DOI:
10.1039/C4RA03226H
(Paper)
RSC Adv., 2014,
4, 23327-23337
Facile, high-yielding preparation of pyrrolidinium, piperidinium, morpholinium and 2,3-dihydro-1H-isoindolinium salts and ionic liquids from secondary amines†
Received
10th April 2014
, Accepted 6th May 2014
First published on 8th May 2014
Abstract
High yield and purity heterocyclic ionic liquids can be obtained via the microwave irradiation of equimolar amounts of a secondary amine and of an α,ω-dibromoalkane (i.e., 1,4-dibromobutane, 1,5-dibromopentane, bis(2-bromoethyl)ether, or α,α′-dibromo-o-xylene) in water, in the presence of potassium carbonate, followed by anion exchange with lithium bis(trifluoromethanesulfonyl)imide, affording pyrrolidinum, piperidinium and morpholinium ionic liquids, respectively. Using this methodology, a large number of homologous ionic liquids can be prepared from a small number of readily available and relatively cheap starting materials, including a new class of ionic liquids based on the 2,3-dihydro-1H-isoindolinium ring system. Analysis (using SWOT and the GSK “greenness” assessments) of this synthetic method and comparison with current literature preparations reveals that this new method delivers significant benefits across a range of relevant parameters.
Introduction
Generally, ionic liquids (ILs), i.e. molten salts with low melting points, consist of unsymmetrical organic cations (e.g., N,N′-dialkylimidazolium, N,N-dialkylpyrolidinium, N-alkylpyridinium, N,N-dialkylpiperidinium, tetraalkylammonium) paired with charge diffuse organic or inorganic anions (such as Cl−, Br−, PF6−, BF4−, (CF3SO2)2N−, CF3SO2−). ILs are characterized by negligible vapour pressure, non-flammability, high ionic conductivity, excellent solvating properties for a wide range of materials, high thermal and electrochemical stability, and a large liquidus range.1–4
In addition to their frequent use as solvents for synthesis and catalysis,5–7 there has been growing interest in ILs as components within a diverse range of applications. Examples of such applications include: electrolytes for use in electrochemistry,8 electrochemical devices (including batteries),9–14 double-layer capacitors,15,16 fuel cells,17–19 and photo-electrochemical cells;20–24 as media for the electrodeposition of metals and semiconductors;25 as heat stabilizers26 and anti-oxidants for lubricating oils;27–29 in gas storage30,31 and separations;32–35 and in separation science.36–38 One of the most established (albeit, until recently, unrecognised) industrial uses of ionic liquids has been in the production of ε-caprolactam.39
Of great value to the field of ionic liquids is the development of generic synthetic methods that are capable of producing a wide range of ILs from only a small pool of readily available, inexpensive and easily purified precursors. An increasingly common example of such a generic method is the preparation of chiral ionic liquids from the chiral pool, using readily accessible acid–base chemistry.40,41 To date, most frequently used ILs are prepared by quaternisation of the parent ring system. However, such simple heterocyclic systems are often expensive and difficult to purify due to their high boiling points. Additionally, significant time and effort must be expended purifying the quaternised intermediate prior to anion exchange.42 As a contribution towards the goal of devising a set of generic, straightforward and rapid methods for facile IL synthesis, we describe the high yielding preparations of a range of ionic liquids based on pyrrolidinium, piperidinium, oxazolium, and 2,3-dihydro-1H-isoindolinium that uses readily available and cheap secondary amines and α,ω-dibromoalkanes significantly extending the method described earlier by Ju and Varma.43
Results and discussion
The creation of a generic method for the preparation of a large range of new and existing ionic liquids from a small pool of cheap and readily available chemicals, using energy efficient methods and minimal purification is an area of increasing importance given the great interest in the applications of ILs. Since the most widely used ionic liquids (with the exception of imidazolium ILs) consist of a quaternised nitrogenous ring systems, the most logical starting point is the derivatisation of secondary amines. Using K2CO3 in water to deprotonate the amine in the presence of an α,ω-dibomoalkane at 120 °C results in the formation of the desired quaternised ring system. The ILs can be isolated easily in high yield through judicious choice of a suitable anion. Throughout this paper the bis(trifluoromethanesulfonyl)imide anion has been used, as its presence results in hydrophobic ILs, which can be readily separated from water and washed to remove any inorganic contaminants. Thus, using this method we have prepared 36 ILs or salts (of a total possible 44) in four homologous series using 15 different starting materials.
It should be noted that we have found this reaction to only be significantly accelerated when using a focused or monomodal microwave system (with microwave power up to 850 W). Attempts to perform this reaction in a standard multimodal laboratory microwave system (with microwave power up to 1000 W) did not result in the isolation of any of the desired products after irradiation times of up to 60 min, indicating that substantially longer reaction times are necessary to achieve the desired products. In both cases, the microwave reactors were operated in a manner whereby only the temperature of the reaction mixture was set (i.e., the microwave power was not fixed for a continuous irradiation experiment). Consequently, it is not believed that the power of the irradiation is a critical parameter. In focused microwave systems the radiation is directed through an accurately designed wave guide into the reaction vessel mounted at a fixed distance from the radiation source, thereby creating a standing wave or single mode.44 The greater reaction rates in the monomodal microwave presumably arise as a result of the more uniform heating pattern and the higher microwave field strengths that can be obtained.45
Once the set irradiation time was completed (and in those cases where the bromide salt was water-soluble), the homogeneous aqueous solution was extracted with diethyl ether to remove any unreacted organic precursors. Anion exchange was then performed by addition of one equivalent of lithium bis(trifluoromethanesulfonyl)imide, which resulted in the desired salt separating from the aqueous phase. Subsequently, additional salt was extracted from the aqueous phase using CH2Cl2 and the combined organic phases washed with water to remove excess NTf2− anion and other salt contaminates present. Removal of the solvent and drying at 60 °C in vacuo afforded the desired products with no further purification necessary.
