DOI:
10.1039/C4RA04332D
(Paper)
RSC Adv., 2014,
4, 36006-36011
Single step synthesis of pluronic stabilized IR responsive gold nanoplates
Received
9th May 2014
, Accepted 4th August 2014
First published on 4th August 2014
Abstract
A single-step seed-mediated synthesis is employed to produce biocompatible infrared (IR) responsive gold nanoplates (Au-NPs) by using a biocompatible tri-block copolymer, pluronic F-127. Pluronic F-127 acts as a reducing, stabilizing and shape directing agent. Its hydrophobic polypropylene oxide (PPO) unit, preferentially binds to the {111} planes of FCC Au nuclei, inhibiting the growth on {111} crystallographic planes and promotes anisotropic growth along the {110} facets. Well faceted hexagonal, triangular and truncated triangular plates are obtained whose size ranges from 46 nm to 108 nm. These gold nanoplates exhibit strong surface plasmon resonance corresponding to in-plane quadruple vibrations which are tunable from 810 nm to 900 nm in the near-infrared (NIR) region. Aging effect of gold nanoplates are also monitored in terms of their plasmonic properties over a period of several weeks as synthesized nanoplates when preserved in pluronic F-127 demonstrate impressive stability over a period of several weeks. Tunable absorption of biocompatible gold nanoplates in the NIR region opens up new possibilities in the field of cancer diagnostics and therapeutics. The aging effect on the plasmon bands of Au-NPs is also monitored for several weeks when preserved in pluronic solvent.
Introduction
The synthesis of metal nanostructures having distinctive size and shape dependent tunable optical properties has attracted a great deal of technological interest ranging from photonic devices, biosensors, and surface enhanced Raman spectroscopy (SERS) to photothermal therapy.1–3 The plasmonic properties of metal nanoparticles are highly sensitive to their size, shape and morphology. Metal nanoparticles exhibit distinct optical properties due to the collective oscillations of conduction band electrons, known as surface plasmon resonance (SPR).4 Spherical nanoparticles exhibit single surface plasmon resonance, while the non-spherical nanoparticles of metal show more than one plasmon bands, which are sensitive to their dimensions.5 Experimental results and simulations have revealed that surface plasmon resonance of anisotropic gold nanoparticles have strong and tunable absorption from visible to near infrared (NIR) region.6,7 This tunable resonant absorption opened up new possibilities in the field of cancer diagnostics and therapy.8 Amongst various anisotropic nano structures of Au (nanorods, nanoshells, nanocages, etc.), gold nanoplates (Au-NPs) display strong absorption in the NIR region due to their in-plane and out-of-plane quadruple resonances.9,10 The size and shape sensitive in-plane dipole resonance of Au-NPs can be tuned from visible to NIR region.
Medical applications of Au nanoparticles necessitate them to be IR responsive and dispersible in water without any decay in their physical and chemical properties. Moreover for biomedical applications, dispersibility and stability of NPs against aggregation is also very important.11 In recent years, various synthesis methods have been established by researchers for the anisotropic growth of the nanoparticles of desired morphologies. Anisotropic Au nanostructures such as nanorods, nanowires, nanoplates, nanobelts and nanocrystals of different geometries (cubes, tetrahedra, icosahedra, octahedra, bipyramids, etc.) were obtained in high yield in recent years via different strategies like photosynthesis,12 thermosynthesis,13 wet-chemical route,14 biochemical synthesis,15 etc. These methods involve complex experimentations, lengthy synthesis protocols and various toxic chemicals.16–18 In addition, for biomedical applications a second step is required in which toxic surfactants are replaced with biocompatible polymers via ligand exchange reactions.19–21 Use of non-toxic chemicals, environmentally benign solvents and renewable materials are the emerging issues in the solution phase synthesis of biocompatible and IR responsive metal nanoparticles.22 In literature a limited work has been found on the synthesis of anisotropic Au nanostructures with tunable optical properties via green chemistry.19,23 Sakai et al. have reported a single step synthesis of Au-NPs in aqueous solution containing Poly(ethylene oxide)-poly(propylene oxide)-poly(ethylene oxide) (PEO-PPO-PEO) block copolymers.23
In the present work we report the single step synthesis of IR responsive Au-NPs by a seed-mediated synthesis using biocompatible tri-block copolymer, pluronic F-127 which acts as a reducing agent, growth director and stabilizer.24–26 Pluronic F-127 possesses no toxicity and excellent biocompatibility.27 It is approved by FDA (food and drug administration of USA) for in vivo biomedical applications.28 It binds to the surface of metal nanoparticles as a very thin film by hydrophobic interactions through polypropylene oxide block of the copolymer and controls the growth of Au-NPs along specific crystallographic directions.29,30 The molecular structure of pluronic is represented in the Fig. 1. The present synthesis protocols yields optically tunable plasmonic nanostructures whose in-plane quadruple resonance band can be tuned into the NIR region (800–1100 nm). Pluronic stabilized aqueous Au-NPs are also stable against aggregation.
