DOI:
10.1039/C4RA03566F
(Paper)
RSC Adv., 2014,
4, 24810-24815
Controlled drug release from a novel drug carrier of calcium polyphosphate/chitosan/aldehyde alginate scaffolds containing chitosan microspheres
Received
19th April 2014
, Accepted 27th May 2014
First published on 28th May 2014
Abstract
Initial burst release of drugs is a major shortcoming which needs to be addressed in treating osteomyelitis by using drug delivery systems (DDS). The aim of this study was to develop a novel DDS for reducing initial burst release based on the combination of chitosan (CS) microspheres and calcium polyphosphate/chitosan/aldehyde alginate (CPP/CS/ADA) composite scaffolds. CS microspheres and CPP/CS/ADA composite scaffolds were characterized by FTIR spectroscopy, a laser particle size analyzer and SEM in order to reveal their composition, size distribution and surface morphology. Tetracycline hydrochloride (TC), as a model drug in the study, was encapsulated in CS microspheres, which further combined with CPP/CS/ADA composite scaffolds to form new DDS. Then the in vitro drug release was studied in detail. The kinetics of the drug release was also systematically studied. The results indicate that this new microspheres/scaffold compound system has a promising potential to reduce initial drug burst release effectively and prolong drug release time even for 30 days.
1 Introduction
Osteomyelitis can be caused by hematogenous or contiguous microbial seeding of the bone, and Staphylococcus aureus is considered the most common etiological agent.1–6 Therefore, delivering pharmaceutical agents to localized sites is useful for the treatment of chronic osteomyelitis.6–8 Tetracycline hydrochloride (TC), an antibiotic fighting bacteria in the body, is a common drug to treat osteomyelitis9 by using drug delivery systems (DDS).10,11 At present, various DDS, such as liposomes, micelles, microspheres and polymer/inorganic scaffolds,12 are widely investigated for this disease treatment, and biopolymer/ceramic scaffolds in drug delivery have achieved some remarkable progress13 due to their characteristics of high biodegradation behavior and good body response. Moreover, these composites as scaffolds for drug delivery can significantly reduce the initial burst release.
Usually, microspheres, such as chitosan14 (CS) microspheres, were designed as drug carriers by encapsulating the drug within the microspheres15 to enhance control of drug release rates. CS is a polysaccharide polymer comprising of copolymers of glucosamine and N-acetyl glucosamine. Compared with other polymers commonly used in drug controlled release field, CS possesses more bioactivities.16 CS has been used in the formulation of particulate DDS to achieve controllable drug delivery due to its biodegradation and biocompatibility. However, the flaws, including weak mechanical properties and burst release of the drugs,17 are inevitable when pristine biopolymers are used as drug carriers.
For biopolymer/ceramic scaffolds, biopolymers are physically or chemically mixed with ceramic to combine tailored degradability and high release efficiency of biopolymers with the osteoconductivity and sustained release characteristics of ceramic materials.18 Meanwhile, the mechanical and physical properties of biopolymers can compromise the brittleness of ceramic materials to obtain better handling properties. In recent years, these biopolymer/ceramic composites used as drug carriers for bone repair and regeneration have been widely researched.19–21 Although drug-loaded CS/hydroapatite (HA) composites deliver therapeutic agents in a sustained manner, HA is less resorbable in biological environment. Compared with these less resorbable materials, materials with good resorbability would have a slow and controlled drug release rate.22 Therefore, the resorbable materials are more suitable for the biopolymer/ceramic scaffolds used as drug carriers. As a class of CaP bio-ceramic, calcium polyphosphate (CPP) has been widely used as grafts for bone repair23 due to its controllable degradability, outstanding biocompatibility and biological activity. Therefore, drug-loaded CPP/CS composite scaffolds were prepared used as DDS for antibiotic treatment of osteomyelitis. These composites have shown a slow and controlled drug release rate. However, compared to microspheres/scaffold compound system, drug-loaded CPP/CS composite scaffolds had a relatively short drug-release time. Based on this, the CPP/CS composite scaffolds containing microspheres have been investigated in our laboratory.