Irradiation of the equimolar mixture of secondary amine and either 1,4-dibromobutane or 1,5-dibromopentane in the presence of aqueous K2CO3 at 120 °C followed by anion exchange resulted in the isolation of the desired pyrrolidinium and piperidinium salts (Scheme 1) with yields usually greater than 80% (Table 1). In general, 20 min and 40 min irradiation times, respectively, were required when 1,4-dibromobutane and 1,5-dibromopentane were used. The longer reaction time when using the 1,5-dibromopentane was required due to its lower reactivity as a consequence of the longer alkyl chain length. When sterically hindered secondary amines were used, such as di-sec-butylamine (entries 5 and 6) or di-iso-propylamine (entries 9 and 10), the yields drop dramatically to 10–24%, even after prolonged irradiation times. When dioctylamine was used, the resultant bromide salt was immiscible in water and consequently no effective anion exchange was possible (entries 20 and 21) using the standard anion metathesis protocol. Attempts to use 1,6-dibromohexane to form the corresponding N,N-dialkylhexahydroazepinium salts were not successful, despite the formation of the 7-membered ring being favourable (by the 7-exo-tet mechanism) according to Baldwin's rules46 and the fact that such rings were formed when using primary amines under the same methodology.43
 |
| Scheme 1 | |
Table 1 Syntheses and properties of pyrrolidinium and piperidinium NTf2 salts prepared from secondary amines and α,ω-dihaloalkanes at 120 °C
|
R1, R2 |
Dihalo compound |
Reaction time (min) |
Yield (%)a |
m.p. (°C) |
Tdec (°C) |
Isolated yield. Isolated as the bromide salt. Glass transition. |
1 |
R1 = R2 = CH2C6H5 |
Br(CH2)4Br |
20 |
84 |
69–71 |
328 |
2 |
R1 = R2 = CH2C6H5 |
Br(CH2)5Br |
40 |
81 |
107–109 |
331 |
3 |
R1 = R2 = C4H9 |
Br(CH2)4Br |
20 |
88 |
38–40 |
367 |
4 |
R1 = R2 = C4H9 |
Br(CH2)5Br |
40 |
88 |
57–59 |
355 |
5 |
R1 = R2 = CH(CH3)C2H5 |
Br(CH2)4Br |
60 |
24 |
−72c |
280 |
6 |
R1 = R2 = CH(CH3)C2H5 |
Br(CH2)5Br |
80 |
10 |
−73c |
232 |
7 |
R1 = R2 = C3H7 |
Br(CH2)4Br |
20 |
83 |
50–52 |
393 |
8 |
R1 = R2 = C3H7 |
Br(CH2)5Br |
40 |
82 |
65–67 |
344 |
9 |
R1 = R2 = CH(CH3)2 |
Br(CH2)4Br |
40 |
23 |
58–60 |
305 |
10 |
R1 = R2 = CH(CH3)2 |
Br(CH2)5Br |
60 |
23 |
90–92 |
246 |
11 |
R1 = R2 = C2H5 |
Br(CH2)4Br |
20 |
85 |
92–94 |
317 |
12 |
R1 = R2 = C2H5 |
Br(CH2)5Br |
40 |
88 |
92–94 |
370 |
13 |
R1 = C4H9; R2 = C2H5 |
Br(CH2)4Br |
20 |
86 |
−87c |
340 |
14 |
R1 = C4H9; R2 = C2H5 |
Br(CH2)5Br |
40 |
86 |
−75c |
360 |
15 |
R1 = C4H9; R2 = CH3 |
Br(CH2)4Br |
20 |
83 |
−15 (−83c) |
348 |
16 |
R1 = C4H9; R2 = CH3 |
Br(CH2)5Br |
40 |
87 |
−72c |
331 |
17 |
R = –(CH2)4– |
Br(CH2)4Br |
20 |
90 |
113–115 |
322 |
18 |
R = –(CH2)5– |
Br(CH2)4Br |
20 |
88 |
110–112 |
330 |
19 |
R = –(CH2)5– |
Br(CH2)5Br |
40 |
89 |
127–129 |
316 |
20 |
R1 = R2 = C8H17b |
Br(CH2)4Br |
20 |
94 |
126–127 |
207 |
21 |
R1 = R2 = C8H17b |
Br(CH2)5Br |
40 |
94 |
149–151 |
202 |
The decomposition point of the salts was determined by thermogravimetric analysis (TGA) and the data are shown in Table 1. The NTf2 salts show remarkable thermal stability, with the majority of the salts decomposing at temperatures greater than 300 °C. In general, the pyrrolidinium NTf2 salts have higher thermal stability as compared to the equivalent piperidinium salts: an exception being found in the case of the N,N-diethyl-substituted salts and the N-butyl-N-ethyl-substituted derivatives for which the piperidinium salt has a higher decomposition point than the corresponding pyrrolidinium salt. The pyrrolidinium NTf2 salts have decomposition points ranging from 322–393 °C, whilst the piperidinium salts have decomposition points ranging from 316–370 °C. Salts containing sec-butyl and iso-propyl groups have significantly lowered decomposition points with all such compounds showing decomposition below 305 °C.
With the success of this procedure when using the α,ω-dihaloalkanes, the method was expanded to the use of bis(2-bromoethyl)ether as the precursor for the backbone of the ring system to form the corresponding morpholinium salts (Scheme 2). As was the case with the 1,5-dibromopentane precursor, an increase in reaction time from 20 to 40 min was required to achieve a yield of more than 75%. Nevertheless, a small decrease in the isolated yields of the products to 78–87% (Table 2) could not be avoided. As was the case with 1,5-dibromopentane, the decrease in yield appears to be due to the increase in the chain length of the dihalogenated precursor. When pyrrolidine and piperidine were used as the secondary amines, a significant decrease in yield (to ∼50%) was observed as compared to the dialkyl secondary amines after 40 min of irradiation. However, increasing the reaction time to 60 min resulted in yields of only ∼65%.
