DOI:
10.1039/C4RA03441D
(Paper)
RSC Adv., 2014,
4, 34350-34355
Coupling interaction of cathodic reduction and microbial metabolism in aerobic biocathode of microbial fuel cell†
Received
16th April 2014
, Accepted 16th June 2014
First published on 18th June 2014
Abstract
Certain mixed consortia colonized on aerobic biocathodes can improve the 4-electron oxygen reduction of cathodes; however, the coupling interaction of the cathodic reaction and microbial metabolism remains unclear. To better understand the abovementioned interaction evolved in the cathodic process, biocathodes were enriched using nitrifying sludge and operated at various NH4Cl and NaHCO3 concentrations in both the open and closed external circuit conditions. Based on the variation of the nitrification and cathodic oxygen reduction activities, it was shown that the oxygen reduction process, to some extent, relied on the nitrification activity of the biocathode; the external electrons from the cathode, in turn, might benefit the nitrifying bacteria selected in the MFC habitat by entering the electron transfer chains as the energy source. Nitrifiers, including Nitrosomonas sp., Nitrospira sp. and Nitrobacter sp., were detected in all the biocathodes that were cultured in different conditions, even the ones cultured without NH4Cl in the medium. These findings provided valuable insights into the possible working mechanism of biocathodes.
Introduction
Microbial fuel cells (MFC) are promising devices that can directly harvest electricity from organic pollutants in wastewater.1,2 At the anode, electrochemical active bacteria consume organic substrates, gain energy for growth and maintenance and transfer electrons to the anode electrodes, which then travel to the cathode through an external circuit; at the cathode, electrons are consumed by electron acceptors, such as oxygen (in most of the cases), to complete electricity production. Platinum catalyst is widely employed to reduce the overpotential of the cathode reactions; however, it suffers from high cost and short service life because of loss, agglomeration and poisoning. The advent of biocathodes has shed light on the lower-cost, efficient and sustainable construction and operation of MFCs. The electron acceptors of biocathodes are also extended to a variety of substrates, e.g. oxygen,3 nitrate,4 sulfate,5 tetrachloroethene,6 CO2,7 Cr(VI),8 U(VI)9 and dyes10,11 in wastewater.
Biocathode has attracted considerable attention for its advantages but studying the working mechanism still remains a big challenge for researchers. The working process of a biocathode is a complex combination of cathode reaction and metabolism and the multiplication of bacteria. It is widely accepted that extracellular electrons evolve in microbial metabolism of biocathodes via direct or indirect electron transfer pathways.12–14 Although the electrochemical active bacteria of a biocathode can be selected in a natural environment, the metabolism activity of these bacteria that is stimulated by extracellular electrons in the MFC habitat should be different from that in a natural circumstance. Ferrous oxidizing bacteria (FeOB) have been utilized in biocathodes. Originally, Fe(III) was considered as the only electron acceptor in cathodes, while the role of FeOB was to recycle Fe(II) in the catholyte, which was reduced to Fe(III) by external electrons.15–18 Later, Carbajosa et al. found that Acidithiobacillus ferrooxidans exhibited redox characteristic without Fe(II) in the solution and could grow using external electrons as the sole energy source.19,20 Their report indicated that extracellular electrons may influence and support microbial activity, which inspired us to further explore the working mechanism involved in biocathodes.
|
 | (1) |
|
 | (2) |
We carried out the research considering a nitrifying biocathode as an example. In the nitrifying biocathode process, two main reactions occur simultaneously during the microbial metabolism process: cathodic reaction (eqn (1)) and nitrification (eqn (2)). Our aim was to explore the complex interaction between the two reactions in the biocathode process. We first enriched the biocathode using a nitrifying sludge and operated the biocathode in different NaHCO3 and NH4Cl concentrations in open or closed circuit conditions, aiming to evaluate the variations of the oxygen reduction and nitrification activities in the biocathode process. The nitrate formation kinetics was used to evaluate the nitrification activity of the biocathode, while the voltage and power outputs of the MFC were used to represent the activity of the cathodic reaction. Microbial communities under different culture conditions were also evaluated, providing a further understanding of the biocathode working mechanism.
