DOI:
10.1039/C4RA04529G
(Paper)
RSC Adv., 2014,
4, 40312-40320
Fabrication and characterization of flexible silk fibroin films reinforced with graphene oxide for biomedical applications
Received
14th May 2014
, Accepted 13th August 2014
First published on 18th August 2014
Abstract
Nanocomposite films based on silk fibroin (SF) and graphene oxide (GO) were fabricated by simply casting the two components in aqueous media. The structure and properties of the films were systematically characterized by scanning electron microscope (SEM), X-ray diffraction (XRD), Fourier transform infrared spectroscopy (FT-IR), differential scanning calorimetry (DSC), thermal gravimetric analysis (TGA) and tensile tests. The investigations demonstrated that the presence of GO in the SF matrix increased the silk II content in the composite films by the intermolecular forces between GO and SF molecular chains. These newly formed interactions also contributed to the improvement of the film's mechanical properties and thermal stability. Further, the biodegradation behavior and biocompatibility were investigated to evaluate the biological performance of the SF/GO films. Compared with the SF film, the films prepared by incorporating GO showed a lower enzymatic degradation rate, reflecting their improved resistance to the applied enzyme solution. The cell viability and proliferation results in vitro indicated that the composite films, especially the SF/GO film with 0.5 wt% GO, could support cell adhesion and proliferation. This study has developed a novel, flexible and biocompatible SF/GO composite film by a green and feasible method, which should encourage the development of a series of SF/GO-based materials for biomedical applications.
1. Introduction
Silkworm silk from Bombxy mori has attracted considerable attention and has been used commercially as sutures for decades. Silk fiber is primarily composed of two protein components. Silk fibroin (SF) is the core structural protein of silk fibers and sericin is the water-soluble glue-like protein that binds the fibroin fibers together.1 During decades of research, SF has been popular for biomedical fields because of its remarkable mechanical properties, biocompatibility, minimal inflammatory reaction and long-term biodegradability.2–7 Additionally, silks offer versatility in processing so that the regenerated SF solution can be easily made into films,8 porous scaffolds,9 gels10 or non-woven mats11 by different fabrication techniques. With such excellent properties and diverse processability, silk proteins have been employed in tissue regeneration as substitutes for skin tissue,12 bone tissue13 and even blood vessels.14
Trying to design and synthesize novel materials instead of adopting traditional natural materials is always a challenge in the biomaterials preparation process. Newfound graphene oxide (GO) has triggered considerable interest in developing a variety of new types of composites owing to its huge potential in various fields including biomedicine.15–18 GO is essentially a monolayer of sp2-bonded carbon atoms with a high density of oxygen-containing functional groups including hydroxyls, epoxides and carbonyls bound on the surface and edges of each single-layered carbon atom sheet.19 Thanks to the abundant functional groups, GO can be uniformly dispersed in water or some organic solvents,20 which provides a convenient means to fabricate graphene-based biomaterials. Moreover, the unique 2D structure,21 high specific surface area, outstanding mechanical properties22 and low cytotoxicity23 are critical reasons for the utility of GO in biomedical fields including drug delivery,24 tumor targeted therapy,25 and biological imaging.26
Since GO-based materials exhibit huge potential in biomedical applications, and silk fibroin is an acknowledged candidate for biomaterials, we are very interested in investigating GO and SF together. Before our work, several researchers have attempted to prepare SF/GO-based materials. Huang et al. were inspired by the natural nacre and prepared strong composite films with layered structures by facile solution casting of SF/GO hydrogels.27 Hu and co-workers fabricated novel ultrathin SF/GO nanocomposite membranes with high tensile strength, modulus and toughness by the layer-by-layer technique.28 Although the development of the SF/GO blend materials has been exciting, our understanding of these nanocomposites remains unclear and limited. No previous reports systematically investigated the structure and properties of the SF/GO films and studied the applicability of the nanocomposite as a scaffold in biomedical fields.
Taking these considerations into account, we here report a novel SF/GO composite film with low content of GO prepared by a simple and green solution casting method. It should be noted that the usage of glycerol as a cross-linking agent of SF and the low concentration of GO in the SF matrix are both essential to avoid significant cytotoxic effects on cell growth. In contrast to the previous reports, we aim to gain a comprehensive understanding of the film structure and properties, to evaluate the influences of GO on the morphology, thermal stability and mechanical properties of the composites, to assess the biological performance of the blend films and ultimately to provide a novel SF/GO-based biomaterial.