In this study, drug-loaded CS microspheres were prepared by crosslinking-emulsion method with glutaraldehyde. Additionally, aldehyde alginate (ADA), a biological crosslinking agent, was also used in this research to crosslink CS/CPP scaffolds. Compared with traditional chemical crosslinking agent, ADA has little toxicity and can improve the biocompatibility of composites. As mentioned above, the CPP/CS/ADA scaffold containing CS microspheres was fabricated as a novel drug carrier. The characteristics and performance of this drug carrier were investigated and the influence factors of the drug release were also explored. Encapsulating the drug-loaded CS microspheres into biopolymer/ceramic scaffolds developed in this study is promising for controlled drug delivery, and the results in vitro release exhibited a sustained release for at least 30 days.
2 Materials and methods
2.1 Materials
Chitosan (CS, MW 5.0 × 105, medical grade, Shanghai Kabo Reagent Co.), and the degree of deacetylation is 80%. Sodium alginate (ALG, medical grade) was purchased from Yingfei Reagent Company (Qingdao, China). Tetracycline hydrochloride (TC) was obtained from Tian-yun Technology Ltd (Wuhan, China). Span-80 (S80, MW 4.3 × 102), petroleum ether, chloroform, glutaraldehyde and dispersion medium (liquid paraffin) were purchased from Chengdu Chemical Reagent Factory. CPP was prepared by our laboratory.
2.2 Fabrication of TC-loaded CS microspheres
TC-loaded CS microspheres were prepared by crosslinking-emulsion method. Briefly, drug-loaded CS microspheres were prepared by dissolving a certain amount of CS in ethylic acid (2.0%, v/v). Then, TC, as the model drug, was added into this solution under vigorous stirring for about 30 min at room temperature. The TC-CS solution was subsequently poured into a 3-neck flask which had been mixed with appropriate liquid paraffin and emulsifier (SP80). Then glutaraldehyde was added dropwise into the 3-neck flask at room temperature and stirred with an electronic stirrer for 90 min. The formed emulsion was centrifuged and then washed (3 times) with petroleum ether to ensure that Span-80 was completely removed. Finally, the collected particles were washed (3 times) with chloroform to wipe off redundant liquid paraffin before drying in the watch glass to achieve the product.
2.3 Preparation of CPP scaffold containing TC-CS microspheres
To prepare CS composite scaffolds, CS powders were first dissolved in ethylic acid to form CS solution (w/v, 2%), and CPP (CPP–CS = 1
:
1, w/w) was added to the CS solution to form a uniform mixture. Then a range of TC-CS microspheres (0.1, 0.2, 0.3–0.5 g), were dispersed in ethanol, added into the above mixture and stirred at room temperature for 30 min. 10% ADA24 solution (w/v) was added into the prepared composite solution and stirred for 5 min. The sol was then poured into 24-well plates, frozen for 24 h at −20 °C and dried for 24 h in a vacuum freeze drier.
2.4 Encapsulation efficiency (EE) and drug loading (DL) of microspheres
During preparation process of drug-loaded microsphere, the fresh suspension was centrifuged and the amount of unincorporated drug in the supernatant was measured by UV-vis spectrophotometer at 350 nm (Shanghai Third Analysis Instrument Factory, China). The encapsulation efficiency (EE) and drug loading (DL) were calculated as follows:
CS microspheres:
EE (%) = (weight of initial TC fed − weight of TC in the supernatant)/weight of initial TC fed × 100 |
DL (%) = (weight of initial TC fed − weight of TC in the supernatant)/weight of dry microspheres × 100 |
2.5 Particle size analysis and morphology of microspheres
The size distribution of microspheres was analyzed by Laser Particle Size Analyzer (Mastersizer 2000, Malvern Co., Britain) and Scanning electron microscopy (JSM-5900LV, JEOL, Japan). The samples were placed on the slide and analyzed without further treatment. The particle diameters of about 100 microspheres were measured randomly.
