DOI:
10.1039/C4RA05088F
(Paper)
RSC Adv., 2014,
4, 35422-35427
An ultrasensitive fluorometric platform for S1 nuclease assay based on cytochrome c†
Received
29th May 2014
, Accepted 1st August 2014
First published on 4th August 2014
Abstract
An ultrasensitive and straightforward fluorescent sensing platform for S1 nuclease activity has been developed based on S1 nuclease-induced DNA strand scission and the difference in affinity of cytochrome c (Cyt c) for single-stranded DNA (ssDNA) containing different numbers of bases. This sensing platform consists of a negatively charged fluorophore carboxyfluorescein (FAM) labeled 20-mer ssDNA (20F) and a positively charged protein Cyt c. In the absence of S1 nuclease, the electrostatic and hydrophobic interaction between 20F and Cyt c makes the FAM be in close proximity to Cyt c, resulting in efficient quenching of the fluorescence of FAM. Conversely, and very importantly, in the presence of S1 nuclease, it cleaves 20F into small fragments. The introduction of Cyt c into the sensing solution results in weak quenching of the fluorescence of FAM due to the relatively weak electrostatic interaction between the fragmented ssDNA chains and Cyt c, making the FAM move away from Cyt c, and thus the fluorescence intensity gradually increases with increasing concentration of S1 nuclease. S1 nuclease can be detected in a range of 4.0 × 10−3 to 4.0 × 10−2 units per mL with a detection limit of 8.3 × 10−4 units per mL and good selectivity. Moreover, the sensing system is used for the detection of an inhibitor of S1 nuclease with satisfying results.
Introduction
Nucleases are a family of enzymes that can cleave the phosphodiester bonds between the nucleotide subunits of nucleic acids.1,2 These cleavage reactions play key roles in a variety of fields ranging from biotechnology to pharmacology, as well as in biological processes, such as DAN replication, repair, recombination, PCR assay, molecular cloning, genotyping, and mapping.3–8 As a result, the assay of nucleases is of high importance for the development of efficient therapeutics and drug discovery.9–11 Traditional techniques, including gel electrophoresis, high-performance liquid chromatography (HPLC), enzyme-linked immunosorbent assay (ELISA), and radioactive labeling have been established to monitor nuclease activity.12–15 Most of them are time-consuming, laborious, and require sophisticated instrumentation or isotope labelling.16–21 To address these limitations, a number of detection methods have been developed in recent years, including fluorescent,22–24 chemiluminescent,25 electrochemical,26 and colorimetric methods.27 Among all these methods fluorometric detection has become an ever-growing and attractive means due to its high sensitivity, rapid response, and simple manipulation.
Recently, cytochrome c (Cyt c) which containing 104 amino acid residues and a metal-containing heme cofactor has attracted increasing interest in making DNA-based optical sensors.28 The isoelectric point (pI) of Cyt c is 10.0–10.5.29 It can interact with single-stranded DNA (ssDNA) by electrostatic and hydrophobic interactions in slightly basic solution.30,31 Furthermore, Cyt c was reported to be a fluorescence quencher due to its heme moiety,32–34 which, in combination with the unique Cyt c/DNA interactions, has been employed to develop sensing systems for the detection of proteins and protease. Liao and coworkers firstly developed a fluorescence turn-on method for real-time monitoring of protease activity using Cyt c as both a quencher and a nature substrate.30 Besides Cyt c, a fluorophore labeled single stranded DNA (FAM-ssDNA) was employed as the signal reporter. When there is a protease, Cyt c will be digested into small peptide fragments thus the FAM-ssDNA was released from Cyt c, which resulted in the recovery of the FAM fluorescence. Subsequently, they designed a new approach for the sensitive and selective sensing of proteins using aptamer as recognition element.31 These fluorescence assays based on the electron transfer between a fluorophore labeled ssDNA and Cyt c have shown great advantages.