2. Experimental section
2.1 Materials
Bombxy mori raw silk fibers were purchased from Dingsheng Silk Co. Ltd. (Wujiang, China). Graphene oxide was purchased from XFNano Materials Tech Co. Ltd. (Nanjing, China). Murine fibroblast cells L-929 were purchased from Edinburgh Biological Tech Co. Ltd. (Shanghai, China). Dulbecco's modification of Eagle's medium (DMEM) and fetal bovine serum (FBS) were purchased from Gibco Invitrogen. Glycerol (G-2025), protease-XIV and methyl thiazolyl tetrazolium (MTT) were purchased from Sigma-Aldrich Trading Co. Ltd. (Shanghai, China).
2.2 Preparation of the SF/GO composite films
A solution of SF was produced as previously described.6 Briefly, the Bombyx mori silk fibers were degummed 3 times in a 0.05 wt% Na2CO3 solution at 100 °C for 30 minutes, rinsed thoroughly with distilled water and dried at 60 °C overnight. 30 g of extracted silk fibers were dissolved in 300 ml ternary solvent of CaCl2
:
CH3CH2OH
:
H2O (1
:
2:8 molar ratio) at 72 °C for 1 hour with constant stirring. Then, the solution was dialyzed against distilled water for 4 days, yielding a ∼3.5 wt% SF solution. 100 mg GO powder was dispersed in 50 ml of distilled water by ultrasonication for 30 minutes, forming a stable GO dispersion (2 mg ml−1). Sodium hydroxide solution (1 mol L−1) was added into the GO dispersion to change its pH value to 10. This GO suspension was introduced into the diluted SF solution (2 wt%) dropwise, and then a homogeneous and transparent blend solution was obtained after stirring for 30 minutes followed by adding glycerol at a weight ratio of 20% (w/w). To avoid serious aggregation of GO and significant cytotoxic effect on cell growth, we adopted relatively low GO loading (within 1 wt%) and the final GO contents in the composite films were 0.1, 0.2, 0.5 and 1.0 wt% relative to the weight of SF, respectively. The mixture was degassed in vacuum, poured into a glass Petri dish and placed in an oven at 40 °C for 48 hours to form the SF/GO composite films. Finally, the films were rinsed with distilled water for 24 hours to remove the residual glycerol and dried at ambient temperature for further characterization.
2.3 Characterizations
2.3.1 Morphology and structure of the SF/GO composite films. The SF/GO composite films were sputter-coated with gold in vacuum and the surface and cross-section morphologies of the samples were observed with a field-emission scanning electron microscope (SEM, JEOL, Japan). X-ray diffraction (XRD) patterns of the SF/GO nanocomposites were recorded using a Miniflex II (Rigaku, Japan) diffractometer with Cu Kα radiation (λ = 1.54178 Å). All samples were cut into micro-particles before analysis and measured with 2θ ranging from 5° to 40°. The Fourier transform infrared (FT-IR) spectra (KBr pellet) of the samples were recorded on a Nicolet Magna-IR 750 spectrometer in the range of 4000–500 cm−1.
2.3.2 Thermal properties of the SF/GO composite films. The thermal properties of the SF/GO films were analyzed by differential scanning calorimetry (DSC) and thermal gravimetric analysis (TGA). DSC was measured by a NETZSCH DSC 200F3 instrument at a heating rate of 10 °C min−1 under a dry nitrogen gas flow of 50 ml min−1. TGA curves were obtained on a TA Q600 instrument. Samples were heated to 600 °C at a scanning rate of 10 °C min−1 under a nitrogen atmosphere.
2.3.3 Mechanical properties of the SF/GO composite films. The specimens for mechanical study were equilibrated in a chamber of RH 65% for 12 hours and then the tensile properties were performed on an electronics universal tensile testing machine (TRAPEZIUM, Japan) with a tensile speed of 5 mm min−1 at 20 °C. The size of the films was 8 cm in length and 1 cm in width and their thicknesses were measured using a micrometer before the test. For each sample, at least 5 measurements were carried out and the average values are reported.
2.3.4 Behavior of the SF/GO composite films subjected to enzyme. The composite films were incubated at 37 °C in 50 ml of PBS solution containing 1 U ml−1 protease-XIV for 7 days. Solutions were replenished with enzyme daily and groups of samples were rinsed in distilled water and prepared for mass balance, SEM and XRD tests at designated time points.