2.6 Scanning electron microscopy of scaffolds
The samples were prepared by brittle failure and then sputtered with gold. The morphology of scaffolds was observed by scanning electron microscopy (SEM).
2.7 In vitro TC release studies
In vitro release of TC from all the samples (CS microspheres and CPP/CS/ADA composite scaffolds containing microspheres) was carried out in normal saline. The samples were placed in glass vials and immersed in 10 mL of saline which used as release medium, and then were continuously stirred at 37 °C. At a specified time interval (1 h, 2 h, 3–8 h, 10 h, 1 day, 2 days, 3–10 days, 11 days, 13 days, 15 days), 5 mL aliquots were withdrawn from the release medium and TC concentration was determined with UV-spectrophotometer at 350 nm. An equal volume of fresh medium was then added to the release medium after each time samples were withdrawn. Experimental data at each time point were expressed as mean for experiments repeated in triplicate, then the release amount of TC was calculated from an absorbance-concentration calibration curve which was plotted over the concentration range from 1 to 100 μg mL−1, and then TC release rate was obtained based on the ratio of the amount of TC in release medium to the initial amount of TC.
2.8 TC release kinetics
In order to understand the TC release mechanism, the results obtained were fit in 2 kinetic models: zero order and first order kinetics.where k0 is the zero-order rate constant expressed in units of concentration/time and t is the time. |
ln Qt = ln Q0 − k1t
| (2) |
where k1 is the first order constant.
The following plots were made; Qt vs. t (zero order kinetic model), log(Q0 − Qt) vs. t (first order kinetic model), where Qt is the total amount of drug released at time t and Q0 is the initial amount of the drug.
Further, in order to define a model which will be better fit for the formulation, the first 50% drug release data were fitted in Korsmeyer–Peppas25 equation.
3 Results and discussion
3.1 The optimization for TC-loaded CS microspheres
The TC-loaded microspheres were fabricated by the method of crosslinking emulsion. During such a process, some parameters – CS concentration (A/%), TC-to-CS mass ratio (B), dosage of emulsifiers (C/mL) and crosslinking agent (D/mL) – shown in Table 1, will substantially determine the properties of the microspheres. Hence, we optimized the processing parameters to increase the encapsulation efficiency and drug loading of the microspheres by orthogonal array experiment and range analysis. The results of the experiments were obtained from UV spectrophotometer at 350 nm and listed in Table 1.
Table 1 The results of orthogonal array experiment
Number |
A |
B |
C |
D |
DL/% |
EE/% |
Overall indicator |
1 |
1 |
1 |
1 |
1 |
37.9 |
41.43 |
58.62 |
2 |
1 |
1/2 |
2 |
2 |
26.5 |
59.6 |
56.30 |
3 |
1 |
1/3 |
3 |
3 |
27.8 |
63.7 |
59.65 |
4 |
2 |
1 |
2 |
3 |
38.7 |
78.3 |
77.85 |
5 |
2 |
1/2 |
3 |
1 |
35.6 |
65.3 |
68.25 |
6 |
2 |
1/3 |
1 |
2 |
36.3 |
80.3 |
76.45 |
7 |
3 |
1 |
3 |
2 |
36.5 |
75.6 |
74.30 |
8 |
3 |
1/2 |
1 |
3 |
30.5 |
78.9 |
69.95 |
9 |
3 |
1/3 |
2 |
1 |
34.3 |
76.3 |
72.45 |
k1 |
58.19 |
70.26 |
68.34 |
66.44 |
Overall indicator = DL + EE × 0.5 |
k2 |
74.18 |
64.83 |
68.87 |
69.02 |
k3 |
72.23 |
69.52 |
47.52 |
69.15 |
R |
14.05 |
5.42 |
21.35 |
2.71 |
|
|
|
According to Table 1, an optimum condition of 2% of CS concentration (A), one to one of TC-to-CS mass ratio (B), 2 mL of emulsifiers (C) and 3 mL of crosslinking agent (D) was determined. The encapsulation efficiency and drug loading under this formulation were 78.3 and 38.7%, respectively. The influence degree of these four factors to the CS microspheres (C > A > B > D) was obtained by range analysis and shown in Table 1.