Inspired by these previous study, herein, we report a simple and ultrasensitive fluorescence method for nuclease activity assay based on Cyt c. S1 nuclease, which exhibits endo- and exolytic hydrolytic activity for the phosphodiester bonds of ssDNA or RNA and produces mono- or oligonucleotide fragments,35,36 is taken as the model nuclease to provide the “proof-of-principle” verification of this method. The FAM-labeled 20-mer ssDNA (5′-FAM-TATATGGATGATGTGGTATT-3′) (20F) is used as the nuclease substrate. In the absence of S1 nuclease, the adsorption of the 20F on Cyt c by electrostatic and hydrophobic interactions makes the dyes close proximity to Cyt c surface, resulting in high efficiency quenching of fluorescence of the FAM. Conversely, in the presence of S1 nuclease, the 20F is hydrolyzed into small fragments. The introduction of Cyt c into the sensing solution results in weak quenching of the fluorescence of the FAM due to the relatively weak electrostatic interaction between the fragmented ssDNA chains and Cyt c, and the fluorescence intensity gradually increases with increasing concentration of S1 nuclease. A new fluorescence assay for the sensing of S1 nuclease activity was therefore established.
Experimental section
Chemicals and materials
The FAM-labeled 20-mer ssDNA with a sequence of 5′-FAM-TATATGGATGATGTGGTATT-3′ (20F), FAM-labeled 10-mer ssDNA with a sequence of 5′-FAM-TATATGGATG-3′ (10F), and FAM-labeled 5-mer ssDNA with a sequence of 5′-FAM-TATAT-3′ (5F), were synthesized by Shanghai Sangon Biotechnology Co., Ltd. (Shanghai, China). Cytochrome c (Cyt c), S1 nuclease, exonuclease I (Exo I), micrococcal nuclease (MNase), deoxyribonuclease I (DNase I) and exonuclease III (Exo III) were purchased from Shanghai Sangon Biotechnology Co., Ltd. (Shanghai, China). Bovine serum albumin (BSA) was purchased from Biosharp Co., Ltd. (Hefei, China). Lysozyme, and hemoglobin were purchased from Solarbio Co., Ltd. (Beijing, China). The buffer solutions used in this work are as follows: S1 nuclease buffer consisted of 40 mM CH3COONa–CH3COOH (pH 4.5), 300 mM NaCl, and 2 mM ZnCl2, and the Tris–HCl buffer consisted of 5 mM Tris–HCl (pH 7.8). Milli-Q purified water was used to prepare all the solutions.
Apparatus
Fluorescent emission spectra were performed on Varian cary eclipse fluorescence spectrophotometer, Varian Medical Systems, Inc. (Palo Alto, American). The sample cell is a 700 μL quartz cuvette. The luminescence intensity was monitored by exciting the sample at 480 nm and measuring the emission at 520 nm. The slits for excitation and emission were set at 5 nm, 10 nm respectively. The fitting of the experimental data was accomplished using the software Origin 8.0.
Optimization of the concentration of Tris–HCl buffer
To optimize the concentration of Tris–HCl, 2 μL of 20F stock solution (10 μM) was diluted with CH3COONa–CH3COOH buffer (pH 4.5) to 20 μL, and 80 μL Cyt c solution (1 μM) as prepared (by diluting the Cyt c stock solution to a concentration of 1 μM using 2 mM, 5 mM, 10 mM, 20 mM, 50 mM and 100 mM Tris–HCl buffer, respectively) were mixed. The mixed solution were diluted with 2 mM, 5 mM, 10 mM, 20 mM, 50 mM and 100 mM Tris–HCl buffer to 500 μL, respectively. The above prepared solution was incubated 10 min at room temperature. Finally, the fluorescence intensity of the incubated solution was measured at 520 nm with excitation at 480 nm.
Optimization of the pH value of Tris–HCl buffer
To optimize the pH value of Tris–HCl buffer, 2 μL of 20F stock solution (10 μM) was diluted with CH3COONa–CH3COOH buffer (pH 4.5) to 20 μL, and 80 μL Cyt c solution (1 μM) as prepared (by diluting the Cyt c stock solution to a concentration of 1 μM using pH 7.4, pH 7.6, pH 7.8 and pH 8.0 Tris–HCl buffer, respectively) were mixed. The mixed solution were diluted with pH 7.4, pH 7.6, pH 7.8 and pH 8.0 Tris–HCl buffer to 500 μL, respectively. The above prepared solution was incubated 10 min at room temperature. Finally, the fluorescence intensity of the incubated solution was measured at 520 nm with excitation at 480 nm.