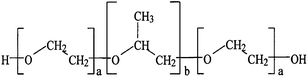 |
| Fig. 1 Chemical structure of Pluronic (poly(ethylene oxide)-poly(propylene oxide) poly(ethylene oxide)). For Pluronic F-127, a = 100 and b = 66. | |
Experimental
Materials
Pluronic F-127 (Molecular weight: 12
600), Gold(III) chloride (HAuCl4, 99.99%), silver nitrate (AgNO3, 99.8%) and sodium borohydride (NaBH4) were purchased from Sigma-Aldrich, USA. Ascorbic acid (AA) was procured from Merck, India. All chemicals were used as-received without any purification. MilliQ ultrapure water (ρ = 18.2 MΩ) was used in all the experiments.
Synthesis of Au-NPs
Au-NPs are generally been synthesized by seed-mediated method.31 In the conventional seed-mediated synthesis of anisotropic gold nanostructures, cationic surfactant cetyltrimethylammonium bromide (CTAB) is used as capping agent which allows growth of nanostructures along specific crystallographic axis only.32 These nanostructures of gold could have potential applications in medicine due to tunable plasmonic properties, which is mainly restrained by the toxicity of CTAB.33 Normally to overcome this difficulty, a two-step synthesis method is followed. In the first step anisotropic Au-NPs are formed with CTAB followed by their ligand exchange with bio-compatible polymers like polyols and triblock co-polymers.21 Hunt is on for the direct synthesis of biocompatible anisotropic gold nanostructures which can be used for in vivo medical applications.
In the present article we report single step synthesis of biocompatible anisotropic gold nanostructures by using pluronic F-127 as capping and shape controlling agent. In a typical experiment, gold seeds are prepared by reduction of an aqueous 0.5 mM, 5 mL aqueous HAuCl4, by 10 mM, 0.6 mL ice-cold NaBH4.in the presence of 1 mM, 5 mL pluronic F-127. The color of the solution immediately changes from golden to brownish yellow (Fig. 2(A)). This seed solution is used within 2 hour for the synthesis of Au-NPs. An aqueous solution containing 1 mM, 5 mL pluronic F-127 and 1 mM, 5 mL HAuCl4 is prepared separately. To this growth solution 4 mM, 0.2 mL AgNO3 is added. Presence of silver ions acts as the nucleation centers for the growth of Au-NPs.34 100 mM, 45 μL ascorbic acid (AA) is added which immediately reduces Au ions and renders the solution colorless. 12 μL seed solution is added and is mixed gently. The color of the growth solution changes to light pink immediately on addition of seed, which further changes to dark-purple within 15–20 minutes (Fig. 2(B)). No further change in color of the colloid is observed. Throughout the experiment the reaction temperature is maintained at 25 °C ± 1 °C.