3.2 Characterization of TC-loaded CS microspheres
Fig. 1 is the FTIR spectra of each sample. The peak at 3440 cm−1 indicated that amido and hydroxy groups remained which provided the binding affinity with TC. Judging from Fig. 1, for A and B, the peak at 2931 cm−1 represents the stretching vibrations of C–H and the doublet peaks at 1651 and 1600 cm−1 represents a bending vibration of NH2 of CS. For CS–glutaraldehyde (D, TC-CS microspheres), the peak at 1600 cm−1 become sharper and the peak at 1651 cm−1 virtually disappear proved that Schiff base was formed through the cross-linking process of amino with the aldehyde group of glutaraldehyde.
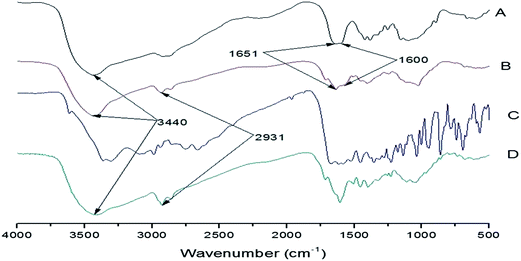 |
| Fig. 1 FTIR spectra of samples (A: CS; B: CS-blank microspheres; C: TC; D: TC-CS microspheres). | |
The average diameter and diameter distribution of TC-loaded CS microspheres are shown in Fig. 2. The average diameter of CS microspheres was 5.574 μm. The SEM image of the CS microsphere in Fig. 3 showed that CS microspheres with a diameter of 2–10 μm was in fine spherical shape with smooth surface without any aggregation or adhesion. These microspheres are hoped to enhance the ability of reducing initial burst release.
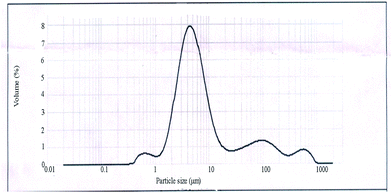 |
| Fig. 2 The size distribution figure of CS microspheres (about 3 spheres in the range of 0.1–1 μm, about 77 spheres in the range of 1–10 μm, about 12 spheres in the range of 10–100 μm and about 8 spheres in the range of 100–1000 μm). | |
 |
| Fig. 3 The SEM images of CS microspheres A: ×1000; B: ×5000. | |
3.3 The drug-release behaviors of CS microspheres
The in vitro release profiles of TC-CS microspheres are presented in Fig. 4. During the first 8 h, the % drug release was about 40%. Such an initial burst release was mostly due to the drug originally absorbed on the tight surface of the microspheres. After 8 h slightly initial burst, the cumulative drug-release profiles maintained a sustained release. For all samples, the TC-loaded CS microspheres exhibit a remarkable sustained-release property. However, the drug release rate of microspheres began to increase rapidly after 20 days, which might be due to the degradation of CS.
 |
| Fig. 4 TC release curve of TC-CS microspheres. | |
3.4 Morphology of scaffolds
The SEM micrographs of the fractured surface of the porous scaffolds are shown in Fig. 5. It demonstrated that the samples possessed highly porous structures with evenly distributed and interconnected pores. The shape of pore was close to roundness, and the pore diameter ranged from 50 to 400 μm. It was also obvious that the pores didn't change significantly after the addition of TC-CS microspheres (Fig. 5c). Furthermore, TC-CS microspheres in scaffold were uniform distributed.
 |
| Fig. 5 The micrographs of the blank scaffold and scaffold containing microspheres: (A) CS/CPP (×100), (B) CS/CPP (×500), (C) TC-microspheres/CS/CPP (×100) and (D) TC-microspheres/CS/CPP (×500). | |
3.5 The drug-release behaviors of microspheres/scaffold compound system
The CS/CPP/ADA blank scaffolds containing TC-loaded CS microspheres were used for exploring the behavior of drug release. Fig. 6 gives the cumulative drug-release curves of the hybrid scaffolds with different CS microspheres compositions (0.1, 0.2, 0.3–0.5 g) called CS1, CS2 and CS3–CS5 in Table 2. As shown in Fig. 6, this compound system prolonged the release profile of TC and the blank scaffolds with the higher content (0.4–0.5 g) of CS microspheres had a better release effect. This may be related to the following reasons: TC-loaded CS microspheres were encapsulated in blank scaffold and thus blocked drug release.