2.3.5 Cell adhesion and viability on the SF/GO composite films. Murine fibroblast L-929 cells were used to evaluate the cytotoxicity and biocompatibility of the SF/GO composite membranes. The different SF/GO films, each with a diameter of 1 cm, were placed in 24-well tissue culture plates (Corning Inc., USA), rinsed with distilled water for 7 days and sterilized by γ-ray irradiation. L929 cells at a density of 2.5 × 104 cells per ml were seeded onto the composite films in the 24-well plates. Then the cell-seeded films were incubated in DMEM with 10% FBS at 37 °C in a humidified atmosphere of 95% air and 5% CO2. The medium in each well was replaced every 2 days.The morphology of the L929 cells on the SF/GO films were observed by SEM. Samples were fixed with 2.5% glutaraldehyde at 4 °C overnight, followed by 3 washes in sterilized PBS, and dehydrated with gradient ethanol solutions. Specimens were then sputter-coated with a 20 nm-thick gold film in vacuum and examined using a JEOL JSM-7001F SEM.
The viability of the L929 cells were assessed by MTT tests on days 1, 3, 5, and 7 after seeding in vitro. Briefly, the cell-seeded films were incubated in an MTT solution (5 mg ml−1 in PBS) at 37 °C for 4 h, and then the blue formazan crystals produced by viable cells were dissolved in DMSO for 10 minutes. The optical density (OD) of formazan was measured on a Synergy HT (BIO-TEK) microplate reader at 490 nm (after subtracting the OD from blank wells only containing unseeded films). Six MTT assay replicates were performed for each sample and culture period.
2.3.6 Statistical analysis. Statistical comparisons were performed using SPSS 16.0 statistical software, and differences at P < 0.05 are considered statistically significant.
3. Results and discussion
3.1 Morphology
The surface and the cross-section morphologies of the SF/GO composite films were observed using SEM (Fig. 1). A similar feature was detected from the surface images of the SF film (Fig. 1a) and the SF/GO film (Fig. 1b): they all had a relatively smooth and homogenous morphology, which indicates that GO nanosheets were well dispersed in the SF matrix without aggregation. However, noticeable changes were shown in the cross-section morphology of the two kinds of films. An interesting nanofilament or nanoglobular morphology was formed in the SF film (Fig. 1c), while such a structure disappeared and the GO nanosheets could be clearly observed in the cross-section of the composite film (Fig. 1d). GO sheets were uniformly dispersed and embedded in the SF matrix, indicating the well-dispersed status without aggregation and the strong interactions between the two components in the composites. Obviously, GO and SF were compatible because of the abundant oxygen-containing groups on the GO sheets and various polar side-chain groups in the SF molecule.
 |
| Fig. 1 SEM images of the SF film, surface (a) and cross-section (c); and the SF/GO 1.0 wt% composite film, surface (b) and cross-section (d). | |
3.2 Structural characteristics
To confirm the influences of GO content on the secondary structure of the SF materials, the condensed structure of the SF/GO composite films was determined by XRD and FT-IR. As shown in Fig. 2f, the characteristic XRD peak of the neat GO appeared at 2θ = 10.6°, corresponding to a layer-to-layer distance (d-spacing) of 0.83 nm.27 For SF, previous studies demonstrated that the main diffraction peaks of the silk I structure are at 12.2°, 19.7°, 24.7° and 28.2°, while those of the silk II structure are at 9.1°, 18.9°, 20.7° and 24.3°.6 The typical peaks at 12.2°, 19.7° and 24.3° appeared in the XRD curve of the SF film (Fig. 2a), providing strong evidence for the presence of silk I structure with a small amount of silk II structure.
 |
| Fig. 2 XRD curves of the SF/GO films with different GO contents: (a) SF, (b) SF/GO 0.1 wt%, (c) SF/GO 0.2 wt%, (d) SF/GO 0.5 wt%, (e) SF/GO 1.0 wt%, (f) GO. | |
After GO was dispersed into the SF matrix, the XRD patterns of the SF/GO films (Fig. 2b–e) only showed the SF diffraction peaks and no obvious diffraction peak at 10.6° was found, demonstrating GO was well exfoliated and uniformly dispersed in the SF matrix.29,30 Compared with the pure SF film, the strength of the silk II peak at 24.3° increased, and it is particularly notable that the typical diffraction peak at 20.7° assigned to the silk II structure emerged gradually as the GO content increased to 0.5 and 1 wt% (Fig. 2d and e). These results provide convincing evidence of a link between the silk II crystal formation and the incorporation of GO nanosheets.