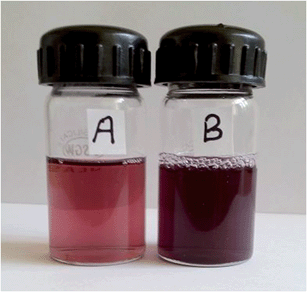 |
| Fig. 2 (A) Photographic view of Au seeds and (B) colloidal Au-NPs. Dark purple color of the solution indicates the formation of anisotropic Au-NPs. | |
Characterizations of Au-NPs
Optical response of as-synthesized Au-NPs in the visible and IR region is studied by Vis-NIR absorption spectroscopy. The measurements are carried out on the shimadzu UV-2600 double beam UV-Visible spectrophotometer in the spectrum range from 400–1200 nm. Morphological evolution of nanostructures is performed by transmission electron microscopy (TEM). TEM of the Au-NPs is obtained on Philips CM200 transmission electron microscope. Samples for transmission electron microscopy are prepared by placing a drop of aqueous colloidal Au-NPs on a carbon coated copper grid. The water is allowed to be evaporated slowly. Hydrodynamic size and size distribution is obtained by photon correlation spectroscopy. Measurements are carried out at 25 °C on the Brookhaven 90Plus particle size analyzer.
Results and discussion
Fig. 3 (solid line) shows the UV-Visible spectra of Au seeds. A shoulder centered at 510 nm is observed, which is a typical character of small Au seeds. The Vis-NIR spectrum of Au-NPs is also shown in Fig. 3 (dashed line). Two distinct plasmon resonance bands are observed indicating the growth of anisotropic nanostructures of Au.35 The first plasmon band at 526 nm corresponds to out-of-plane and second plasmon band at 810 nm corresponds to in-plane oscillations of electrons in Au-NPs.36 The hydrodynamic size of Au seeds is determined from the size distribution histogram by fitting it with the log normal particle size distribution function (Fig. 4(A)). Anisotropic characteristics of as-synthesized Au-NPs are further confirmed by their photon-correlation spectroscopy (Fig. 4(B)). Two distinct size distributions are observed which are corresponding to the different diffusion coefficients of Au-NPs along their long and short axis. The mean hydrodynamic size of NPs along their short axis is 15.1 nm and along the long axis is 73.9 nm. These bimodal hydrodynamic size distributions of Au-NPs further support our claim in Vis-NIR spectroscopy that as-synthesized Au nanoparticles have plate like asymmetric morphology.
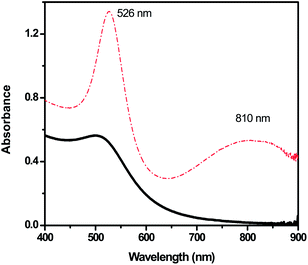 |
| Fig. 3 Vis-NIR spectra of gold seeds ( ) and Au-NPs ( ). Two distinct plasmon bands centered at 526 nm and 810 nm corresponds to the out-of-plane and in-plane oscillations indicating the formation of anisotropic Au-NPs. | |
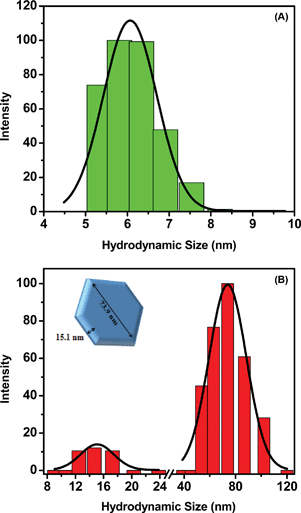 |
| Fig. 4 Hydrodynamic particle size distribution fitted with log normal particle size distribution. (A) Au-seeds and (B) Au-NPs. The mean hydrodynamic size of Au seeds is 6.2 nm and their polydispersity is 0.3. The mean hydrodynamic size of Au-NPs along the short axis (out-of-plane) is 15.1 nm and 73.9 nm along the long axis (in-plane) of Au-NPs. | |
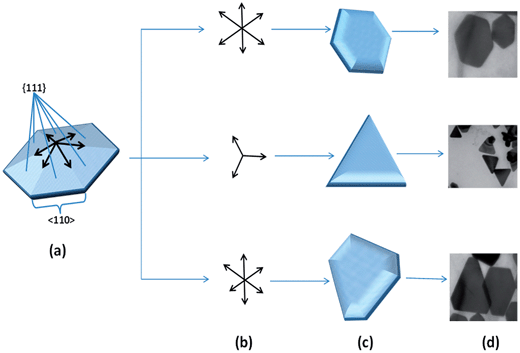 |
| Fig. 5 Schematic illustration of proposed growth mechanism of Au-NPs: (a) crystallographic facets with basal {111} plane and 〈110〉 side facet, (b) the formation of hexagonal plate, triangular plate and truncated triangular plate like shapes along the different 〈110〉 directions, (c) the expected particles shapes, (d) representative TEM images of hexagonal NPs, triangular NPs, and truncated triangular NPs. | |
Growth kinetics of Au-NPs