Optimization of the concentration of cytochrome c (Cyt c)
To optimize the concentration of Cyt c, 2 μL of 20F stock solution (10 μM) was diluted with CH3COONa–CH3COOH buffer (pH 4.5) to 20 μL, and 0, 20, 40, 60, 80, and 100 μL Cyt c solution (1 μM) as prepared were mixed. The mixed solution was diluted with Tris–HCl buffer to 500 μL. The above prepared solution was incubated 10 min at room temperature. Finally, the fluorescence intensity of the incubated solution was measured at 520 nm with excitation at 480 nm.
Optimization of the reaction time between FAM-labeled 20-mer ssDNA (20F) and cytochrome c (Cyt c)
To optimize the reaction time between 20F and Cyt c assays, 2 μL of the 20F stock solution (10 μM) was diluted with CH3COONa–CH3COOH buffer (pH 4.5) to 20 μL, and 80 μL Cyt c solution (1 μM) as prepared were mixed. The mixed solution was diluted with Tris–HCl buffer to 500 μL. The above prepared solution was incubated for 0, 1, 2, 5, 10, 15, 20, 25, and 30 min at room temperature. Finally, the fluorescence intensity of the incubated solution was measured at 520 nm with excitation at 480 nm.
Optimization of the reaction time between FAM-labeled 20-mer ssDNA (20F) and S1 nuclease
To optimize the reaction time between 20F and S1 nuclease assays, 2 μL of the 20F stock solution (10 μM), and 3.2 × 10−2 units per mL S1 nuclease solution were mixed. The above prepared solution was incubated for 0, 5, 10, 15, 20, 25, 30, 35, 40, 45, 50, 55, and 60 min at 37 °C. Then 80 μL Cyt c solution (1 μM) as prepared was added to the solution, the mixed solution was diluted with Tris–HCl buffer to 500 μL. The above prepared solution was incubated for 10 min at room temperature. Finally, the fluorescence intensity of the incubated solution was measured at 520 nm with excitation at 480 nm.
Performance of S1 nuclease detection
For S1 nuclease assays, 2 μL of the 20F stock solution (10 μM), and appropriate concentrations of S1 nuclease solution were mixed, the mixed solution was diluted with CH3COONa–CH3COOH buffer (pH 4.5) to 20 μL. The above prepared solution was incubated for 30 min at 37 °C. Then 80 μL Cyt c solution (1 μM) as prepared was added to the solution, the mixed solution was diluted with Tris–HCl buffer to 500 μL. The above prepared solution was incubated for 10 min at room temperature. Finally, the fluorescence intensity of the incubated solution was measured at 520 nm with excitation at 480 nm.
Performance of inhibition assay
For ATP assays, 2 μL of the 20F stock solution (10 μM), 2 μL of the S1 nuclease solution (10 units per mL) and appropriate concentrations of ATP solution were mixed, the mixed solution was diluted with CH3COONa–CH3COOH buffer (pH 4.5) to 20 μL. The above prepared solution was incubated for 30 min at 37 °C. Then 80 μL Cyt c solution (1 μM) as prepared was added to the solution, the mixed solution was diluted with Tris–HCl buffer to 500 μL. The above prepared solution was incubated for 10 min at room temperature. Finally, the fluorescence intensity of the incubated solution was measured at 520 nm with excitation at 480 nm.