Materials and methods
Process setup and operation
Two-chambered reactors were constructed. Both anode and cathode chambers were cylindrical rooms of the same sizes (4 cm long and 3 cm in diameter; 28 mL liquid volume). Carbon fibre brushes, heat treated in a muffle furnace at 450 °C for 30 min, were used as both anode and cathode electrodes.21 A homogeneous anion exchange membrane (Qianqiu Co. Ltd., China) was used to separate the anode and cathode chambers.
The anode chambers were inoculated with an effluent of glucose-fed MFCs and the details were described previously.22 The cathode chambers were inoculated with municipal wastewater (50%, v/v) collected on the HIT campus, and the medium contained NaHCO3 (1.0 g L−1) and NH4Cl (0.3 g L−1) during the startup period. A phosphate buffered solution (KCl 0.13 g L−1, NaH2PO4·2H2O 3.32 g L−1 and Na2HPO4·12H2O 10.32 g L−1), tracer minerals and vitamins were added in the medium solution as described previously.23 The anode was sealed with a rubber plug to maintain anaerobic conditions, except during the replacement of the anolyte, while the cathode was continuously aerated with a pump during the experiment (DO 5.0 ± 0.3 mg L−1). The anode and cathode chambers were simultaneously refilled with the inoculation medium every 24 h until a stable voltage was achieved. The external resistance was maintained at 1000 Ω, except where otherwise mentioned. All the tests were conducted at a constant temperature of 30 °C. To avoid the growth of algae and photosynthetic bacteria, the MFC reactors were wrapped with aluminium foil.
After successful enrichment, the anodes of all the MFCs were operated with the same substrate (glucose 1.0 g L−1 and NH4Cl 0.1 g L−1), while the cathodes were operated under different culture conditions to investigate the performance of nitrification and oxygen reduction processes. The cathode substrate for the first group (MFC-1) was composed of 0.3 g L−1 NH4Cl and NaHCO3 at different concentrations (1.0, 0.5 and 0 g L−1). The second group (MFC-2) was cultured with a fixed concentration of NaHCO3 (1.0 g L−1) but with different NH4Cl concentrations (0.3, 0.1 and 0 g L−1). The third group (MFC-3) was operated with a fixed substrate containing NaHCO3 1.0 g L−1 and NH4Cl 0.3 g L−1 but with the external circuit connected to 1000 Ω or kept open (Table 1). All the experiments were duplicated for repeatability.
Table 1 Culture conditions of biocathode MFC
|
NaHCO3 concentration, g L−1 |
NH4Cl concentration, g L−1 |
External circuit |
MFC-1 |
1.0 |
0.5 |
0 |
0.3 |
1000 Ω |
MFC-2 |
1.0 |
0.3 |
0.1 |
0 |
1000 Ω |
MFC-3 |
1.0 |
0.3 |
1000 Ω |
Open-circuit |
Calculations and analysis
The cell voltages (V) were recorded with a data acquisition board every 30 min. Power density, polarization curves, coulombic efficiency (CE) and total electric quantity (Q) were obtained as described previously.24
The samples obtained from the cathode and anode compartments were immediately centrifuged (10
000 × g) to remove the biomass. The chemical oxygen demand (COD), NH4+–N, NO2−–N and NO3−–N were measured according to the standard methods of the American Public Health Association.25 The total nitrogen (TN) was calculated as the sum of NH4+–N, NO2−–N and NO3–N.