The growth mechanism of anisotropic gold nanostructures, specifically Au-NPs is of great concern as very limited information is available about this aspect.35 The growth of Au-NPs by using pluronics as shape directing agents has yet to be established. Generally the role of performed ‘seed’ and the capping agents are discussed in the various gold nanoparticles synthesis. Many groups37–41 have shown that by using growing seeds in solutions containing surfactant micelles, nanoparticles with well-defined shapes could be obtained. The growth rates of nanoparticles are often controlled kinetically rather than thermodynamically by different sticking probabilities by atoms on a particular crystallographic face.42 Being of lowest surface energy, the (111) face of the FCC metal has the lowest sticking probability as compared to other faces (γ(110) > γ(100) > γ(111)).43 Thus FCC metal has the highest energy to nucleate and grow into nanoparticles with their surfaces bounded by (111) facets in wet chemical synthesis.44 At a specific pluronic F-127/HAuCl4 molar ratio, the hydrophobic polypropylene oxide (PPO) preferentially adsorbed on {111} planes of FCC Au nuclei, inhibiting the growth on {111} crystallographic planes, and promotes anisotropic growth along the {110} facets.32,45–47 Under ideal conditions, the nuclei grow uniformly along six 〈110〉 directions if the growth environment is uniform, resulting in the formation of hexagonal plate surrounded by three pairs of {110} lateral faces (Fig. 5). However, due to local fluctuations in the growth medium, the crystal growth deviate from uniform growth along 〈110〉 directions. Therefore, triangular and truncated triangular (Fig. 5(c)) plates will be formed if the nuclei are grown along different 〈110〉 directions at different rates. TEM images of hexagonal nanoplates, triangular nanoplates and truncated triangular nanoplates are also shown in the Fig. 5.
To elucidate the growth mechanism of Au-NPs we monitored its growth kinetics using Vis-NIR spectroscopy. Within a minute of the addition of gold seeds into the growth solution, the formation of Au-NPs starts, which is evidenced from the two plasmon bands in the Vis-NIR spectra (Fig. 6). The plasmon band at 526 nm corresponds to the out-of-plane vibrations while the second band at 900 nm corresponds to in-plane quadruple oscillations of the electrons. As the time progresses, intensity of both the plasmon bands increases along with the red shift in the band position corresponding to in-plane quadruple oscillations indicating the growth of nanostructures along the {110} facets. The in-plane SPR stabilizes at 960 nm after 30 minutes. No further shift in the SPR band is observed indicating the saturation of the growth.
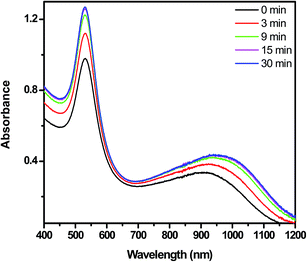 |
| Fig. 6 Growth kinetics of pluronic F-127 stabilized Au-NPs. Two plasmon bands (in-plane and out-of-plane) whose intensity increases as a function of time indicate the anisotropic growth of nanostructures. | |
Stability of Au-NPs
As-synthesized Au-NPs are separated from the growth medium by centrifugation @ 6000 rpm for 10 minutes. The supernatant is removed and the precipitates are washed multiple times with ultrapure water. Repeated centrifugations will remove free and excess pluronic along with the water soluble impurities (chloride and nitrate ions). Au-NPs are redispersed in 1 mM pluronic F-127 and stored at room temperature. The stability of Au-NPs is monitored by Vis-NIR spectroscopy. Fig. 7 shows Vis-NIR spectra of Au-NPs recorded after different time intervals of their storage. No considerable shift in the plasmon bands of pluronic stabilized Au-NPs are observed confirming the long term stability of pluronic stabilized Au-NPs. TEM image of pluronic stabilized Au-NPs is also shown in Fig. 8. Well faceted nanocrystals bounded by 〈111〉 planes are observed in the micrograph. The average physical size of NPs is 46 to 108 nm. The micrograph of NPs is either hexagonal, triangle or truncated triangle, vindicating the proposed growth mechanism in Fig. 5. An impressive stability of SPR peak at 810 nm is observed over first three weeks with remarkable increase in absorption from 0.3 to 1. From fourth week onwards, no further increase in the absorption maximum is observed.
