a singlet at 3.68 ppm and α-methylene protons as a triplet at 2.317 ppm were observed. These two peaks are the distinct peaks for the confirmation of methyl esters.14 The other peaks observed were at 0.882 ppm, due to terminal methyl protons, a strong signal at 1.318 ppm arises from the methylene proton of carbon chain, a multiplet at 1.6 ppm related to β carbonyl methylene protons, and a signal at 5.36 ppm due to olefinic hydrogen. The percentage conversion of triglycerides to methyl esters using eqn (1) was found to be 93.48%.
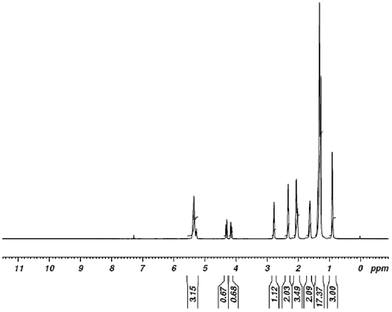 |
| Fig. 8 1H NMR spectrum of the waste frying oil. | |
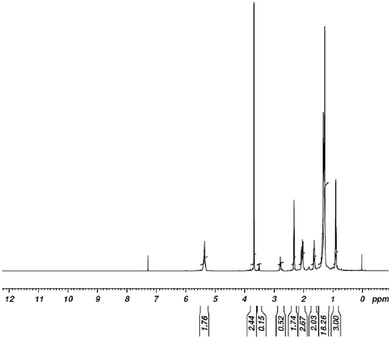 |
| Fig. 9 1H NMR spectrum of the methyl ester catalyzed by egg shell based CaO. | |
Reusability of the catalyst
Reusability of the egg shell based CaO for transesterification of waste frying oil in a continuous flow RD system was carried out for 5 cycles under the conditions of time at 7 h, methanol to oil ratio at 4
:
1, catalyst bed height at 150 mm and feed flow rate at 0.2 mL min−1 (Fig. 10). At the end of each cycle, the column was unloaded and the catalysts were completely rinsed with methanol to remove the adsorbed stains. Then it was recalcined at 900 °C for 2.5 h for further reuse. The same procedure was repeated for five times and the methyl ester conversion obtained after each time were 93.48, 92.47, 91.66, 93.33, and 93.22% respectively. This result clearly indicates that there was insignificant catalyst deactivation observed after five cycles of usage.
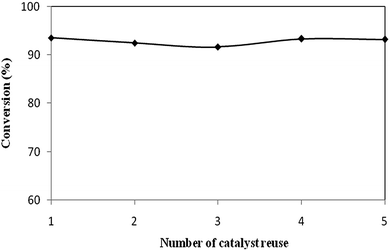 |
| Fig. 10 Effect of reusability of the egg shell based CaO catalyst (time = 7 h, flow rate = 0.2 mL min−1, height of the catalyst bed = 150 mm, methanol–oil ratio = 4 : 1). | |
Conclusions
A continuous flow jacketed reactive distillation process using heterogeneous catalyst was developed for transesterification of waste frying oil with methanol. Egg shell based CaO catalyst showed excellent activity for the production of biodiesel in the continuous flow RD system. In this system, the methanol to oil ratio was reduced to 4
:
1 compared to 6
:
1 in the conventional homogeneous processes and 9
:
1 in the batch processes studied earlier which provides an effective and economical approach to the existing continuous process for biodiesel production. Application of this system can be further investigated by using different heterogeneous catalysts for commercial feasibility.
Acknowledgements
The authors gratefully acknowledge the financial assistance from Council of Scientific and Industrial Research (CSIR), New Delhi, India in the form of Senior Research Fellowship to S. Niju.
Notes and references
- D. W. Lee, Y. M. Park and K. Y. Lee, Catal. Surv. Asia, 2009, 13, 63–77 CrossRef CAS.
- Z. Helwani, M. R. Othman, N. Aziz, J. Kim and W. J. N. Fernando, Appl. Catal., A, 2009, 363, 1 CrossRef CAS PubMed.
- A. Kawashima, K. Matsubara and K. Honda, Bioresour. Technol., 2009, 100, 696–700 CrossRef CAS PubMed.
- Y. Feng, A. Zhang, J. Li and B. He, Bioresour. Technol., 2011, 102, 3607–3609 CrossRef CAS PubMed.
- Z. Wei, C. Xu and B. Li, Bioresour. Technol., 2009, 100, 2883–2885 CrossRef CAS PubMed.
- S. Niju, K. M. Meera, S. Begum and N. Anantharaman, Environ. Prog. Sustainable Energy, 2014 DOI:10.1002/ep.11939.
- N. Girish, S. Niju, K. M. Meera, S. Begum and N. Anantharaman, Fuel, 2013, 111, 653–658 CrossRef CAS PubMed.
- W. Suryaputra, I. Winata, N. Indraswati and S. Ismadji, Renewable Energy, 2013, 50, 795–799 CrossRef CAS PubMed.
- P. L. Boey, G. P. Maniam, S. A. Hamid and D. M. H. Ali, Fuel, 2011, 90, 2353–2358 CrossRef CAS PubMed.
- J. Boro, A. J. Thakur and D. Deka, Fuel Process. Technol., 2011, 92, 2061–2067 CrossRef CAS PubMed.
- Y. Ren, B. He, F. Yan, H. Wang, Y. Cheng, L. Lin, Y. Feng and J. Li, Bioresour. Technol., 2012, 113, 19–22 CrossRef CAS PubMed.
- K. Prasertsit, C. Mueanmas and C. Tongurai, Chem. Eng. Process., 2013, 70, 21–26 CrossRef CAS PubMed.
- A. A. Kiss, A. C. Dimian and G. Rothenberg, Energy Fuels, 2008, 22, 598–604 CrossRef CAS.
- G. Knothe, J. Am. Oil Chem. Soc., 2006, 83, 823–833 CrossRef CAS.
- S. F. A. Halim, A. H. Kamaruddin and W. J. N. Fernando, Bioresour. Technol., 2009, 100, 710–716 CrossRef CAS PubMed.
- O. Tepe and A. Y. Dursun, J. Hazard. Mater., 2008, 151, 9–16 CrossRef CAS PubMed.
- A. Buasri, B. Ksapabutr, M. Panapoy and N. Chaiyut, Korean J. Chem. Eng., 2012, 29, 1708–1712 CrossRef CAS PubMed.
- X. Liu, X. Piao, Y. Wang, S. Zhu and H. He, Fuel, 2008, 87, 1076–1082 CrossRef CAS PubMed.
- M. Kouzu, T. Kasuno, M. Tajika, Y. Sugimoto, S. Yamanaka and J. Hidaka, Fuel, 2008, 87, 2798–2806 CrossRef CAS PubMed.
|
This journal is © The Royal Society of Chemistry 2014 |