 |
| Scheme 2 | |
Table 2 Syntheses and properties of N,N-dialkylmorpholinium NTf2 ILs prepared from secondary amines and bis(2-bromoethyl)ether at 120 °C
|
R1, R2 |
Dihalo compound |
Reaction time (min) |
Yield (%)a |
m.p. (°C) |
Tdec (°C) |
Isolated yield. Glass transition point. |
22 |
R1 = R2 = CH2C6H5 |
{Br(CH2)2}2O |
40 |
78 |
149–151 |
265 |
23 |
R1 = R2 = C4H9 |
{Br(CH2)2}2O |
40 |
87 |
−48b |
327 |
24 |
R1 = R2 = C3H7 |
{Br(CH2)2}2O |
40 |
87 |
50–52 |
301 |
25 |
R1 = R2 = C2H5 |
{Br(CH2)2}2O |
40 |
85 |
39–41 |
322 |
26 |
R1 = C4H9; R2 = CH3 |
{Br(CH2)2}2O |
40 |
82 |
29–31 |
294 |
27 |
R = –(CH2)4– |
{Br(CH2)2}2O |
60 |
66 |
73–75 |
369 |
28 |
R = –(CH2)5– |
{Br(CH2)2}2O |
60 |
64 |
119–121 |
359 |
A new class of ionic liquids based on the 2,3-dihydro-1H-isoindolinium ring system could also be readily prepared in high yields using this method. Substitution of the α,ω-dibromoalkane with α,α′-dibromo-o-xylene resulted in the formation of the dihydro-1H-isoindolinium ring system in yields ranging from 78–96% (Scheme 3, Table 3). In the case of the dibenzyl analogue (29) the irradiation time had to be increased to 60 min in order to achieve a 75% yield of the bromide salt. The longer reaction time needed and lower yield obtained when compared to the equivalent piperidinium and pyrrolidinium salts may be attributed to the steric bulk of the amine and the α,α′-dibromo-o-xylene. In this case, the N,N-dibenzyl-2,3-dihydro-1H-isoindolinium bromide obtained was insoluble in water, hence, no anion exchange with LiNTf2 was possible using the standard anion metathesis protocol outlined (Experimental section), and thus was not attempted. The products obtained after anion exchange were liquids at room temperature (with the exception of the diethyl and the spiro salts) with melting points in the range from −43 to −54 °C and decomposition temperatures >340 °C. In the case of the N,N-diethyl-2,3-dihydro-1H-isoindolinium bis(triflouro-methanesulfonyl)imide IL, crystallisation occurred only after a prolonged period at room temperature. N,N-Dibenzyl-2,3-dihydro-1H-isoindolinium bromide had a decomposition point of 175 °C.
 |
| Scheme 3 | |
Table 3 Syntheses and properties of N,N-dialkyl-2,3-dihydro-1H-isoindolinium NTf2 ILs prepared from secondary amines and α,α′-dibromo-o-xylene at 120 °C
|
R1, R2 |
Dihalo compound |
Reaction time (min) |
Yield (%)a |
m.p. (°C) |
Tdec (°C) |
Isolated yield. Isolated as the bromide salt. Decomposes before melting. Determined by differential scanning calorimetry. Glass transition. |
29 |
R1 = R2 = CH2C6H5b |
o-(BrCH2)2C6H4 |
60 |
75 |
—c |
175 |
30 |
R1 = R2 = C4H9 |
o-(BrCH2)2C6H4 |
60 |
84 |
−44d |
340 |
31 |
R1 = R2 = C3H7 |
o-(BrCH2)2C6H4 |
60 |
95 |
−43d |
365 |
32 |
R1 = R2 = C2H5 |
o-(BrCH2)2C6H4 |
60 |
84 |
81–83 |
354 |
33 |
R1 = C4H9; R2 = CH3 |
o-(BrCH2)2C6H4 |
60 |
92 |
−54d (−17e) |
363 |
34 |
R1 = C4H9; R2 = C2H5 |
o-(BrCH2)2C6H4 |
60 |
83 |
−54d (−10e) |
347 |
35 |
R = –(CH2)4– |
o-(BrCH2)2C6H4 |
60 |
91 |
74–76 |
391 |
In order to determine the effectiveness of the microwave synthesis, the reaction replicating the preparation of N,N-dibutylpiperidinium NTf2 (entry 4, Table 1) was undertaken using conventional heating at 120 °C. This was performed using a sealed stainless steel autoclave with a Teflon liner. After 16 h, the reaction was worked up as previously to obtain the desired product in 80% yield. This is slightly lower than the 88% yield obtained for the identical reaction using 40 min irradiation. Additionally, the effectiveness of this synthetic methodology is highlighted by comparison to the previously reported syntheses for the azoniaspiro salts (entries 17–19 in Table 1; entries 27–28 in Table 2).47–49 The original synthetic methodology involves the addition of either pyrrolidine, piperidine, or oxazoline to a boiling solution of the appropriate α,ω-dibromoalkane in aqueous NaOH for 12–24 h. After the work-up, yields of the desired bromide salts were in the range of 19–68%. In this work, yields of the corresponding NTf2 salts ranged from ∼65% (for the morpholinium salts) and 80–89% (for the pyrrolidinium and piperidinium salts). Even if the conversion of the bromide salts to their NTf2 analogues was quantitative, the present synthetic method is clearly more efficient. As a consequence, it can be concluded that the focussed microwave irradiation used herein has a dramatic effect on the acceleration of this reaction compared to multi-modal microwave irradiation and conventional heating.
To highlight the increased sustainability of this synthetic method a comparison to existing methods to prepare pyrrolidinium or morpholinium ILs was undertaken. To fairly compare the chosen syntheses, the sequential functionalisation of pyrrolidine, piperidine, and morpholine to form the corresponding ILs was chosen (Scheme 4). Recent work in this area has shown the reductive alkylation of these cyclic amines can be achieved in good yields using either zinc powder50 or NaBH4 (ref. 51) and a suitable aldehyde in 1.5 to 5 molar equivalents with respect to the starting amine. The former method reports only methylations using formaldehyde in water, whilst the latter uses a variety of different aldehydes (and paraformaldehyde for the methylation reaction) in trifluoroethanol (TFE). For the methylation reaction, the yield of the resultant methyl-substituted compounds is 82–98% after 2–4 h at temperatures between RT and 78 °C. Isolation of the products was performed by filtration, followed by washing of the residue, distillation of the solvent for recycling (in the case of TFE) and then chromatography if required. Subsequent alkylation of the thus obtained methyl substituted amine with bromobutane (for example) at 40 °C followed by anion exchange with LiNTf2 in water affords the desired IL.42 The removal of coloured impurities can be achieved by heating an aqueous solution of the intermediate bromide salt at 65 °C for 24 h in the presence of activated charcoal.42 Ultimately, after the 3 steps the final yield of the desired IL is in the range of 73–87% (cf. this work achieves yields of 82–92% for the identical ILs). However, significantly greater molar amounts of starting materials were required, substantially longer heating times needed, and a greater number of purification steps required, as compared to our method described here.