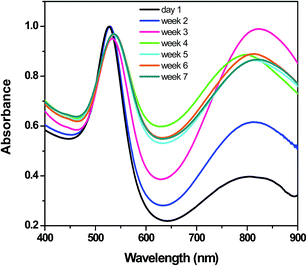 |
| Fig. 7 Investigation of aging of Au-NPs preserved in 1 mM pluronic F-127. The aging effect is monitored for 7 weeks from the synthesis. The plasmon band corresponding to in-plane quadruple resonance is stable at 810 nm upto 3 weeks. | |
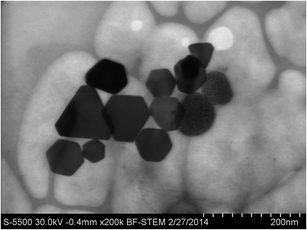 |
| Fig. 8 TEM micrograph of Au-NPs preserved in 1 mM pluronic F-127. Well faceted hexagonal plates, triangular plates and truncated triangles are observed whose size ranges from 46.5 nm to 108 nm. | |
Effect of ascorbic acid on Au-NPs
Ascorbic acid (AA) plays a vital role in the growth of asymmetric Au-NPs. In this work, a series of Au-NPs are prepared with different amount of (30 μL–60 μL) AA. The role of AA in the formation of Au-NPs is monitored by Vis-NIR spectroscopy (Fig. 9). SPR band corresponding to in-plane vibrations is obtained, when 30–35 μL of AA is used (curve a and b). As the amount of AA is increased to 40 μL the second SPR band appeared at 1000 nm (curve c). When the amount of AA is further increased, a consistent rise in absorption with a slight red shift is observed until the amount of AA reaches to 50 μL (curve c and d). When the amount of AA is increased beyond 50 μL, a significant blue shift is observed in the plasmon band corresponding to in-plane oscillations of the electrons. This blue shift is also accompanied by decrease in absorption maximum. The second plasmon resonance band disappears when AA is 60 μL indicating the formation of spherical nanoparticles. This study concludes that the amount of AA plays a vital role in the growth of anisotropic Au nanoparticles with a stable optical response in the NIR region.
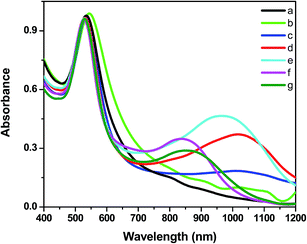 |
| Fig. 9 Effect of amount of AA on the spectral response of Au-NPs. Au-NPs are prepared with different amounts of AA, i.e. (a) 30 μL, (b) 35 μL, (c) 40 μL, (d) 45 μL, (e) 50 μL, (f) 55 μL and (g) 60 μL. With increasing AA amount blue shift of plasmon band corresponding to in-plane oscillations is observed which finally disappeared when AA is increase to 60 μL. With 60 μL AA, a single plasmon band is observed indicating the formation of spherical Au nanoparticles. | |
Conclusions
Single step synthesis of biocompatible pluronic F-127 stabilized Au nanoparticles in the form of hexagonal plates, triangles and truncated triangles is achieved by seed mediated synthesis. Growth mechanism that leads to formation of anisotropic nanoplates is also presented. NIR responsive Au-NPs are stable when preserved in pluronic F-127 and can be used for in vivo applications of cancer diagnosis and therapy.
Acknowledgements
Parveer Kaur is thankful to UGC, New Delhi for junior research fellowship under the MANF scheme for Sikh minority community. Authors also acknowledge financial support from DST (scheme no. SR/FTP/PS-109/2010) and CSIR (scheme no. 03(1226)/12/EMR-II).