Microbial community analysis
The biofilm samples of the biocathodes were collected by removing a small amount of carbon brush fibre with sterile scissors from the cathode materials that were operated stably at different culture conditions. Sample S1 was collected from MFC-1 fed with NaHCO3 1.0 g L−1 and NH4Cl 0.3 g L−1 in the closed circuit condition for ca. 15 cycles. Sample S2 was collected from MFC-2 fed with NaHCO3 1.0 g L−1 but not NH4Cl in the closed circuit condition after ca. 15 cycles. Sample S3 was collected from MFC-3 fed with NaHCO3 1.0 g L−1 and NH4Cl 0.3 g L−1 in the open circuit condition after ca. 21 cycles. DNA extraction and PCR amplification, purification and cloning were carried out as previously described.26 16S rRNA gene sequences were analyzed at the National Center for Biotechnology Information (http://blast.ncbi.nlm.nih.gov/blast.cgi). A sequence similarity of >97% was defined as an operational taxonomic unit (OTU).
Data deposition
All the sequences used in this study have been deposited in the NCBI database. The 16S rRNA gene sequences were deposited in GenBank with accession numbers KJ627743–KJ627767.
Results
Interactions of nitrification and O2 reduction reaction depending on NaHCO3 concentrations
Nitrifying bacteria and most of the biocathode bacteria are autotrophic microorganisms utilizing inorganic carbon, such as HCO3−, CO32− and CO2, as a carbon source for growth.27 The results demonstrated that both nitrification and cathodic activity decreased as the initial NaHCO3 concentrations in the cathode chamber declined from 1.0 g L−1 to 0 g L−1 (Fig. 1). The average nitrate formation rates were ca. 6.08, 4.91 and 3.02 mg (L−1 h−1) and the maximum power outputs were 1191, 1070 and 1013 mW m−2 for the initial NaHCO3 concentrations of 1.0, 0.5 and 0 g L−1, respectively. The polarization curves showed that the effect of NaHCO3 concentrations was more obvious at current densities higher than 2.0 A m−2. The maximum current density (3.988 A m−2) was obtained for a bicarbonate concentration of 1.0 g L−1, followed by 0.5 g L−1 (2.898 A m−2) and 0 g L−1 (2.735 A m−2). Because the anode potential showed a slight difference with the variation of NaHCO3 concentrations, the difference in the power generation was mainly caused by the cathode performance (Fig. 1c).
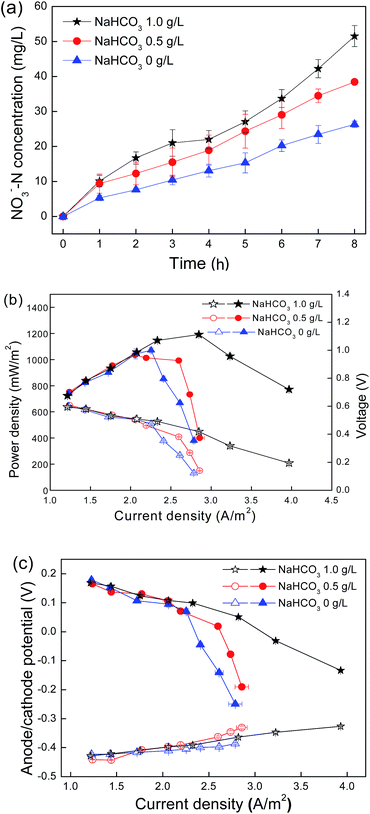 |
| Fig. 1 (a) Nitrate concentrations in the cathode chamber during the initial 8 h of a cycle; (b) power density (filled symbol) and polarization (open symbol) curves; (c) anode potential (open symbol) and cathode potential (filled symbol) (vs. Ag/AgCl) of the nitrifying biocathode MFC cultured with different bicarbonate concentrations. | |
Interactions of nitrification and O2 reduction reaction influenced by NH4Cl concentrations
Nitrifiers, including ammonia-oxidizing bacteria (AOB) and nitrite-oxidizing bacteria (NOB), obtain energy for growth from the oxidation of inorganic nitrogen such as ammonia and nitrite. The amounts of ammonia in the medium were, therefore, fixed to affect the activity and quantity of nitrifiers in the cathode. In this work, a cathode substrate was composed of different NH4Cl concentrations (0.3, 0.1 and 0 g L−1) with a fixed concentration of NaHCO3 (1.0 g L−1). Power generation decreased with the decline of the initial NH4Cl concentrations (Fig. 2a). As NH4Cl concentration reached 0.3 g L−1, the MFC delivered the maximum power output of 1098 mW m−2 at a current density of 3.26 A m−2. As the NH4Cl concentration reduced to 0.1 g L−1, the power generation declined to 989 mW m−2. When no NH4Cl was supplied to the cathode microorganism, the maximum power generation was 797 mW m−2. The variation in the power generation was mainly caused by the cathode performance, which was confirmed by the anode and cathode potentials during the polarization test (Fig. 2b).