Further, FT-IR was employed to investigate the molecular conformation of the SF/GO composite films (Fig. 3). In the FT-IR spectrum of GO, strong absorption bands at 3389 cm−1, 1728 cm−1, 1630 cm−1 and 1045 cm−1 appeared, indicative of O–H stretching vibration, C
O stretching vibration of the carboxylic group, C
C stretching vibration assigned to the sp2 network of the unoxidized graphite domains, and C–O–C stretching vibration of the epoxy group respectively.31
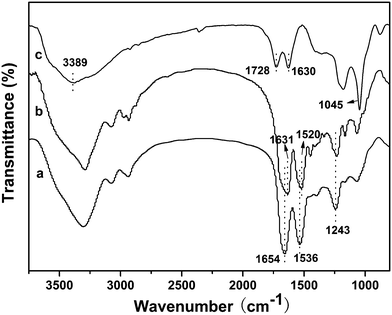 |
| Fig. 3 FT-IR spectra of the SF/GO films with different GO contents: (a) SF film, (b) SF/GO 1.0 wt% and (c) GO. | |
For SF, FT-IR studies have shown that absorption bands at 1650–1655 cm−1 (amide I), 1525–1540 cm−1 (amide II), 1266 cm−1 (amide III), and 669 cm−1 (amide V) are characteristics of the silk I secondary structure, the bands at 1620–1635 cm−1 (amide I), 1530 cm−1 (amide II), 1230–1235 cm−1 (amide III), and 700 cm−1 (amide V) are from the silk II structure, and those at 1655–1660 cm−1 (amide I), 1535–1545 cm−1 (amide II), 1235 cm−1 (amide III), and 650 cm−1 (amide V) are indicative of the random coil conformation.6,8 In the spectrum of the SF film, strong bands at 1654 cm−1, 1536 cm−1 and 1243 cm−1 were observed, indicating a typical silk I conformation. After GO was dispersed into the SF matrix, the amide I and amide II bands shifted to 1631 cm−1 and 1520 cm−1 respectively, implying the formation of the silk II structure under the influence of GO. The FT-IR results correspond with the XRD results, helping to explain the relationship between the SF molecular chains and the GO nanosheets.
In brief, by incorporating GO into the SF-based materials, formation of the silk II crystal structure in the SF/GO composite films was promoted. This transformation is likely caused by the formation of hydrogen bonds or other intermolecular forces between the two components. When the GO nanosheets were introduced and once the interactions were formed, the SF molecular chains should be in a relatively stretched state due to the hydrophilic or hydrophobic interactions, which would promote the rearrangements of the adjacent chain segments, and lead to new hydrogen bonds in new positions and consequently produce more silk II crystal structure.
3.3 Thermal properties
The thermal properties of the SF/GO composite films were estimated by DSC and TGA. Fig. 4a shows the DSC curves for the SF/GO composite films. All the samples produced an endothermic peak at around 83 °C, which is due to the evaporation of the bound water in the films. Two degradation peaks were found in the region 270–283 °C for both the SF film and the SF/GO composite films, indicating the coexistence of the silk I and silk II structures.8 Interestingly, with the incorporation of GO, the strength of the degradation peak assigned to the silk I structure at around 270 °C decreased, while the strength of the silk II structure degradation peak at around 283 °C increased, implying the decrease in the amount of the silk I crystal structure and the increase in the amount of the silk II structure.7 The DSC results are consistent with the XRD and FT-IR results, confirming that the presence of GO in the SF matrix promoted the formation of the silk II crystal structure by the intermolecular forces between GO and SF molecular chains.
 |
| Fig. 4 DSC and TGA curves of the SF/GO films with different GO contents. (A) DSC curves. The samples are as follows: (a) SF, (b) SF/GO 0.1 wt%, (c) SF/GO 0.2 wt%, (d) SF/GO 0.5 wt%, (e) SF/GO 1.0 wt%. (B) TGA curves. Inset: magnification of the water loss stage during the degradation process. | |
TGA curves of the SF/GO composite films are shown in Fig. 4b. All the samples showed a mass loss of about 8% before 100 °C due to the evaporation of the bound water in the films (Fig. 4b inset). The rapid weight loss from about 260 °C represents the thermal degradation of the silk fibroin. After GO was added, both the SF film and SF/GO nanocomposites yielded similar TGA curves; the composite films underwent a rapid weight loss in the same temperature region, and no obvious decomposition step corresponding to GO was observed as a result of the low loading of GO in the films. However, some differences between the curves of the pure SF film and the composite films were still obvious. The temperature at which the weight loss was 3% increased from 56.82 to 68.46 °C with the increase of GO content. Additionally, the total weight loss of the SF film was obviously greater than that of the composite films at 600 °C, which was 63.99% for the neat SF and 60.11% for the SF/GO 1.0 wt% film. As expected, GO could stabilize the composite films at high temperature and this was ascribed to the strong interactions between the SF molecules and the GO nanosheets. Moreover, the increased content of silk II structure provided synergistic effects to improve the thermal properties of the SF/GO-based films.