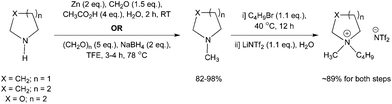 |
| Scheme 4 | |
A metric to test the sustainability and “greenness” of the current process to that shown in Scheme 4 was recently published by GSK.52 The method of determining the overall “greenness” of a process involves a standardized assessment of each reagent used within a chemical process: the calculation of an EHS (Environmental, Health, Safety) score is done by considering the presence or absence of certain risk phrases associated with each chemical followed by the geometric mean of the three scores allocated; the calculation of an overall clean chemistry score by taking the geometric mean of scores allocated to assessing aspects of the process such as ease of work-up, use of co-reagents, stoichiometry, atom efficiency and other issues (such as decomposition, the need for low temperatures, the use of inert atmospheres, etc.). Finally, the Overall “Greenness” score is determined by taking the geometric mean of the EHS and overall clean chemistry scores: thus, for the synthesis of 1-butyl-1-methylpyrrolidinium bis(trifluoromethanesulfon)-imide (15), the Overall “Greenness” score for the method described herein is 7.5 whilst when using the methodology outlined in Scheme 4 the score is 4.2 or 4.5 (for both methods in step 1). Note that these scores have been calculated without including the reaction or extraction solvents as this method only takes into account the reagents used. The use of different substrates to make the different ring systems has little effect on the overall “greenness” of either process (see ESI† for the calculations).
In attempting to increase the “greenness” of ionic liquid syntheses many variables need to be considered in order to improve the process. Analysis of the synthesis of 1-alkyl-3-methylimidazolium ionic liquids suggests the use of the strategic planning SWOT analysis to assess the greenness of any synthesis method.53 Utilising such an analysis for the syntheses outlined herein (Scheme 1) reveals that the process employed has a low E-factor, high atom economy (as only equimolar amounts of the starting materials are used) and upholds seven of the twelve Principles of Green Chemistry54 (prevention of waste; high atom economy; the elimination of the use of unnecessary auxiliary substances; low energy requirements; elimination of unnecessary derivatisation; the use of analytical methodologies to prevent formation of hazardous substances; and, the use of a chemical process to minimize the potential for chemical accidents). It should be noted that the E-factor could be lowered even further in those cases where room temperature ionic liquids are formed, as these could be effectively washed with water to remove the salt by-products without the use of CH2Cl2. Further benefits are also derived by the use of water as the solvent as this overcomes many of the issues associated with the use of microwave heating (i.e. mass transport issues of starting materials due to viscosity of the ionic liquid as the intermediate halide salts are highly soluble; and, there is no IL decomposition due to the presence of hot-spots). In all of the cases mentioned here, the final products were obtained as colourless to pale yellow products that were judged to be pure according to NMR spectroscopy (and melting points/glass transition points/decomposition points of the known ILs prepared were very close to the reported literature values) and hence required no additional purification steps.
Conclusions
Rapid, high-yielding syntheses of a range of ionic liquids from readily available (and inexpensive) secondary amines and a variety of α,ω-dihaloalkanes have been introduced, including a route to a new class of ionic liquids based on the 2,3-dihydro-1H-isoindolinium ring system. Coupled with the easy work-up this represents an excellent generic synthetic method to four families of heterocyclic ILs. The application of different metrics (the GSK reagent guide and SWOT) indicates that the new synthetic procedure is significantly greener than the corresponding synthesis using existing procedures.
Experimental
The following chemicals were used without further purification: 1,4-dibromobutane, piperidine, lithium bis(trifluoromethanesulfonyl)imide, bis(2-bromoethyl)ether (all Alfa Aesar), 1,5-dibromopentane (Fluka), pyrrolidine (BDH), α,α′-dibromo-o-xylene (Aldrich) and potassium carbonate (Merck). The amines N-butyl-N-ethylamine, N-butyl-N-methylamine (both Aldrich), dibenzylamine, dipropylamine, di-iso-propylamine, diethylamine (all Merck), dibutylamine, and di-sec-butylamine (all BDH) were passed through a plug of activated basic alumina to remove any coloured impurities prior to use.
All irradiations were performed using a Biotage Initiator 2.5 focused microwave system or an Anton Paar Monowave 300 focused microwave system. All reactions were performed in a 20 mL glass reaction vessel with a crimped cap (Biotage) or a 30 mL glass vessel with a snap cap (Anton Paar) with a magnetic stirrer bar.
1H (300.13 MHz), 13C{1H} (75.4 MHz), and 19F (282.38 MHz) NMR spectra were recorded on a Bruker DPX300 spectrometer. 1H and 13C{1H} NMR spectra were referenced internally to the proteo impurity of the solvent (CDCl3, δ 7.26 ppm (1H) or δ 77.16 ppm (13C)). 19F NMR spectra were referenced externally to TMS. Infrared spectra were recorded using a Shimadzu FTIR-8000S spectrophotometer. Samples were recorded as thin films on NaCl plates. Spectra were recorded using 16 scans with a resolution of 4 cm−1 over a range of 4000–400 cm−1. Electrospray ionisation (ESI) mass spectroscopy was performed using a Finnigan LCQ mass spectrometer.
Melting points were determined in air using a Gallenkamp melting point apparatus and are uncorrected. Thermogravimetric analyses (TGA) of all compounds were performed using a TA Instruments Hi-Res TGA 2950 thermogravimetric analyzer. Samples (5–15 mg) were analysed on a platinum pan with a ramp rate of 5 °C min−1 to a temperature of 500 °C in a N2/O2 atmosphere. Data were analysed using the TA Universal Analysis program. The decomposition temperature (Tdec) was the intersection of the tangents of the thermogravimetry curve at the horizontal baseline and the steepest part of the curve.55 Differential scanning calorimetry (DSC) was performed using a TA Instruments Modulated 2920 DSC. Samples (5–10 mg) were loaded into an aluminum pan and were cooled at 10 °C min−1 to −140 °C, equilibrated for 10 min then warmed at 10 °C min−1 to 25 °C. The samples were cycled through this temperature program twice to ensure the reproducibility of the data. Data were analysed using the TA Universal Analysis program.