 |
| Fig. 6 Release profiles of CS/CPP compound containing CS microspheres. | |
Table 2 Release rate constants kx, correlation coefficients (R2) calculated after fitting the release profiles obtained using different mathematical models
Formulation code |
Zero order |
First order |
R2 |
k0 |
R2 |
k1 |
CS |
0.8931 |
2.0564 |
0.7856 |
0.0135 |
CS1 |
0.8273 |
1.8600 |
0.7742 |
−0.0581 |
CS2 |
0.7758 |
1.5930 |
0.7015 |
−0.0677 |
CS3 |
0.7902 |
1.5594 |
0.6988 |
−0.0704 |
CS4 |
0.8306 |
1.6055 |
0.7891 |
−0.0592 |
CS5 |
0.6843 |
1.1189 |
0.6643 |
−0.0509 |
In order to determine the release model which could better describe the pattern of drug release, the in vitro release data (the range of % drug release is 20–80%) were fitted to models representing zero-order and first-order model. The release constants were calculated from the slope of the appropriate plots, and regression coefficient (R2) was determined by linear regression analysis and the results were tabulated in Table 2. According to the results, the related correlation coefficients of zero-order were better than first-order kinetics.
In order to better understand the release mechanism of the encapsulated TC from the hybrid scaffolds, we analyzed hybrid scaffolds which containing 0.5 g CS microspheres. According to Korsmeyer–Peppas index model: log
Q(t) = m + n
log
t, where Q(t) is the fractional TC release (the percentage of the total TC amount incorporated into the scaffold), t is the TC release time, m (m = log
k) is a constant related to the properties of the scaffold and the drug, and n is the release exponent that depends on the transport mechanism and the geometry of the device. According to this classification, there are two distinguishable modes of diffusion: (i) a value of n < 0.45 indicates drug release according with Ficks diffusion mechanism; (ii) if 0.45 < n < 0.89, the release of drug is coupled Fickian diffusion–erosion mechanism. From n = 0.5217, we can infer that the compound system release behavior belongs to case (ii). Compared to case (i), the higher value of n in case (ii) illustrates the closer correlation between drug-release and scaffolds. Such an interaction might slow down the degradation of scaffold and further prolong release time.26
4 Conclusions
In this study, we focused on developing a novel drug delivery system for reducing initial burst release based on the combination of microspheres and scaffolds. These results showed microspheres/scaffold compound system could reduce TC initial burst release effectively and prolong drug release time even for 30 days. The reason for these results might be the fact that the microspheres combined with composite scaffolds can embed drug well and evidently minimize administration frequency. Accordingly, this microspheres/scaffold compound system might possess a promising potential as a controlled release carrier.
Acknowledgements
The authors would like to thank the Scientific and Technological Project of Sichuan Province (no. 2012SZ0015) and the Scientific Research Project of Department of Public Health of Sichuan Province (no. 100194).
References
- I. G. Sia and E. F. Berbari, Best Pract. Res., Clin. Rheumatol., 2006, 20, 1065–1081 CrossRef PubMed.
- L. S. Jorge, A. G. Chueire and A. R. Rossit, Braz. J. Infect. Dis., 2010, 14, 310–315 CrossRef.
- P. Tattevin, A. C. Cremieux, P. Pottier, D. Huten and C. Carbon, Clin. Infect. Dis., 1999, 29, 292–295 CAS.
- S. L. Kaplan, Infect. Dis. Clin. North Am., 2005, 19, 787–797 CrossRef PubMed.
- M. E. Shirtliff and J. T. Mader, Clin. Microbiol. Rev., 2002, 15, 527–544 CrossRef.