3.4 Mechanical properties
Appropriate mechanical properties are necessary for biomaterials to support the regeneration of functional tissues. However, the single-component SF-based materials always show lower flexibility and mechanical toughness without additional reagent, which contributes to their stiff and brittle behavior in a dry state. These features significantly hinder their use in a wide range of applications. A previous study demonstrated that glycerol could improve the toughness of the SF films by increasing the ultimate elongation, whereas the tensile strength at break was compromised gradually.32 Thus, we have integrated GO into the SF matrix in the current study to compensate for the loss of strength, and ultimately fabricated an SF/GO-based biomaterial with high strength as well as impressive flexibility.
The mechanical behaviors of the films are shown in Fig. 5. The obvious yield point in the strength–strain curves of all the samples provides strong evidence for the toughness of the composite films. The improvement of the flexibility of the film was ascribed to the usage of glycerol because it functioned as a plasticizer to preserve high water content.32 Compared with the pure SF film, the mechanical performance of the composite films was significantly improved by the incorporation of GO sheets. Specifically, the tensile strength and Young's modulus of the SF/GO nanocomposites increased from 20.64 to 35.49 MPa and from 381.26 to 844.75 MPa respectively as the GO loading increased from 0 to 1.0 wt%. The enhancement of the tensile strength and Young's modulus was related to the uniform dispersion of GO nanosheets and the intermolecular forces formed between the SF and GO nanosheets, which could have increased the load transfer efficiency and thus reinforced the composite films effectively. However, the breaking elongation of the nanocomposites gradually decreased from 78.92% to 34.36% with the increase of GO content. This phenomenon could be ascribed to the interactions between the SF molecules and GO nanosheets, which restricted the free slippage or the movement of the SF chains and ultimately led to a lower elongation. Additionally, it could be due to the preferential formation of the crystalline silk II when GO was used, which could be stiffer/stronger but more brittle than the silk I structure.
 |
| Fig. 5 Tensile stress–strain curves of the SF/GO composite films with different GO contents. | |
3.5 Enzymatic degradation behavior of the SF/GO composite films
Degradability is critically important for biomaterials used in tissue engineering. In the current study, the enzymatic degradation behavior of the SF/GO composite films was explored systematically. Fig. 6 shows the morphological changes of the composite films during the degradation process. As expected, the morphology of the pure SF film and the SF/GO composite film exhibited visible differences after being cultivated in the protease-XIV solution at 37 °C for a period of time. The neat SF film showed a relatively rapid degradation and many cracks were formed on the film surface. From the magnified image (Fig. 6b), the nanofilaments were observed to be oriented and arranged distinctly in the internal parts of the crack, which is consistent with the nanostructure of the SF film shown in Fig. 1c. Compared with the SF film, the composite film with 1.0 wt% GO loading degraded slowly and the internal GO nanosheets were exposed gradually with increased cultivation time. The surface morphology of the composite film showed a lamellar structure. Parts of the GO nanosheets still seemed to be coated with an SF matrix (Fig. 6c), while other parts of the GO presented a clear crumpled structure and similarly anchored to the film tightly (Fig. 6d and e). These results indicate that GO had strong interfacial adhesion with the SF molecules. Additionally, the dispersion state of GO seemed to be almost parallel to the surface of the composite film, which was caused by the unique 2D structure and the gravitational forces of the GO sheets.