Standard synthetic procedure
In a typical reaction, a mixture of the dialkylamine (11.12 mmol), α,ω-dibromoalkane (11.12 mmol), potassium carbonate (1.54 g, 11.12 mmol) and water (15 mL) was irradiated at 120 °C for 20 min (for 1,4-dibromobutane), 40 min (for 1,5-dibromopentane and bis(2-bromoethyl)ether), or 60 min (for α,α′-dibromo-o-xylene). The resultant reaction mixture was washed with diethyl ether (2 × 10 mL). To the aqueous phase was added lithium bis(trifluoromethanesulfonyl)imide (0.800 g, 2.79 mmol) and the resultant solution was stirred for 1 h at room temperature. The reaction mixture was extracted with dichloromethane (3 × 20 mL) and the combined organic phases were washed with water (2 × 10 mL) and separated. The solvent of the combined organic phases was removed in vacuo to afford the desired product. The product was dried at 60 °C in vacuo overnight to remove all traces of water.
Procedure for conventional heating
To a sealed stainless steel autoclave was added diethylamine (1.44 g, 11.12 mmol), 1,5-dibromopentane (2.56 g, 11.12 mmol), potassium carbonate (1.54 g, 11.12 mmol) and water (15 mL). The autoclave was sealed and heated at 120 °C in an oil bath for 16 h. Upon termination of the heating, the autoclave was cooled to room temperature and the reaction worked up according to the method described above. Yield 4.26 g (80%). See below for characterisation.
N,N-Dibenzylpyrrolidinium bis(trifluoromethanesulfonyl)imide
White powder. Yield: 4.97 g (84%). m.p. 69–71 °C. Tdec 328 °C. 1H NMR (CDCl3): δ 7.38 (m, 10H, ArH), 4.39 (s, 4H, ArCH2), 3.42 (m, 4H, NCH2 ring), 1.93 (m, 4H, NCH2CH2 ring) ppm. 13C{1H} NMR (CDCl3): δ 133.04, 131.15, 129.71, 127.12, 120.05 (q, 1JCF = 321 Hz, CF3), 64.70, 58.39, 21.41 ppm. 19F NMR (CDCl3): δ −79.19 (s) ppm. m/z (ESI+): 252 (M+, 100%), 783 ([2M + NTf2]+, 11). IR (thin film, NaCl): ν 3065(m), 3036(m), 3013(m), 2984(m), 1497(s), 1456(vs), 1323(vs), 1267(s), 1180(vs), 1168(vs), 1130(vs), 1060(vs), 910(m), 891(m), 788(s) cm−1.
N,N-Dibenzylpiperidinium bis(trifluoromethanesulfonyl)imide
White powder. Yield: 4.92 g (81%). m.p. 107–109 °C. Tdec 331 °C. 1H NMR (CDCl3): δ 7.36 (m, 10H, ArH), 4.37 (s, 4H, ArCH2), 3.17 (m, 4H, NCH2 ring), 1.97 (m, 4H, NCH2CH2 ring), 1.45 (m, 2H, NCH2CH2CH2 ring) ppm. 13C{1H} NMR (CDCl3): δ 133.22, 131.05, 129.57, 126.22, 120.0 (q, 1JCF = 321 Hz, CF3), 64.10, 56.02, 20.65, 19.79 ppm. 19F NMR (CDCl3): δ −79.19 (s) ppm. m/z (ESI+): 266 (M+, 100%), 811 ([2M + NTf2]+, 11). IR (thin film, NaCl): ν 3069(m), 3041(m), 2970(m), 2947(s), 2876(m), 1475(m), 1454(s), 1394(m), 1350(vs), 1327(vs), 1265(s), 1188(vs), 1139(s), 1056(vs), 972(m), 790(m) cm−1.
N,N-Dibutylpyrrolidinium bis(trifluoromethanesulfonyl)imide
White powder. Yield: 4.53 g (88%). m.p. 38–40 °C. Tdec 367 °C. 1H NMR (CDCl3): δ 3.30 (t, 4H, 3JHH = 6 Hz, NCH2), 3.00 (4H, NCH2 ring), 1.99 (m, 4H, NCH2CH2 ring), 1.46 (M, 4H, NCH2CH2), 1.19 (m, CH2CH3), 0.78 (t, 6H, 3JHH = 7 Hz, CH3) ppm. 13C{1H} NMR (CDCl3): δ 119.63 (q, 1JCF = 321 Hz, CF3), 62.63, 59.55, 24.75, 21.39, 19.13, 12.87 ppm. 19F NMR (CDCl3): δ −79.79(s) ppm. m/z (ESI+): 184 (M+, 100%), 648 ([2M + NTf2]+, 11). IR (thin film, NaCl): ν 2968(s), 2941(s), 2880(m), 1466(s), 1350(vs), 1333(vs), 1225(vs), 1194(vs), 1138(vs), 1055(vs), 908(m), 788(m) cm−1.
N,N-Dibutylpiperidinium bis(trifluoromethanesulfonyl)imide
White powder. Yield: 4.66 g (88%). m.p. 57–59 °C. Tdec 355 °C. 1H NMR (CDCl3): δ 3.22 (t, 4H, 3JHH = 6 Hz, NCH2), 3.10 (m, 4H, NCH2 ring), 1.75 (m, 4H, NCH2CH2 ring), 1.56 (m, 2H, NCH2CH2CH2 ring), 1.49 (m, 4H, NCH2CH2), 1.29 (m, CH2CH3), 0.88 (t, 6H, 3JHH = 7 Hz, CH3) ppm. 13C{1H} NMR (CDCl3): δ 119.89 (q, 1JCF = 321 Hz, CF3), 59.41, 58.54, 23.25, 20.71, 19.53, 19.48, 13.28 ppm. 19F NMR (CDCl3): δ −79.53(s) ppm. m/z (ESI+): 198 (M+, 100%), 676 ([2M + NTf2]+, 20). IR (thin film, NaCl): ν 2966(s), 2880(m), 1479(s), 1462(s), 1454(s), 1354(vs), 1333(vs), 1227(vs), 1138(vs), 1057(vs), 1036(s), 931(m), 788(m) cm−1.