Results and discussion
Design strategy
Fig. 1 illustrates the sensing strategy for the detection of S1 nuclease. In the absence of S1 nuclease, FAM-labeled 20-mer ssDNA (20F) which is used as the nuclease substrate is polyanion. Cytochrome c (Cyt c) is positively charged when the assay solution pH is lower than its pI value. 20F could interact with the positively charged Cyt c via electrostatic and hydrophobic interactions. Then the fluorescence of 20F was effectively quenched through electron transfer from the FAM fluorophore to the metal-containing heme in Cyt c. The system shows very low background. However, upon the addition of S1 nuclease, the 20F is cut into fragments by S1 nuclease, the introduction of Cyt c into the sensing solution results in weak quenching of the fluorescence of the FAM due to the weak binding affinity of the short FAM-linked oligonucleotide fragment to Cyt c, and the fluorescence intensity gradually increases with increasing concentration of S1 nuclease. Therefore, the fluorescence intensity of FAM as a function of S1 nuclease concentration is measured correspondingly.
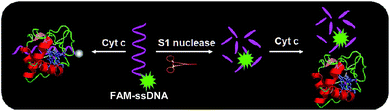 |
| Fig. 1 Scheme for the mechanism of Cyt c-based biosensor for S1 nuclease detection. | |
In order to demonstrate the feasibility of our strategy, we first investigated the fluorescence quenching efficiency of Cyt c to ssDNA containing different numbers of bases in length. As shown in Fig. S1,† the fluorescence quenching efficiency of Cyt c to ssDNA increased with the increasing number of bases in length, where F0 is the fluorescence intensity of these FAM-ssDNA in the absence of Cyt c; F is the fluorescence intensity of these FAM-ssDNA in the presence of Cyt c. When the concentration of Cyt c was fixed at 0.16 μM and oligonucleotide fixed at 40 nM, we observed that the fluorescence quenching efficiency of Cyt c to 20F was 89%. In contrast, for the similar ssDNA containing 5 bases (named 5F), a 41% fluorescence quenching efficiency was achieved. Thus, we chose 20F as the nuclease substrate. And this phenomenon indicated that the affinity of the short ssDNA to Cyt c is significantly weaker than that of the long ssDNA, which forms the basis for the design of Cyt c-based fluorescence biosensor for S1 nuclease detection.
Control experiments were performed with the fixed concentrations of 20F, and in the presence of three proteins of different sizes and pI values. They were bovine serum albumin (BSA), lysozyme, and hemoglobin (Table S1†). As shown in Fig. S2,† no obvious fluorescence quenching was observed except Cyt c, where F0 is the fluorescence intensity of the 20F in the absence of these proteins; F is the fluorescence intensity of the 20F in the presence of Cyt c, BSA, lysozyme, and hemoglobin, respectively. These results strongly support the notion that both the binding and the electron transfer between 20F and Cyt c caused the fluorescence quenching.
The process of fluorescence changing of the Cyt c-based biosensor for S1 nuclease detection is shown by fluorescence spectra. Fig. S3† shows 20F under different conditions. The fluorescence spectrum of 20F in Tris–HCl buffer shows strong fluorescence intensity that can be attributed to the presence of the FAM (Fig. S3,† curve a). In the presence of Cyt c, up to 89% quenching of the fluorescence emission was observed (Fig. S3,† curve b) because of the electron transfer between 20F and Cyt c. Upon the reaction of 20F with 3.2 × 10−2 units per mL S1 nuclease, the introduction of Cyt c into the sensing solution exhibits significant fluorescence intensity (Fig. S3,† curve c). However, in the absence of Zn2+, which is required for the maximal activity of S1 nuclease, no obvious fluorescence increasing was observed (Fig. S3,† curve d) comparing with the blank control (Fig. S3,† curve b). Therefore, the fluorescent response of the system mentioned above really comes from the degradation of 20F caused by S1 nuclease and S1 nuclease detection could be easily realized by monitoring the change of fluorescence signal.
Optimization of detection strategy
To achieve the best sensing performance, a series of experiments were performed to optimize the conditions with acceptable signal response. The effect of pH value, the concentration of buffer, the concentration of Cyt c, the quenching reaction time between Cyt c and 20F, and S1 nuclease-catalyzed digestion reaction time were investigated.