 |
| Fig. 6 SEM images of the different films cultivated in protease XIV solution: (a and b) SF film; (c–e) SF/GO 1.0 wt% film degraded for 7 days (the white arrows represent the GO nanosheets coated with SF matrix, the black arrows represent the fully exposed GO nanosheets). | |
Weight loss is a visual indicator to measure the degradation rate for biodegradable materials. Fig. 7 shows the mass of the SF/GO composite films during a degradation period of 7 days by protease-XIV. All the samples clearly degraded in the enzymatic solution as evidenced by the vigorous weight loss. However, the SF/GO films exhibited a slower degradation rate than did the SF film. Specifically, the SF film rapidly degraded, and the mass remaining was only 7.02% of the initial mass after 7 days. After GO incorporation, the composite films degraded gradually with time and the degradation rate was decreased with an increase in the concentration of GO. With a GO loading of 1.0 wt%, the mass was reduced to 26.59% of the initial mass, yielding a significantly higher remaining mass than that of the pure SF film. The incorporation of GO probably played an important role in determining the extent of degradation of the various films. The multiblock nature of silk backbones with alternating hydrophilic and hydrophobic nanoscale domains facilitated a combination of hydrogen bonding, polar–polar, and hydrophobic interactions with the amphipathic GO nanosheets, which could reduce the diffusion of the enzymatic solution into the composite films and consequently lead to a lower degradation rate. Furthermore, the increased silk II structure in the composite films also made considerable contributions to the improved resistance to the enzymatic solutions.
 |
| Fig. 7 Quantitative changes of the SF/GO composite films during the enzymatic degradation. | |
To reveal the biodegradation behavior of the SF/GO composite films further, the structural changes during the degradation process were also investigated. As shown in Fig. 8, the structures of both the SF film and SF/GO 0.5 wt% blend film were primarily silk I before degradation. After treatment with protease-XIV, the typical diffraction peaks at 9.1°, 20.7° and 24.3° emerged while the strength of the silk I peak at 12.2° decreased with time, indicating the amount of silk II structure increased gradually. These findings show that the amorphous region and unstable crystal structure (silk I) first degraded in protease-XIV solution, resulting in the increase of stable crystal structure (silk II) content in the degraded films. Moreover, compared with the pure SF film, the silk II peak at 20.7° of the SF/GO composite film appeared earlier in the degradation process, which might imply the higher silk II content in the original composite films before degradation. Such results are consistent with our structural investigations described above.
 |
| Fig. 8 XRD curves of the degraded SF film (a) and SF/GO 0.5 wt% film (b) with degradation time. | |
3.6 Cell adhesion and viability on the SF/GO composite film
As a naturally occurring fibrous protein, the impressive biocompatibility and nontoxicity of silk fibroin make the SF-based materials widely used in biomedical fields such as tissue engineering, controlled release systems of drugs or growth factors and even optical apparatuses.33–35 With regards to GO, many researchers have investigated its biocompatibility and the exciting result is that low concentrations of GO have proven to be safe in vitro and even in vivo.22,36 However, the interaction between cells and materials as well as the surface morphology may lead to some yet unknown impacts on the biocompatibility of the composites.37 Thus, it still needs to be confirmed whether the incorporation of GO will lead to some negative effects on the single-component SF-based materials. In the current study, murine fibroblast L-929 cells were selected to be cultured on the films for 7 days to assess the cytotoxicity and biocompatibility of the nanocomposites.
The SEM observation of the L-929 cells cultured on the SF/GO composite films showed a relatively high density and normal morphology at day 7 (Fig. 9). Cells adhered and anchored onto the films tightly and they were coated with a large amount of extracellular matrix. Further, obvious pseudopods were observed using SEM, indicating a good growth state and viability of the cells.
 |
| Fig. 9 SEM images of L-929 cells cultured on the SF/GO composite films at day 7: (A, a) SF, (B, b) SF/GO 0.1 wt%, (C, c) SF/GO 0.2 wt%, (D, d) SF/GO 0.5 wt%, (E, e) SF/GO 1.0 wt%. | |
An MTT test was performed to reveal the cell viability and proliferation on different films (Fig. 10). An obvious increase of cell proliferation was observed during the 7 day culture in standard conditions, which demonstrated that all samples were nontoxic and that L929 cells were able to adhere and proliferate normally. However, the trend of the cell growth speed was distinct for different culture times and the cell viability differed from film to film. The number of L929 cells on all the films increased relatively slowly from day 1 to day 5, while by day 7 the number of viable L929 fibroblasts increased sharply as evidenced by the increase in the OD490 value from ∼0.3 to ∼0.6. For SF/GO 0.5 wt% composite film, there was a statistically significant (P < 0.05) increase in the number of viable cells at day 1, 5 and 7 compared with other SF/GO composite films. Although the majority of the SF/GO films exhibited a lower cell proliferation rate than did the pure SF film, the composite film with 0.5 wt% GO loading still showed a similar OD490 value to that of the SF membrane at day 3 and day 5, and even exceeded that of the SF membrane at day 1 and day 7. The composite film showed better biocompatibility with a GO content of 0.5 wt%. These results demonstrate the excellent cytocompatibilty of the SF/GO composite films and that these films could support cellular attachment, proliferation and differentiation.