N,N-Di-sec-butylpyrrolidinium bis(trifluoromethanesulfonyl)imide
Yellow oil. Yield: 1.23 g (24%). Tg −72 °C. Tdec 280 °C. 1H NMR (CDCl3): δ 3.54 (bm, 1H, N–CH ring), 3.40 (m, 2H, NCH), 3.22 (m, 3H, NCH2 ring and NCH ring), 2.06 (m, 2H, NCH2CH2 ring), 1.85 (bm, 1H, NCH2CH ring), 1.77 (m, 2H, CHCH2), 1.53 (m, 2H, CHCH2), 1.46 (bm, 1H, NCH2CH ring), 1.30 (d, 3H, 3JHH = 7 Hz, CHCH3), 1.28 (d, 3H, 3JHH = 7 Hz, CHCH3), 0.96 (t, 3H, 3JHH = 7 Hz, CHCH3), 0.94 (t, 3H, 3JHH = 7 Hz, CHCH3) ppm. 13C{1H} NMR (CDCl3): δ 119.68 (q, 1JCF = 321 Hz, CF3), 70.37, 70.25, 54.39, 54.35, 26.31, 26.02, 23.68, 23.57, 15.59, 14.92, 9.56, 9.47 ppm. 19F NMR (CDCl3): δ −79.43(s) ppm. m/z (ESI+): 184 (M+, 100%), 648 ([2M + Br]+, 34). IR (thin film, NaCl): ν 3171(s), 3117(m), 2982(s), 2947(m), 2887(m), 1468(m), 1398(m), 1346(vs), 1327(vs), 1203(vs), 1136(vs), 1059(vs), 791(m) cm−1.
N,N-Di-sec-butylpiperidinium bis(trifluoromethanesulfonyl)imide
Pale yellow oil. Yield: 0.53 g (10%). Tg −73 °C. Tdec 232 °C. 1H NMR (CDCl3): δ 3.45 (m, 1H, NCH), 3.29 (m, 2H, NCH2 ring), 3.14 (m, 2H, NCH2 ring), 1.95 (m, 1H, NCH), 1.65 (m, 4H, NCH2CH2 ring), 1.58 (m, 4H, NCH2CH2 ring), 1.48 (m, 4H, CHCH2), 1.33 (d, 3H, 3JHH = 7 Hz, CHCH3), 1.22 (d, 3H, 3JHH = 7 Hz, CHCH3), 0.93 (t, 3H, 3JHH = 7 Hz, CH2CH3), 0.87 (t, 3H, 3JHH = 7 Hz, CH2CH3) ppm. 13C{1H} NMR (CDCl3): δ 119.81 (q, 1JCF = 321 Hz, CF3), 67.81, 54.41, 54.36, 26.37, 25.70, 24.40, 24.38, 15.74, 14.47, 14.40, 9.71, 9.63 ppm. 19F NMR (CDCl3): δ −79.43(s) ppm. m/z (ESI+): 198 (M+, 100%), 676 ([2M + Br]+, 24). IR (thin film, NaCl): ν 3169(m), 3123(m), 2982(m), 2949(m), 2887(m), 1460(m), 1398(m), 1350(vs), 1329(s), 1227(s), 1196(vs), 1136(s), 1059(s), 791(m) cm−1.
N,N-Dipropylpyrrolidinium bis(trifluoromethanesulfonyl)imide
White powder. Yield: 4.04 g (83%). m.p. 50–52 °C. Tdec 393 °C. 1H NMR (CDCl3): δ 3.52 (t, 4H, 3JHH = 7 Hz, NCH2), 3.17 (m, 4H, NCH2 ring), 2.21 (m, 4H, NCH2CH2 ring), 1.72 (m, 4H, NCH2CH2), 1.03 (t, 6H, 3JHH = 7 Hz, CH3) ppm. 13C{1H} NMR (CDCl3): δ 120.27 (q, 1JCF = 321 Hz, CF3), 63.40, 61.97, 22.19, 17.23, 10.68 ppm. 19F NMR (CDCl3): δ −79.36(s) ppm. m/z (ESI+): 156 (M+, 100%), 592 ([2M + NTf2]+, 17). IR (thin film, NaCl): ν 3061(w), 2978(s), 2945(m), 2887(m), 1462(s), 1350(vs), 1333(vs), 1269(m), 1180(vs), 1138(vs), 1053(vs), 788(s) cm−1.
N,N-Dipropylpiperidinium bis(trifluoromethanesulfonyl)imide
White powder. Yield: 4.13 g (82%). m.p. 65–67 °C. Tdec 344 °C. 1H NMR (CDCl3): δ 3.32 (t, 4H, 3JHH = 7 Hz, NCH2), 3.16 (m, 4H, NCH2 ring), 1.86 (m, 4H, CH2 ring), 1.69 (m, 6H, CH2 ring and NCH2CH2), 1.00 (t, 6H, 3JHH = 7 Hz, CH3) ppm. 13C{1H} NMR (CDCl3): δ 120.04 (q, 1JCF = 321 Hz, CF3), 60.40, 59.59, 20.88, 19.69, 15.12, 10.57 ppm. 19F NMR (CDCl3): δ −79.43(s) ppm. m/z (ESI+): 170 (M+, 100%), 620 ([2M + NTf2]+, 77). IR (thin film, NaCl): ν 3064(w), 2978(s), 2968(s), 2887(m), 1484(s), 1463(s), 1351(vs), 1268(s), 1197(vs), 1138(vs), 1055(vs), 790(m) cm−1.
N,N-Di-iso-propylpyrrolidinium bis(trifluoromethanesulfonyl)imide
White powder. Yield: 1.10 g (23%). m.p. 58–60 °C. Tdec 305 °C. 1H NMR (CDCl3): δ 3.93 (sept, 3JHH = 7 Hz, CHCH3), 3.45 (m, 4H, NCH2 ring), 2.11 (m, 4H, CH2 ring), 1.39 (t, 3JHH = 7 Hz, 12H, CH3) ppm. 13C{1H} NMR (CDCl3): δ 119.82 (q, 1JCF = 321 Hz, CF3), 64.79, 56.40, 26.02, 17.44 ppm. 19F NMR (CDCl3): δ −78.81 (s) ppm. m/z (ESI+): 156 (M+, 100%), 592 ([2M + NTf2]+, 17). IR (thin film, NaCl): ν 3062(w), 2999(m), 2986(m), 2894(w), 1491(m), 1470(m), 1409(m), 1392(m), 1353(vs), 1268(s), 1195(vs), 1138(vs), 1102(m), 1058(vs), 1003(w), 924(w), 789(m), 740(vs) cm−1.