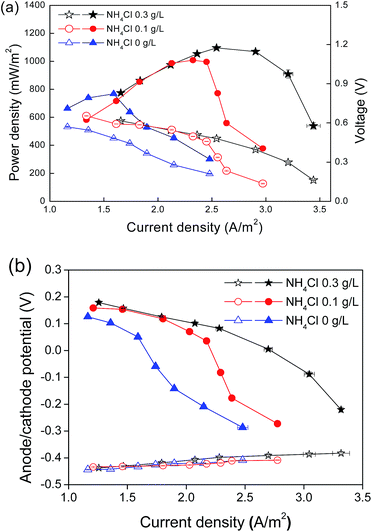 |
| Fig. 2 (a) Power density (filled symbol) and polarization (open symbol) curves; (b) anode potential (open symbol) and cathode potential (filled symbol) (vs. Ag/AgCl) of the nitrifying biocathode MFC cultured with different NH4Cl concentrations. | |
Nitrification activity influenced by cathodic reaction
As the nitrification activity significantly affected the cathode catalysis activity, to address the influence of the cathodic reaction on the nitrification activity, nitrate formation kinetics were studied with the external circuit of the biocathode MFC connected to 1000 Ω or disconnected (Fig. 3). As the external circuit connected to 1000 Ω, the nitrate concentration in the early 8 h of the cycle was 25.7 mg L−1 and the nitrification rate was 2.838 mg (L−1 h−1). Because the external circuit was opened, the nitrification dynamics showed two different tendencies: first, slight declined to 1.614 mg (L−1 h−1) in the first 7 cycles and dramatic increase to 5.322 mg (L−1 h−1) after the circuit remained open for more than 21 cycles.
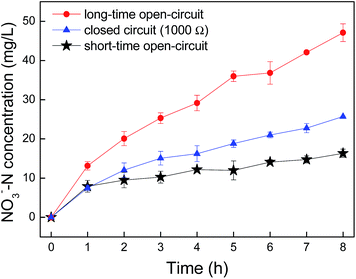 |
| Fig. 3 Nitrate formation dynamics during the initial 8 h of a cycle with external circuit connected to 1000 Ω or open ( for MFC with circuit connected to 1000 Ω; ● for MFC with circuit open for less than 7 cycles; ▲ for MFC with circuit open for more than 21 cycles). | |
Analysis of microbial community
To investigate phylogenetic differentiation depending on NH4Cl as the energy source for nitrifiers and external circuit conditions, the biofilm attached on the biocathode was analyzed by gene libraries. For S1, S2 and S3, the positive clones were 99, 99 and 98, grouped into operating taxonomic units (OTUs) of 17, 29 and 18, respectively, on the basis of more than 97% sequence similarity within an OTU. The coverage for the samples are 90.8%, 83.8% and 87.8%, respectively, indicating that the three clone libraries could reflect the microbial community composition in the samples (Fig. 4).