 |
| Fig. 10 MTT assays of the cell adhesion and viability on the SF/GO composite films. | |
4. Conclusions
Flexible and biocompatible SF/GO composite films were prepared by a simple and environmentally friendly method without any nocuous reagent. A higher content of silk II crystal structure in the composite films was produced as a result of the intermolecular forces between the functional groups of GO and the polar groups on the SF molecular chains. The thermal stability and mechanical properties of the composite films increased at fairly low concentrations of GO, and the incorporation of GO also improved the resistance of these films to degradation from an enzyme solution. The data of in vitro cell culture experiments demonstrated that the SF/GO composite films could support cell survival and proliferation and the SF/GO 0.5 wt% film exhibited optimal biocompatibility. The newly developed SF/GO-based nanocomposite with excellent properties and good biocompatibility is a competitive candidate in biomedical engineering.
Acknowledgements
We gratefully acknowledge the financial support from Shanxi Scholarship Council of China (no. 2012-049).
References
- D. L. Kaplan, W. Adams, B. Farmer and C. Viney, ACS Symposium Series, Washington, DC, 1994, vol. 544, pp. 2–16 Search PubMed.
- G. H. Altman, F. Diaz, C. Jakuba, T. Calabro, R. L. Horan, J. S. Chen, H. L. Lu, J. Richmond and D. L. Kaplan, Biomaterials, 2003, 24, 401–416 CrossRef CAS.
- C. Vepari and D. L. Kaplan, Prog. Polym. Sci., 2007, 32, 991–997 CrossRef CAS PubMed.
- C. Y. Jiang, X. Y. Wang, R. Gunawidjaja, Y. H. Lin, M. K. Gupta, D. L. Kaplan, R. R. Naik and V. V. Tsukruk, Adv. Funct. Mater., 2007, 17, 2229–2237 CrossRef CAS PubMed.
- L. Meinel, S. Hofmann, V. Karageorgiou, C. Kirker-Head, J. McCool, G. Gronowicz, L. Zichner, R. Langer and D. L. Kaplan, Biomaterials, 2005, 26, 147–155 CrossRef CAS PubMed.
- M. Z. Li, M. Ogiso and N. Minoura, Biomaterials, 2003, 24, 357–365 CrossRef CAS.
- Q. Lu, B. Zhang, M. Z. Li, B. Q. Zuo, D. L. Kaplan, Y. L. Huang and H. S. Zhu, Biomacromolecules, 2011, 12, 1080–1086 CrossRef CAS PubMed.
- Q. Lu, X. Hu, X. Q. Wang, J. A. Kluge, S. Z. Lu, P. Cebe and D. L. Kaplan, Acta. Biomater., 2010, 6, 1380–1387 CrossRef CAS PubMed.
- R. Nazarov, H. J. Jin and D. L. Kaplan, Biomacromolecules, 2004, 5, 718–726 CrossRef CAS PubMed.
- A. M. Hopkins, L. D. Laporte, F. Tortelli, E. Spedden, C. Staii, T. J. Atherton, J. A. Hubbell and D. L. Kaplan, Adv. Funct. Mater., 2013, 23, 5140–5149 CrossRef CAS PubMed.
- A. Schneider, X. Y. Wang, D. L. Kaplan, J. A. Garlick and C. Egles, Acta. Biomater., 2009, 5, 2570–2578 CrossRef CAS PubMed.
- S. Q. Yan, Q. Zhang, J. N. Wang, Y. Liu, S. Z. Lu, M. Z. Li and D. L. Kaplan, Acta. Biomater., 2013, 9, 6771–6782 CrossRef CAS PubMed.
- S. Miyamoto, R. Koyanagi, Y. Nakazawa, A. Nagano, Y. Abiko, M. Inada, C. Miyaura and T. Asakura, J. Biosci. Bioeng., 2013, 115, 575–578 CrossRef CAS PubMed.
- M. Lovett, C. Cannizzaro, L. Daheron, B. Messmer, G. Vunjak-Novakovic and D. L. Kaplan, Biomaterials, 2007, 28, 5271–5279 CrossRef CAS PubMed.