The concentration and the pH value of the assay buffer always play an important role in chemical reaction, especially in biochemical reaction, thus the influence of the concentration and the pH value of the assay buffer on the fluorescence quenching were investigated. Fig. S4† shows fluorescence quenching efficiency of Cyt c to 20F in different concentration of Tris–HCl buffer. When the concentration of Tris–HCl buffer is 5 mM, the fluorescence quenching efficiency of Cyt c to 20F reaches the highest value. As a result, 5 mM was used as the optimized concentration of Tris–HCl buffer. Fig. S5† shows the influence of pH value of the assay buffer on the fluorescence quenching efficiency of Cyt c to 20F. The fluorescence quenching of 20F by Cyt c was studied in 5 mM Tris–HCl buffer with pH range of 7.4–8.0. This pH range is selected to ensure Cyt c is positively charged (pI 10.0–10.5). As shown in Fig. S5,† no obvious changed in the fluorescence quenching efficiency of Cyt c to 20F. This was a gratifying phenomenon that revealed the sensor had good anti-pH impact ability. In our experiment, a pH value of 7.8 was selected as the assay buffer pH value unless otherwise mentioned.
After optimizing the assay buffer condition, the concentration of Cyt c was optimized. As shown in Fig. 2, with the increasing concentration of Cyt c, the fluorescence intensity of 20F decreased and trended to a minimum value at 0.16 μM. Thus, 0.16 μM was taken as the optimized concentration for Cyt c.
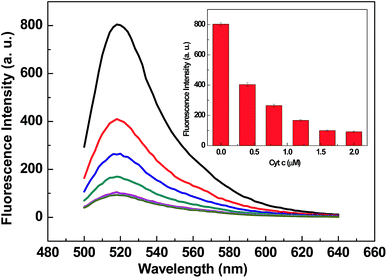 |
| Fig. 2 Fluorescence emission spectra of 20F upon the addition of different concentrations of Cyt c. Inset: fluorescence intensity of 20F versus concentration of Cyt c. Concentration: 20F, 40 nM; Cyt c, 0, 0.04, 0.08, 0.12, 0.16, 0.2 μM. Excitation: 480 nm. | |
The kinetic behaviors of 20F and Cyt c, as well as the 20F with S1 nuclease, were studied by monitoring the fluorescence intensity as a function of time. Fig. S6A† shows the fluorescence quenching of 20F in the presence of Cyt c as a function of incubation time. The interaction process of 20F to Cyt c is very fast at room temperature. It reaches equilibrium in 5 min. Fig. S6B† shows fluorescence intensity versus different reaction time of 20F (40 nM) with S1 nuclease (3.2 × 10−2 units per mL). It indicates that the reaction of 20F with S1 nuclease becomes slower and is completed in about 30 min at 37 °C. So we introduced Cyt c into the sensing solution after the system had reacted for 30 min at 37 °C.
S1 nuclease detection
The assay of S1 nuclease was carried out under the optimized conditions with the fixed concentrations of 20F (40 nM) and Cyt c (0.16 μM). Fig. 3A shows the fluorescence emission spectra of the Cyt c-based biosensor in the presence of different concentrations of S1 nuclease. The fluorescence intensity of the biosensor dramatically increases with the increasing concentration of S1 nuclease. The calibration curve for S1 nuclease detection is shown in Fig. 3B, and the linear range is from 4.0 × 10−3 to 4.0 × 10−2 units per mL with linear equation y = 15125x + 97.7, where y is the fluorescence intensity of FAM at 520 nm and x is the concentration of S1 nuclease (determination coefficient R2 = 0.9983). The detection limit is estimated to be 8.3 × 10−4 units per mL (3S0/S, in which S0 is the standard deviation for the blank solution, n = 11, and S is the slope of the calibration curve), which is of much lower than those reported S1 nuclease biosensors.21,22,24,37–39 A series of eleven repetitive measurements of 3.2 × 10−2 units per mL S1 nuclease were used for estimating the precision, and the relative standard deviation (RSD) was 2.7%, showing good reproducibility of the proposed method.