N,N-Di-iso-propylpiperidinium bis(trifluoromethanesulfonyl)imide
White powder. Yield: 1.14 g (23%). m.p. 90–92 °C. Tdec 246 °C. 1H NMR (CDCl3): δ 3.89 (sept, 3JHH = 7 Hz, CHCH3), 3.22 (m, 4H, N–CH2 ring), 1.78 (m, 4H, N–CH2CH2 ring), 1.612 (m, 2H, N–CH2CH2CH2 ring), 1.35 (d, 12H, 3JHH = 7 Hz, CHCH3) ppm. 13C{1H} NMR (CDCl3): δ 119.71 (q, 1JCF = 321 Hz, CF3), 60.66, 53.70, 20.52, 19.48, 17.51 ppm. 19F NMR (CDCl3): δ −79.05(s) ppm. m/z (ESI+): 170 (M+, 100%), 620 ([2M + NTf2]+, 17). IR (thin film, NaCl): ν 2958(w), 3001(w), 2889(w), 2849(w), 1467(m), 1407(m), 1349(vs), 1333(s), 1197(vs), 1140(s), 1051(s), 935(w), 903(w), 863(w), 793(m), 740(m) cm−1.
N,N-Diethylpyrrolidinium bis(trifluoromethanesulfonyl)imide
White powder. Yield: 3.87 g (85%). m.p. 92–94 °C. Tdec 317 °C. 1H NMR (CDCl3): δ 3.41 (m, 4H, NCH2 ring), 3.27 (q, 3JHH = 7 Hz, NCH2), 2.15 (m, 4H, OCH2 ring), 1.29 (t, 3JHH = 7 Hz, CH3) ppm. 13C{1H} NMR (CDCl3): δ 119.87 (q, 1JCF = 321 Hz, CF3), 62.11, 54.66, 21.74, 8.46 ppm. 19F NMR (CDCl3): δ −79.22(s) ppm. m/z (ESI+): 128 (M+, 100%), 536 ([2M + NTf2]+, 17). IR (thin film, NaCl): ν 2995(m), 2952(w), 2900(w), 1466(m), 1408(m), 1352(vs), 1330(s), 1198(vs), 1141(s), 1056(vs), 790(m), 740(m) cm−1.
N,N-Diethylpiperidinium bis(trifluoromethanesulfonyl)imide
White powder. Yield: 4.13 g (88%). m.p. 99–100 °C. Tdec 370 °C. 1H NMR (CDCl3): δ 3.19 (q, 4H, 3JHH = 7 Hz, CH2N), 3.14 (m, 4H, CH2N ring), 1.73 (m, 4H, NCH2CH2 ring), 1.56 (m, 2H, NCH2CH2CH2 ring), 1.15 (t, 6H, 3JHH = 7 Hz, CH3) ppm. 13C{1H} NMR (CDCl3): δ −119.67 (q, 1JCF = 321 Hz, CF3), 58.25, 53.11, 20.54, 19.15, 6.50 ppm. 19F NMR (CDCl3): δ −79.65(s) ppm. m/z (ESI+): 142 (M+, 100%), 564 ([2M + NTf2]+, 26). IR (thin film, NaCl): ν 2995(w), 2958(m), 2883(w), 1484(m), 1464(m), 1405(m), 1352(vs), 1333(s), 1198(vs), 1139(s), 1054(s), 944(m), 790(m), 739(m) cm−1.
N-Butyl-N-ethylpyrrolidinium bis(trifluoromethanesulfonyl)imide
White powder. Yield: 4.18 g (86%). Tg −87 °C (lit. −84 °C (ref. 56)). Tdec 340 °C. 1H NMR (CDCl3): δ 3.34 (m, 4H, NCH2 and NCH2 ring), 3.16 (q, 2H, 3JHH = 7 Hz, CH2CH3), 3.06 (m, 2H, NCH2 ring), 2.06 (m, 4H, NCH2CH2 ring), 1.54 (m, 2H, NCH2CH2), 1.27 (m, 2H, CH2CH3), 1.20 (t, 3H, 3JHH = 7 Hz, NCH2CH3) ppm. 13C{1H} NMR (CDCl3): δ 119.64 (q, 1JCF = 321 Hz, CF3), 62.31, 59.18, 54.87, 24.75, 21.45, 19.21, 12.94, 8.21 ppm. 19F NMR (CDCl3): δ −79.73(s) ppm. m/z (ESI+): 156 (M+, 100%), 592 ([2M + Br]+, 46). IR (thin film, NaCl): ν 3184(w), 2968(m), 2943(m), 2880(m), 1466(m), 1346(vs), 1331(vs), 1180(vs), 1138(vs), 1057(vs), 910(w), 788(m) cm−1.
N-Butyl-N-ethylpiperidinium bis(trifluoromethanesulfonyl)imide
White powder. Yield: 4.31 g (86%). Tg −75 °C (lit. −72 °C (ref. 57)). Tdec 360 °C (lit. 371 °C). 1H NMR (CDCl3): δ 3.30–3.18 (m, 6H, NCH2 × 2, NCH2 ring), 3.09 (m, 2H, NCH2 ring), 1.77 (m, 4H, NCH2CH2 ring), 1.55 (m, 2H), 1.51 (m, 2H), 1.19 (t, 3H, 3JHH = 7 Hz, NCH2CH3), 0.89 (t, 3H, 3JHH = 7 Hz, CH3) ppm. 13C{1H} NMR (CDCl3): δ 119.80 (q, 1JCF = 321 Hz, CF3), 61.77, 58.84, 58.02, 53.66, 23.01, 20.63, 19.35 (2 resonances), 13.13, 10.72, 6.75 ppm. 19F NMR (CDCl3): δ −79.61(s) ppm. m/z (ESI+): 170 (M+, 100%), 620 ([2M + Br]+, 36). IR (thin film, NaCl): ν 2964(s), 2880(m), 1481(m), 1460(s), 1350(vs), 1331(vs), 1226(vs), 1188(vs), 1138(vs), 1057(vs), 938(w), 788(m) cm−1.