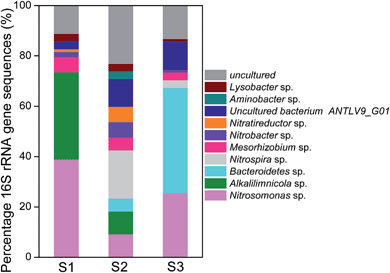 |
| Fig. 4 Bacterial community composition and relative abundance of nitrifying biocathodes determined from 16S rRNA gene libraries (S1: the biocathode cultured with NH4Cl in closed circuit condition; S2: the biocathode cultured without NH4Cl in closed circuit condition; S3: the biocathode cultured with NH4Cl in open circuit condition). | |
The most abundant sequence in all the three samples belonged to Proteobacteria, which was found to be colonized on both the anode and cathode electrodes and generally called “exoelectrogens”.14,28 The ratios of Proteobacteria were S1 89.8%, S2 44.4% and S3 34.7%. The nitrifiers, including Nitrosomonas sp., Nitrospira sp. and Nitrobacter sp., were detected in the three samples. The ratios of the total nitrifiers were S1 40.8%, S2 34.3% and S3 29.6%. The highest abundance of Nitrosomonas sp. was 38.8% (S1), followed by 25.5% (S3) and 9.1% (S2). Nitrite-oxidizing bacteria (NOB), including Nitrospira sp. and Nitrobacter sp., accounted for a small fraction (S1 2.0% and S3 4.1%); S2 with the lowest abundance of AOB (9.1%) showed the highest ratio of NOB (25.3%). Even though the abundance of NOB was significantly lower than that of AOB in S1 and S3, the accumulation of NO2− was not observed in the catholyte. At the end of each cycle, ca. 99% of ammonia was converted into nitrate. This was probably because ammonia oxidation was often the rate-limiting step of the total nitrification process and nitrite rarely accumulated in most of the environments.29 Alkalilimnicola sp. was also detected in S1 (34.7%) and S2 (9.1%) but not detected in S3.
Discussion
The cathodic reaction is promoted by electrochemically active bacteria in the biocathode, where metabolism and the multiplication of microorganisms occur simultaneously. Competitive and synergetic relations exist between the two reactions. In the case of the nitrifying biocathode, there is a competition for oxygen that is essential for both nitrification and the 4-electron oxygen reduction of the cathode;30,31 proton consumption by a cathodic reaction (eqn (1)) and proton reproduction by biological nitrification (eqn (2)), which benefit each other by maintaining a pH balance in the cathode chamber.32 In our work, the interactions of the cathodic reaction and nitrification were carried out in terms of the substrate concentration and external circuit conditions. Nitrifying bacteria are affiliated with chemoautotrophic microorganisms;33,34 therefore, the availability of an inorganic carbon source and an inorganic energy source affects the metabolism and multiplication of bacteria.35 In our work, the variations of the MFC performance and nitrification rate towards changes in the substrate demonstrated that the cathodic activity was strongly correlated to the microbial activity of the bacteria. With a higher nitrification activity, the cathodic reaction rate was also faster.
In addition, the cathodic reaction does not passively rely on the microbial activity of nitrifying bacteria, but influences the nitrification activity by extracellular electron transfer (EET).36,37 The evidence in our work involved the differences of the nitrification rate with the external circuit kept closed and open (Fig. 3). With the external circuit changing from close to open, the rapid increase in the nitrification activity was expected because the oxygen competition from the cathodic reaction was terminated. However, the obvious decrease of the nitrification rate was observed in the early stage of the open-circuit condition. One possible explanation was that the external electrons may benefit the microorganisms as an energy source by entering the electron transport chains; once the electron transfer was terminated, the activity of the nitrifying bacteria declined. Liang et al. also reported an accelerated reduction in the chlorinated nitroaromatic antibiotic chloramphenicol (CAP) by the biocathode bioelectrochemical system (BES) connected to an external circuit compared with that in the open circuit condition.38 The dramatic increase of the nitrification rate after the long-term open-circuit condition in our work may be due to the microbial community changes caused by the external circuit; the nitrifying bacteria that were suppressed in the MFC condition multiplied quickly when the external circuit became open and caused a dramatic increase in the nitrification rate.