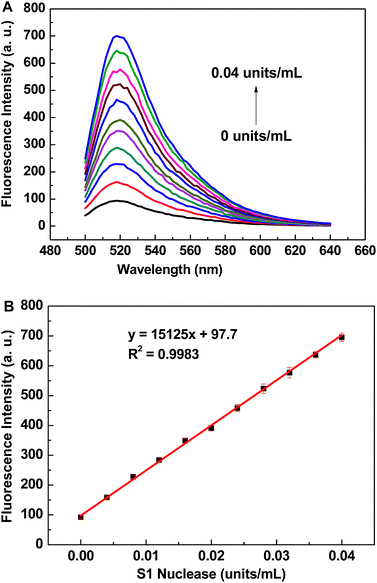 |
| Fig. 3 Fluorescence emission spectra of Cyt c-based biosensor in the presence of increasing amount of S1 nuclease and calibration curve for S1 nuclease detection. (A) Fluorescence emission spectra of Cyt c-based biosensor in the presence of increasing amount of S1 nuclease. (B) Calibration curve for S1 nuclease detection. Concentration: 20F, 40 nM; Cyt c, 0.16 μM. Excitation: 480 nm. | |
Specificity
In this study, under the optimized detection condition, other nucleases (Exo I, MNase, DNase I and Exo III) were selected to study the specificity of Cyt c-based biosensor under the same conditions. Exo I breaks apart single-stranded DNA in the direction 3′ → 5′, releasing deoxyribonucleoside 5′-monophosphates one after another. Exo I has its maximal activity at the basic pH (9.5) and requires Mg2+ for maximal activity. MNase is an extracellular nuclease, which could preferentially digest single-stranded DNA at AT-rich regions at slightly basic pH (8.0) and require Ca2+ for maximal activity. DNase I could digest both ssDNA and double-stranded DNA (dsDNA) at slightly basic pH (8.0). Exo III is a 3′ → 5′ exonuclease specific for dsDNA or DNA–RNA hybrids. In a typical experiment, 20F was incubated with 0.032 units per mL Exo I, 0.032 units per mL MNase, 0.032 units per mL DNase I, 0.032 units per mL Exo III and 0.032 units per mL Exo I 30 min at 37 °C in CH3COONa–CH3COOH buffer (pH 4.5), respectively. Then introduce Cyt c into these sensing solution, respectively. As shown in Fig. 4, the fluorescence intensity of this Cyt c-based biosensor changed less for Exo I, MNase, DNase I and Exo III, while a significant fluorescence increase was observed for S1 nuclease. The observation indicates the specificity of the Cyt c-based biosensor for S1 nuclease testing.
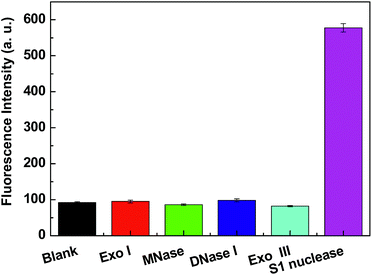 |
| Fig. 4 Fluorescence intensity of Cyt c-based biosensor in the presence of different nucleases: blank control (without S1 nuclease); Exo I (0.032 units per mL); MNase (0.032 units per mL); DNase I (0.032 units per mL) Exo III (0.032 units per mL) and S1 nuclease ((0.032 units per mL)). Concentration: 20F, 40 nM; Cyt c, 0.16 μM. Excitation: 480 nm. | |
Inhibition assay
The cleavage reaction of DNA with nuclease can be prohibited when the nuclease inhibitor is present. ATP has been reported to be an effective inhibitor to prevent the cleavage of single strands by S1 nuclease.40 Fig. 5 shows fluorescence intensity changes of the sensing system in the presence of 0.04 units per mL S1 nuclease and different amounts of ATP. With the increase of inhibitor amount, the fluorescence intensity of the biosensor dramatically decreases. The digestion of 20F by S1 nuclease is effectively inhibited by ATP at 0.32 mM. The control experiments indicated that ATP itself could not result in the fluorescence intensity change (shown in ESI Fig. S7†). These results further demonstrate that Cyt c-based biosensor can be used not only for nuclease activity assay but also for the detection of nuclease inhibitors.