- M. Ionita, M. A. Pandele and H. Iovu, Carbohydr. Polym., 2013, 94, 339–344 CrossRef CAS PubMed.
- S. Praneetha and A. V. Murugan, RSC Adv., 2013, 3, 25403–25409 RSC.
- H. A. Becerril, J. Mao, Z. F. Liu, R. M. Stoltenberg, Z. N. Bao and Y. S. Chen, ACS Nano, 2008, 2, 463–470 CrossRef CAS PubMed.
- D. Bitounis, H. A. Boucetta, B. H. Hong, D. H. Min and K. Kostarelos, Adv. Mater., 2013, 25, 2258–2268 CrossRef CAS PubMed.
- A. K. Geim and K. S. Novoselov, Nat. Mater., 2007, 6, 183–191 CrossRef CAS PubMed.
- J.
I. Paredes, S. Villar-Rodil, A. Martinez-Alonso and J. M. D. Tascon, Langmuir, 2008, 24, 10560–10564 CrossRef CAS PubMed.
- M. I. Katsnelson, Mater. Today, 2007, 10, 20–27 CrossRef CAS.
- H. Q. Chen, M. B. Muller, K. J. Gilmore, G. G. Wallace and D. Li, Adv. Mater., 2008, 20, 3557–3561 CrossRef CAS PubMed.
- X. Y. Zhang, J. L. Yin, C. Peng, W. Q. Hu, Z. Y. Zhu, W. X. Li, C. H. Fan and Q. Huang, carbon, 2011, 49, 986–995 CrossRef CAS PubMed.
- Z. Liu, J. T. Robinson, X. M. Sun and H. J. Dai, J. Am. Chem. Soc., 2008, 130, 10876–10877 CrossRef CAS PubMed.
- L. M. Zhang, J. G. Xia, Q. H. Zhao, L. W. Liu and Z. J. Zhang, Small, 2010, 4, 537–544 CrossRef PubMed.
- X. M. Sun, Z. Liu, K. Welsher, J. T. Robinson, A. Goodwin, S. Zaric and H. J. Dai, Nano Res., 2008, 1, 203–212 CrossRef CAS PubMed.
- L. Huang, C. Li, W. J. Yuan and G. Q. Shi, Nanoscale, 2013, 5, 3780–3786 RSC.
- K. S. Hu, M. K. Gupta, D. D. Kulkarni and V. V. Tsukruk, Adv. Mater., 2013, 25, 2301–2307 CrossRef CAS PubMed.
- X. S. Du, M. Xiao, Y. Z. Meng and A. S. Hay, Carbon, 2005, 43, 195–197 CrossRef CAS PubMed.
- X. S. Du, Z. Z. Yu, A. Dasari, J. Ma, M. S. Mo, Y. Z. Meng and Y. W. Mai, Chem. Mater., 2008, 20, 2066–2068 CrossRef CAS.
- H. L. Guo, X. F. Wang, Q. Y. Qian, F. B. Wang and X. H. Xia, ACS Nano, 2009, 3, 2653–2659 CrossRef CAS PubMed.
- S. Z. Lu, X. Q. Wang, Q. Lu, X. H. Zhang, J. A. Kluge, N. Uppal, F. Omenetto and D. L. Kaplan, Biomacromolecules, 2010, 11, 143–150 CrossRef CAS PubMed.
- A. M. Hopkins, L. D. Laporte, F. Tortelli, E. Spedden, C. Staii, T. J. Atherton, J. A. Hubbell and D. L. Kaplan, Adv. Funct. Mater., 2013, 23, 5140–5149 CrossRef CAS PubMed.
- F. P. Seib and D. L. Kaplan, Biomaterials, 2012, 33, 8442–8450 CrossRef CAS PubMed.
- D. G. Harkin, K. A. George, P. W. Madden, I. R. Schwab, D. W. Hutmacher and T. V. Chirila, Biomaterials, 2011, 32, 2445–2458 CrossRef CAS PubMed.
- K. Yang, H. Gong, X. Z. Shi and Z. Liu, Biomaterials, 2013, 34, 2787–2795 CrossRef CAS PubMed.
- R. C. You, X. F. Li, Y. Liu, G. Y. Liu, S. Z. Lu and M. Z. Li, J. Biomed. Mater. Res., Part A, 2014 DOI:10.1002/jbm.a.35097.
|
This journal is © The Royal Society of Chemistry 2014 |