The study of microbial community offered another deep insight into the working mechanism of biocathodes. Proteobacteria are considered to be responsible for electron transfer in biocathodes.14,28 The voltage and power outputs were correlated with the abundances of Proteobacteria in S1, S2 and S3. The highest voltage output was achieved with the same culture condition as S1, followed by S2 and the MFC operated in the open circuit condition as S3 showed the lowest voltage output (Fig. S1, ESI† and Fig. 2b).
The bacteria in biocathodes can be selected in a natural environment but the living habitat in the MFC is fairly different. Nitrifying bacteria obtain their energy from the oxidation of ammonia and nitrite in a natural environment; however, in the MFC habitat, the abundance of nitrifying bacteria was influenced by both substrate concentration and external electron transfer. Nitrifying bacteria were detected in S2, which was cultured without NH4Cl in the closed circuit condition for more than 15 cycles. Nitrifying bacteria in S2 accounted for 34.3%, even higher than S3 (29.6%) that was cultured with NH4Cl in the open circuit condition for other parallel conditions such as NaHCO3, DO and pH. These results suggested that nitrifying bacteria may survive by using external electrons as the energy source in the MFC habitat, except for the energy from ammonia and nitrite oxidation. This hypothesis was also supported by the changes in the nitrification rates in close and open circuit conditions (Fig. 3). Carbajosa et al. also reported the growth of the acidophile bacterium At. ferrooxidans directly on a graphite electrode using an applied voltage of 0 V as the only energy source without adding redox mediators to the solution.19 Sorokin et al. reported the versatile metabolism of Alkalilimnicola sp. with nitrate as the electron acceptors and H2 or polysulfide as the electron donors.39 However, in our work, no organic or inorganic species that Alkalilimnicola sp. could utilize existed in the cathode. We assumed that Alkalilimnicola sp. might be also responsible for the cathodic reaction and utilize the electrons from the cathode as an energy source. This may explain why Alkalilimnicola sp. disappeared in S3 when the external circuit was cut off. Isolation and electrochemical studies regarding Alkalilimnicola sp. are ongoing in our lab.
For a nitrifying biocathode, the activity of the oxygen reduction reaction was, to some extent, dependent on the nitrification activity: the activity of the oxygen-reducing reaction increased as the nitrification activity increased. The external electrons derived from the cathode, in turn, may benefit nitrifying bacteria selected in the MFC habitat by entering the electron transport chain as another energy source besides the oxidation of ammonia and nitrite. The microbial community analysis confirmed the hypothesis on the interaction of nitrification and cathodic reaction in the biocathode.
Conclusions
For a nitrifying biocathode, the activity of oxygen reduction reaction was, to some extent, dependent on the nitrification activity: the activity of oxygen reduction increased as the nitrification activity increased. The external electrons derived from the cathode may, in turn, benefit the nitrifying bacteria selected in the MFC habitat by entering the electron transport chain as another energy source besides the oxidation of ammonia and nitrite. A microbial community analysis indicated that the nitrifiers might be able to catalyze oxygen reduction reaction and utilize the external electrons as an energy source.
Acknowledgements
This work was supported by the State Key Laboratory of Urban Water Resource and Environment, Harbin Institute of Technology (Grant no. 2013DX08) and by the National Natural Science Fund for Distinguished Young Scholars (Grant no. 51125033) and National Natural Science Fund of China (Grant no. 51209061). The authors also acknowledge the supports from the Creative Research Groups of China (Grant no. 51121062) and the International Cooperating Project between China and Canada (Grant no. S2012GR01820).