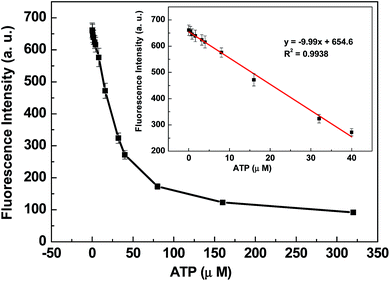 |
| Fig. 5 Fluorescence intensity of the 20F-S1 nuclease–Cyt c system versus concentration of ATP. Inset: calibration curve for ATP detection. Concentration: 20F, 40 nM; S1 nuclease, 0.04 units per mL; Cyt c, 0.16 μM. Excitation: 480 nm. | |
Conclusions
In summary, an ultrasensitive and straightforward strategy for S1 nuclease detection based on cytochrome c (Cyt c) is developed in this work. The FAM labeled single-stranded DNA (20F) was connected to Cyt c for energy transfer via an electrostatic interaction. Upon the addition of S1 nuclease, the FAM labeled ssDNA was cleaved into small fragments. The introduction of Cyt c into the sensing solution results in weak quenching of the fluorescence of the dyes due to the different electrostatic interaction strength of ssDNA and fragments with Cyt c caused different changes of quenching efficiency, and increasing of the FAM fluorescence was observed. The emission intensity changes could be directly related to the amount of S1 nuclease added to the assay solution. This Cyt c-based biosensor is extraordinarily sensitive to S1 nuclease detection. As to S1 nuclease, a sensitive detection limit of 8.3 × 10−4 units per mL was obtained. Moreover, the sensing system is used for the detection of an inhibitor of S1 nuclease with satisfying results. We expect that this assay platform will become an important assay tool in drug screening and basic research related to S1 nuclease.
Acknowledgements
This work was supported by the Fundamental Research Funds for the Central Universities (no. SWU113099) and the National Key Technology R&D Program (no. 2009BADB7B04) and China Agriculture Research System (no. CARS-27).
Notes and references
- O. T. Avery, C. M. MacLeod and M. McCarty, J. Exp. Med., 1944, 79, 137 CrossRef CAS.
- W. Arber, Angew. Chem., Int. Ed., 1978, 17, 73 CrossRef CAS PubMed.
- M. R. Lieber, BioEssays, 1997, 19, 233 CrossRef CAS PubMed.
- S. C. West, Nat. Rev. Mol. Cell Biol., 2003, 4, 435 CrossRef CAS PubMed.
- T. M. Marti and O. Fleck, Cell. Mol. Life Sci., 2004, 61, 336 CrossRef CAS PubMed.
- J. Hoorfar, P. Ahrens and P. Rådström, J. Clin. Microbiol., 2000, 38, 3429 CAS.
- N. D. Grindley, K. L. Whiteson and P. A. Rice, Annu. Rev. Biochem., 2006, 75, 567 CrossRef CAS PubMed.
- P. Norberg, T. Bergstrom and J. A. J. Liljeqvist, J. Clin. Microbiol., 2006, 44, 4511 CrossRef CAS PubMed.
- J. Cote and A. Ruiz-Carrillo, Science, 1993, 261, 765 CAS.
- W. Choi and R. M. Harshey, Proc. Natl. Acad. Sci. U. S. A., 2010, 107, 10014 CrossRef CAS PubMed.
- P. Rouet, F. Smih and M. Jasin, Proc. Natl. Acad. Sci. U. S. A., 1994, 91, 6064 CrossRef CAS.
- H. J. Kwon and D. S. Kim, Int. J. Biochem. Cell Biol., 1998, 30, 217 CrossRef CAS.
- V. Bouriotis, A. Zafeiropoulos and Y. D. Clonis, Anal. Biochem., 1987, 160, 127 CrossRef CAS.
- A. Jeltsch, A. Fritz, J. Alves, H. Wolfes and A. Pingoud, Anal. Biochem., 1993, 213, 234 CrossRef CAS PubMed.
- J. Smith and E. V. Anslyn, Anal. Biochem., 1994, 220, 53 CrossRef CAS PubMed.
- L. J. Ou, P. Y. Jin, X. Chu, J. H. Jiang and R. Q. Yu, Anal. Chem., 2010, 82, 6015 CrossRef CAS PubMed.