- M. S. Rouse, K. E. Piper, M. Jacobson, D. J. Jacofsky, J. M. Steckelberg and R. Patel, J. Antimicrob. Chemother., 2006, 57, 301–305 CrossRef CAS PubMed.
- C. Vilhena and A. Bettencourt, Mini-Rev. Med. Chem., 2012, 12, 202–209 CrossRef CAS.
- J. K. Smith, A. R. Moshref, J. A. Jennings, H. S. Courtney and W. O. Haggard, Clin. Orthop. Relat. Res., 2013, 471, 3158–3164 CrossRef PubMed.
- H. S. Fraimow, Semin. Plast. Surg., 2009, 23, 90–99 CrossRef PubMed.
- G. Wei, Y. Kotoura, M. Oka, T. Yamamuro, R. Wada, S. H. Hyon and Y. Ikada, J. Bone Jt. Surg., Br. Vol., 1991, 73, 246–252 CAS.
- K. Kawanabe, Y. Okada, Y. Matsusue, H. Iida and T. Nakamura, J. Bone Jt. Surg., Br. Vol., 1998, 80, 527–530 CrossRef CAS PubMed.
- L. Zhang, D. Pornpattananangku, C. M. Hu and C. M. Huang, Curr. Med. Chem., 2010, 17, 585–594 CrossRef CAS.
- A. R. Boccaccini, S. Keim, R. Ma, Y. Li and I. Zhitomirsky, J. R. Soc., Interface, 2010, 7(suppl. 5), S581–S613 CrossRef CAS PubMed.
- V. R. Sinha, A. K. Singla, S. Wadhawan, R. Kaushik, R. Kumria, K. Bansal and S. Dhawan, Int. J. Pharm., 2004, 274, 1–33 CrossRef CAS PubMed.
- R. Pandey and G. K. Khuller, J. Antimicrob. Chemother., 2004, 53, 635–640 CrossRef CAS PubMed.
- K. Oungbho and B. W. Muller, Int. J. Pharm., 1997, 156, 229–237 CrossRef CAS.
- J. H. Lee, B. S. Chang, U. O. Jeung, K. W. Park, M. S. Kim and C. K. Lee, Clin. Orthop. Surg., 2011, 3, 238–244 CrossRef PubMed.
- J. H. Lee, B. S. Chang, U. O. Jeung, K. W. Park, M. S. Kim and C. K. Lee, Clin. Orthop. Surg., 2011, 3, 238–244 CrossRef PubMed.
- H. Ueda, T. Nakamura, M. Yamamoto, N. Nagata, S. Fukuda, Y. Tabata and Y. Shimizu, J. Controlled Release, 2003, 88, 55–64 CrossRef CAS.
- A. Hokugo, M. Ozeki, O. Kawakami, K. Sugimoto, K. Mushimoto, S. Morita and Y. Tabata, Tissue Eng., 2005, 11, 1224–1233 CrossRef CAS PubMed.
- Y. Takahashi, M. Yamamoto and Y. Tabata, Biomaterials, 2005, 26, 4856–4865 CrossRef CAS PubMed.
- Y. Zhang and M. Q. Zhang, J. Biomed. Mater. Res., 2002, 62, 378–386 CrossRef CAS PubMed.
- J. C. Lee, M. F. Hou, H. W. Huang, F. R. Chang, C. C. Yeh, J. Y. Tang and H. W. Chang, Cancer Cell Int., 2013, 13, 55 CrossRef PubMed.
- Y. Xu, L. Li, X. Yu, Z. Gu and X. Zhang, Carbohydr. Polym., 2012, 87, 1589–1595 CrossRef CAS PubMed.
- R. W. Korsmeyer, R. Gurny, E. M. Doelker, P. Buri and N. A. Peppas, Int. J. Pharm., 1983, 15, 25–35 CrossRef CAS.
- A. Budhian, S. J. Siegel and K. I. Winey, Int. J. Pharm., 2008, 346, 151–159 CrossRef CAS PubMed.
|
This journal is © The Royal Society of Chemistry 2014 |