Notes and references
- Y. J. Feng, X. Wang, S. A. Cheng, M. D. Merrill, T. Saito and B. E. Logan, Environ. Sci. Technol., 2009, 43, 6870–6874 CrossRef.
- J. A. Sun, Z. Bi, B. Hou, Y. Q. Cao and Y. Y. Hu, Water Res., 2011, 45, 283–291 CrossRef CAS PubMed.
- A. Ter Heijne, O. Schaetzle, S. Gimenez, F. Fabregat-Santiago, J. Bisquert, D. P. B. T. B. Strik, F. Barriere, C. J. N. Buisman and H. V. M. Hamelers, Energy Environ. Sci., 2011, 4, 5035–5043 CAS.
- K. Y. Cheng, M. P. Ginige and A. H. Kaksonen, Environ. Sci. Technol., 2012, 46, 10372–10378 CAS.
- L. Pons, M. L. Delia and A. Bergel, Bioresour. Technol., 2011, 102, 2678–2683 CrossRef CAS PubMed.
- F. Aulenta, P. Reale, A. Canosa, S. Rossetti, S. Panero and M. Majone, Biosens. Bioelectron., 2010, 25, 1796–1802 CrossRef CAS PubMed.
- X. X. Cao, X. Huang, P. Liang, N. Boon, M. Z. Fan, L. Zhang and X. Y. Zhang, Energy Environ. Sci., 2009, 2, 498–501 CAS.
- L. P. Huang, X. L. Chai, G. H. Chen and B. E. Logan, Environ. Sci. Technol., 2011, 45, 5025–5031 CrossRef CAS PubMed.
- K. B. Gregory and D. R. Lovley, Environ. Sci. Technol., 2005, 39, 8943–8947 CrossRef CAS.
- S. Kalathil, J. Lee and M. H. Cho, New Biotechnol., 2011, 29, 32–37 CrossRef CAS PubMed.
- S. Kalathil, J. Lee and M. H. Cho, Bioresour. Technol., 2012, 119, 22–27 CrossRef CAS PubMed.
- M. Rosenbaum, F. Aulenta, M. Villano and L. T. Angenent, Bioresour. Technol., 2011, 102, 324–333 CrossRef CAS PubMed.
- L. Huang, J. M. Regan and X. Quan, Bioresour. Technol., 2011, 102, 316–323 CrossRef CAS PubMed.
- D. R. Lovley, Curr. Opin. Biotechnol., 2008, 19, 564–571 CrossRef CAS PubMed.
- Y. Mao, L. Zhang, D. Li, H. Shi, Y. Liu and L. Cai, Electrochim. Acta, 2010, 55, 7804–7808 CrossRef CAS PubMed.
- A. Ter Heijne, H. V. M. Hamelers and C. J. N. Buisman, Environ. Sci. Technol., 2007, 41, 4130–4134 CrossRef CAS.
- A. Lopez-Lopez, E. Exposito, J. Anton, F. Rodriguez-Valera and A. Aldaz, Biotechnol. Bioeng., 1999, 63, 79–86 CrossRef CAS.
- Z. Lewandowski, A. Rhoads and H. Beyenal, Environ. Sci. Technol., 2005, 39, 4666–4671 CrossRef.
- S. Carbajosa, M. Malki, R. Caillard, M. F. Lopez, F. J. Palomares, J. A. Martin-Gago, N. Rodriguez, R. Amils, V. M. Fernandez and A. L. De Lacey, Biosens. Bioelectron., 2010, 26, 877–880 CrossRef CAS PubMed.
- J. C. Thrash and J. D. Coates, Environ. Sci. Technol., 2008, 42, 3921–3931 CrossRef CAS.
- Y. Feng, Q. Yang, X. Wang and B. E. Logan, J. Power Sources, 2010, 195, 1841–1844 CrossRef CAS PubMed.
- X. Wang, Y. Feng, J. Liu, H. Lee, C. Li, N. Li and N. Ren, Biosens. Bioelectron., 2010, 25, 2639–2643 CrossRef CAS PubMed.