- C. H. Leung, D. S. Chan, B. Y. Man, C. J. Wang, W. Lam, Y. C. Cheng, W. F. Fong, W. L. Hsiao and D. L. Ma, Anal. Chem., 2011, 83, 463 CrossRef CAS PubMed.
- G. Song, C. Chen, J. Ren and X. Qu, ACS Nano, 2009, 3, 1183 CrossRef CAS PubMed.
- Y. Huang, S. Zhao, M. Shi, J. Chen, Z. F. Chen and H. Liang, Anal. Chem., 2011, 83, 8913 CrossRef CAS PubMed.
- X. Xu, M. S. Han and C. A. Mirkin, Angew. Chem., Int. Ed., 2007, 46, 3468 CrossRef CAS PubMed.
- R. Cao, B. Li, Y. Zhang and Z. Zhang, Chem. Commun., 2011, 47, 12301 RSC.
- X. J. Yang, F. Pu, J. S. Ren and X. G. Qu, Chem. Commun., 2011, 47, 8133 RSC.
- J. Lee and D. H. Min, Analyst, 2012, 137, 2024 RSC.
- F. Pu, D. Hu, J. S. Ren, S. Wang and X. G. Qu, Langmuir, 2010, 26, 4540 CrossRef CAS PubMed.
- W. Li, Z. L. Liu, H. Lin, Z. Nie, J. H. Chen, X. H. Xu and S. Z. Yao, Anal. Chem., 2010, 82, 1935 CrossRef CAS PubMed.
- E. Palecek, M. Fojta, M. Tomschik and J. Wang, Biosens. Bioelectron., 1998, 13, 621 CrossRef CAS.
- R. Cao, B. X. Li, Y. F. Zhang and Z. N. Zhang, Chem. Commun., 2011, 47, 12301 RSC.
- M. M. Bushey and J. W. Jorgenson, J. Microcolumn Sep., 1990, 2, 299 CrossRef PubMed.
- B. F. Van Gelder and E. C. Slater, Biochim. Biophys. Acta, 1962, 58, 595 Search PubMed.
- D. L. Liao, Y. X. Li, J. Chen and C. Yu, Anal. Chim. Acta, 2013, 784, 72 CrossRef CAS PubMed.
- D. L. Liao, J. Chen, W. Y. Li, Q. F. Zhang, F. Y. Wang, Y. X. Li and C. Yu, Chem. Commun., 2013, 49, 9458 RSC.
- C. H. Fan, K. W. Plaxco and A. J. J. Heeger, J. Am. Chem. Soc., 2002, 124, 5642 CrossRef CAS PubMed.
- M. Liu, P. Kaur, D. H. Waldeck, C. H. Xue and H. Y. Liu, Langmuir, 2005, 21, 1687 CrossRef CAS PubMed.
- Y. Y. Wang, Y. Zhang and B. Liu, Anal. Chem., 2010, 82, 8604 CrossRef CAS PubMed.
- F. Harada and J. E. Dahlberg, Nucleic Acids Res., 1975, 2, 865 CrossRef CAS PubMed.
- R. C. Wiegand, G. N. gocdson and C. M. Radding, J. Biol. Chem., 1975, 250, 8848 CAS.
- Z. X. Zhou, J. B. Zhu, L. B. Zhang, Y. Du, S. J. Dong and E. K. Wang, Anal. Chem., 2013, 85, 2431 CrossRef CAS PubMed.
- Y. Zhang, Y. Y. Wang and B. Liu, Anal. Chem., 2009, 81, 3731 CrossRef CAS PubMed.
- M. Liu, H. M. Zhao, S. Chen, H. T. Yu and X. Quan, ACS Nano, 2012, 6, 3142 CrossRef CAS PubMed.
- P. Wrede and A. Rich, Nucleic Acids Res., 1979, 7, 1457 CrossRef CAS PubMed.
Footnote |
† Electronic supplementary information (ESI) available. See DOI: 10.1039/c4ra05088f |
|
This journal is © The Royal Society of Chemistry 2014 |