References
- A. N. Shipway, E. Katz and I. Willner, ChemPhysChem, 2000, 1, 18–52 CrossRef CAS.
- M. C. Daniel and D. Astruc, Chem. Rev., 2004, 104, 293–346 CrossRef CAS PubMed.
- X. H. Huang, I. H. El-Sayed, W. Qian and M. A. El-Sayed, J. Am. Chem. Soc., 2006, 128, 2115–2120 CrossRef CAS PubMed.
- P. Patnaik, Appl. Biochem. Biotechnol., 2005, 126, 79–92 CrossRef.
- J. P. Wilcoxon and B. L. Abrams, Chem. Soc. Rev., 2006, 35, 1162–1194 RSC.
- S. Link, M. B. Mohamed and M. A. El-Sayed, J. Phys. Chem. B, 1999, 103, 3073–3077 CrossRef CAS.
- P. J. Jorge, P. S. Isabel, M. L. M. Luis and M. Paul, Coord. Chem. Rev., 2005, 249, 1870–1901 CrossRef PubMed.
- J. P. Justea, I. P. Santosa, L. M. L. Marzána and P. Mulvaney, Coord. Chem. Rev., 2005, 249, 1870–1901 CrossRef PubMed.
- L. J. Sherry, R. Jin, C. A. Mirkin, G. C. Schatz and R. P. V. Duyne, Nano Lett., 2006, 6, 2060–2065 CrossRef CAS PubMed.
- J. E. Millstone, S. J. Hurst, G. S. Métraux, J. I. Cutler and C. A. Mirkin, Small, 2009, 5, 646–664 CrossRef CAS PubMed.
- C. Burda, X. B. Chen, R. Narayanan and M. A. El-Sayed, Chem. Rev., 2005, 105, 1025–1102 CrossRef CAS PubMed.
- J. U. Kim, S. H. Cha, K. Shin, J. Y. Jho and J. C. Lee, Adv. Mater., 2004, 16, 459–464 CrossRef CAS PubMed.
- J. Zhang, Y. Gao, R. A. Alvarez-Puebla, J. M. Buriak and H. Fenniri, Adv. Mater., 2006, 18, 3233–3237 CrossRef CAS PubMed.
- N. Malikova, I. Pastoriza-Santos, M. Schierhorn, N. A. Kotov and L. M. Liz-Marzán, Langmuir, 2002, 18, 3694–3697 CrossRef CAS.
- S. S. Shankar, A. Rai, B. Ankamwar, A. Singh, A. Ahmad and M. Sastry, Nat. Mater., 2004, 3, 482–488 CrossRef CAS PubMed.
- N. Khlebtsov and L. Dykmana, Chem. Soc. Rev., 2011, 40, 1647–1671 RSC.
- E. C. Dreaden, A. M. Alkilany, X. Huang, C. J. Murphy and M. A. El-Sayed, Chem. Soc. Rev., 2012, 41, 2740–2779 RSC.
- N. Li, P. Zhao and D. Astruc, Angew. Chem., Int. Ed., 2014, 53, 1756–1789 CrossRef CAS PubMed.
- L. Vigderman, P. Manna and E. R. Zubarev, Angew. Chem., 2012, 124, 660–665 CrossRef PubMed.
- R. Sardar, J.-W. Park and J. S. S.- Parry, Langmuir, 2007, 23, 11883–11889 CrossRef CAS PubMed.
- Q. Dai, J. Coutts, J. H. Zou and Q. Huo, Chem. Commun., 2008, 2858–2860 RSC.
- P. Raveendran, J. Fu and S. L. J. Wallen, J. Am. Chem. Soc., 2003, 125, 13940–13941 CrossRef CAS PubMed.
- T. Sakai, Y. Horiuchi, P. Alexandridis, T. Okada and S. Mishima, J. Colloid Interface Sci., 2013, 394, 124–131 CrossRef CAS PubMed.
- Y. Lin and A. Paschalis, J. Phys. Chem. B, 2002, 42, 10834–10844 CrossRef.
- L. Yang and P. Alexandridis, Curr. Opin. Colloid Interface Sci., 2000, 5, 132–143 CrossRef CAS.