- D. R. Lovley and E. J. P. Phillips, Appl. Environ. Microbiol., 1988, 54, 1472–1480 CAS.
- B. E. Logan, S. Cheng and H. Liu, Environ. Sci. Technol., 2006, 40, 2426–2432 CrossRef.
- APHA, Standard Methods for the Examination of Water and Wastewater, American Public Health Association, Washington DC, USA, 1999 Search PubMed.
- Y. Qu, Y. Feng, J. Liu, W. He, X. Shi, Q. Yang, J. Lv and B. E. Logan, Desalination, 2013, 317, 17–22 CrossRef CAS PubMed.
- X. Xia, Y. Sun, P. Liang and X. Huang, Bioresour. Technol., 2012, 120, 26–33 CrossRef CAS PubMed.
- T. P. Sciarria, A. Tenca, A. D'Epifanio, B. Mecheri, G. Merlino, M. Barbato, S. Borin, S. Licoccia, V. Garavaglia and F. Adani, Bioresour. Technol., 2013, 147, 246–253 CrossRef CAS PubMed.
- K. Isobe, K. Koba, S. Otsuka and K. Senoo, J. For. Res., 2011, 16, 351–362 CAS.
- S. Puig, M. Serra, A. Vilar-Sanz, M. Cabre, L. Baneras, J. Colprim and M. Dolors Balaguer, Bioresour. Technol., 2011, 102, 4462–4467 CrossRef CAS PubMed.
- J. Keller, B. Virdis, K. Rabaey and Z. Yuan, Water Res., 2008, 42, 3013–3024 CrossRef PubMed.
- S. J. You, N. Q. Ren, Q. L. Zhao, P. D. Kiely, J. Y. Wang, F. L. Yang, L. Fu and L. Peng, Biosens. Bioelectron., 2009, 24, 3698–3701 CrossRef CAS PubMed.
- A. E. Ingalls, S. R. Shah, R. L. Hansman, L. I. Aluwihare, G. M. Santos, E. R. M. Druffel and A. Pearson, Proc. Natl. Acad. Sci. U. S. A., 2006, 103, 6442–6447 CrossRef CAS PubMed.
- S. M. Strycharz-Glaven, R. H. Glaven, Z. Wang, J. Zhou, G. J. Vora and L. M. Tender, Appl. Environ. Microbiol., 2013, 79, 3933–3942 CrossRef CAS PubMed.
- H.-T. Tran, D.-H. Kim, S.-J. Oh, K. Rasool, D.-H. Park, R.-H. Zhang and D.-H. Ahn, Water Sci. Technol., 2009, 59, 1803–1808 CAS.
- S. Freguia, S. Tsujimura and K. Kano, Electrochim. Acta, 2010, 55, 813–818 CrossRef CAS PubMed.
- L. T. Angenent and Z. He, Electroanalysis, 2006, 18, 2009–2015 CrossRef PubMed.
- B. Liang, H.-Y. Cheng, D.-Y. Kong, S.-H. Gao, F. Sun, D. Cui, F.-Y. Kong, A.-J. Zhou, W.-Z. Liu, N.-Q. Ren, W.-M. Wu, A.-J. Wang and D.-J. Lee, Environ. Sci. Technol., 2013, 47, 5353–5361 CrossRef CAS PubMed.
- D. Y. Sorokin, T. N. Zhilina, A. M. Lysenko, T. P. Tourova and E. M. Spiridonova, Extremophiles, 2006, 10, 213–220 CrossRef CAS PubMed.
Footnote |
† Electronic supplementary information (ESI) available: Fig. S1. Voltage generation of nitrifying MFC before open circuit experiment and after open circuit for 21 days. See DOI: 10.1039/c4ra03441d |
|
This journal is © The Royal Society of Chemistry 2014 |
Click here to see how this site uses Cookies. View our privacy policy here.