- R. Ivanova, B. Lindman and P. Alexandridis, J. Colloid Interface Sci., 2002, 252, 226–235 CrossRef CAS PubMed.
- E. Kim, J. Yang, J. Choi, J.-S. Suh, Y.-M. Huh and S. Haam, Nanotechnology, 2009, 20, 365602 CrossRef PubMed.
- P. Alexandridis and T. A. Hatton, Colloids Surf., A, 1995, 96, 1–46 CrossRef CAS.
- T. I. Abdullin, O. V. Bondar, Y. G. Shtyrlin, M. Kahraman and M. Culha, Langmuir, 2010, 26, 5153–5159 CrossRef CAS PubMed.
- G. Dumortier, G. L. Grossiord, F. Agnely and J. C. Chaumeil, Pharm. Res., 2006, 23, 2709–2728 CrossRef CAS PubMed.
- C. J. Murphy, T. K. Sau, A. M. Gole, C. O. Orendorff, J. Gao, L. Gao, S. E. Hunyadi and T. Li, J. Phys. Chem. B, 2005, 109, 13857–13870 CrossRef CAS PubMed.
- Z. Khan, S. Al-Thabaiti, A. Obaid, Z. Khan and A. Al-Youbi, J. Colloid Interface Sci., 2012, 367, 101–108 CrossRef CAS PubMed.
- C. J. Murphy, A. M. Gole, J. W. Stone, P. N. Sisco, A. M. Alkilany, E. C. Goldsmith and S. C. Baxter, Acc. Chem. Res., 2008, 41, 1721–1730 CrossRef CAS PubMed.
- X. Huang, S. Neretina and M. A. El-Sayed, Adv. Mater., 2009, 21, 4880–4910 CrossRef CAS PubMed.
- Y. Xia, Y. Xiong, B. Lim and S. E. Skrabalak, Angew. Chem., Int. Ed., 2009, 48, 60–103 CrossRef CAS PubMed.
- R. Jin, Y. W. Cao, C. A. Mirkin, K. L. Kelly, G. C. Schatz and J. G. Zheng, Science, 2001, 294, 1901–1903 CrossRef CAS PubMed.
- D. J. Smith, A. K. P.- Long, L. R. Wallenberg and J. O. Bovin, Science, 1986, 233, 872–875 CAS.
- N. R. Jana, L. Gearheart and C. J. Murphy, Adv. Mater., 2001, 13, 1389–1393 CrossRef CAS.
- T. K. Sau, A. Pal, N. R. Jana, Z. L. Wang and T. Pal, J. Nanopart. Res., 2001, 3, 257–261 CrossRef CAS.
- T. K. Sau and C. J. Murphy, J. Am. Chem. Soc., 2004, 126, 8648–8649 CrossRef CAS PubMed.
- X. Zou, E. Ying and S. Dong, Nanotechnology, 2006, 17, 4758–4764 CrossRef CAS PubMed.
- H. S. Nam, N. M. Hwang, B. D. Yu and J. K. Yoon, Phys. Rev. Lett., 2002, 89, 275502 CrossRef.
- J. M. Zhang, F. Ma and K. W. Xu, Appl. Surf. Sci., 2004, 229, 34–42 CrossRef CAS PubMed.
- B. Wiley, Y. Sun, J. Chen, H. Cang, Z.-Y. Li, X. Li and Y. Xia, MRS Bull., 2005, 30, 356–361 CrossRef CAS.
- H. Heinz, B. L. Farmer, R. S. Pandey, J. M. Slocik, S. S. Patnaik, R. Pachter and R. R. Naik, J. Am. Chem. Soc., 2009, 131, 9704–9714 CrossRef CAS PubMed.
- J. Feng, R. B. Pandey, R. J. Berry, B. L. farmer, R. R. Naik and H. Heinz, Soft Matter, 2011, 7, 2113–2120 RSC.
- C. Kan, X. Zhu and G. Wang, J. Phys. Chem. B, 2006, 110, 4651–4656 CrossRef CAS PubMed.
|
This journal is © The Royal Society of Chemistry 2014 |