N-Butyl-N-methylpyrrolidinium bis(trifluoromethanesulfonyl)imide
Colourless oil. Yield: 3.91 g (83%). m.p. −15 °C (lit. −15 °C (ref. 58 and 59)); Tg −83 °C (lit. −81 °C;60 −83 °C;58,59 −85 °C;57 −87 °C;61 −89 °C (ref. 62)). Tdec 348 °C. 1H NMR (CDCl3): δ 3.16 (m, 4H, NCH2 and NCH2 ring), 2.96 (m, 2H, NCH2 ring), 2.68 (s, 3H, NCH3), 1.89 (m, 4H, NCH2CH2 ring), 1.41 (m, 2H, NCH2CH2), 1.06 (m, 2H, CH2CH3), 0.64 (t, 3H, 3JHH = 7 Hz, CH3) ppm. 13C{1H} NMR (CDCl3): δ 119.58 (q, 1JCF = 321 Hz, CF3), 64.75, 64.06, 47.82, 25.15, 21.03, 19.06, 12.70 ppm. 19F NMR (CDCl3): δ −80.03(s) ppm. m/z (ESI+): 142 (M+, 100%), 564 ([2M + Br]+, 40). IR (thin film, NaCl): ν 2968(m), 2941(m), 2880(m), 1466(m), 1350(vs), 1331(s), 1192(vs), 1138(s), 1057(vs), 929(m), 788(m) cm−1.
N-Butyl-N-methylpiperidinium bis(trifluoromethanesulfonyl)imide
Colourless oil. Yield: 4.22 g (87%). Tg −72 °C (lit. −73 °C;63 −75 °C;57,64 −76 °C;60 −77 °C (ref. 62)). Tdec 331 °C. 1H NMR (CDCl3): δ 3.14 (m, 4H, NCH2 and NCH2 ring), 3.08 (m, 4H, NCH2 ring), 2.81 (s, 3H, NCH3), 1.68 (m, 4H, NCH2CH2 ring), 1.51 (m, 4H, NCH2CH2 and NCH2CH2CH2 ring), 1.20 (m, 2H, CH2CH3), 0.79 (t, 3H, 3JHH = 7 Hz, CH3) ppm. 13C{1H} NMR (CDCl3): δ 119.53 (q, 1JCF = 321 Hz, CF3), 63.79, 60.82, 46.91, 23.11, 20.15, 19.42, 19.01, 18.32, 12.75 ppm. 19F NMR (CDCl3): δ −79.88(s) ppm. m/z (ESI+): 156 (M+, 100%), 592 ([2M + Br]+, 32). IR (thin film, NaCl): ν 2964(s), 2880(m), 1468(m), 1350(vs), 1331(vs), 1226(vs), 1196(vs), 1138(vs), 1057(vs), 937(m), 789(m) cm−1.
5-Azonaspiro[4.4]nonane bis(trifluoromethanesulfonyl)imide
White powder. Yield: 4.04 g (90%). m.p. 113–115 °C (lit. 113 °C (ref. 48)). Tdec 322 °C. 1H NMR (CDCl3): δ 3.32 (m, 8H, NCH2 ring), 2.05 (m, 8H, NCH2CH2 ring) ppm. 13C{1H} NMR (CDCl3): δ 119.65 (q, 1JCF = 321 Hz, CF3), 62.84, 21.63 ppm. 19F NMR (CDCl3): δ −79.83(s) ppm. m/z (ESI+): 126 (M+, 100%), 532 ([2M + NTf2]+, 46). IR (thin film, NaCl): ν 2980(w), 2944(w), 2902(w), 1463(m), 1350(vs), 1222(s), 1187(vs), 1138(s), 1056(s), 789(m), 739(m) cm−1.
6-Azonaspiro[5.5]undecane bis(trifluoromethanesulfonyl)imide
White powder. Yield: 4.31 g (89%). m.p. 127–129 °C (lit. 130 °C (ref. 48)). Tdec 316 °C. 1H NMR (CDCl3): δ 3.25 (t, 8H, 3JHH = 6 Hz, NCH2), 1.74 (m, 8H, NCH2CH2), 1.61 (dt, 4H, 3JHH = 6 Hz, N–CH2CH2CH2) ppm. 13C{1H} NMR (CDCl3): δ 119.86 (q, 1JCF = 321 Hz, CF3), 59.67, 21.14, 19.16 ppm. 19F NMR (CDCl3): δ −79.58(s) ppm. m/z (ESI+): 154 (M+, 100%), 588 ([2M + NTf2]+, 30). IR (thin film, NaCl): ν 2957(w), 2881(w), 1470(m), 1352(vs), 1333(s), 1184(vs), 1138(s), 1053(s), 891(m), 795(m) cm−1.
5-Azonaspiro[5.4]decane bis(trifluoromethanesulfonyl)imide
White powder. Yield: 4.13 g (88%). m.p. 123–125 °C. Tdec 330 °C. 1H NMR (CDCl3): δ 3.39 (m, 4H), 3.22 (m, 4H), 2.09 (m, 4H), 1.77 (m, 4H), 1.58 (m, 2H) ppm. 13C{1H} NMR (CDCl3): δ 119.74 (q, 1JCF = 321 Hz, CF3), 62.67, 60.70, 21.22, 21.09, 20.71 ppm. 19F NMR (CDCl3): δ −79.68(s) ppm. m/z (ESI+): 140 (M+, 100%), 560 ([2M + NTf2]+, 23). IR (thin film, NaCl): ν 2962(w), 1350(vs), 1335(m), 1221(m), 1184(vs), 1136(m), 1055(m), 788(m) cm−1.
N,N-Dioctylpyrrolidinium bromide
White powder. Yield: 3.91 g (94%). m.p. 126–127 °C (lit. 120–124 °C (ref. 65)). Tdec 207 °C. 1H NMR (CDCl3): δ 3.43 (t, 4H, 3JHH = 6 Hz, NCH2), 3.06 (m, 4H, NCH2 ring), 1.91 (m, 4H, NCH2CH2), 1.32 (m, 4H, NCH2CH2 ring), 0.97 (m, 8H), 0.87 (m, 18H), 0.48 (t, 3JHH = 7 Hz, CH3) ppm. 13C{1H} NMR (CDCl3): δ 62.43, 59.05, 30.91, 28.38, 28.28, 25.68, 22.87, 21.84, 21.38, 13.34 ppm. m/z (ESI+): 296 (M+, 100%), 671 ([2M + Br]+, 19). IR (thin film, NaCl): ν 2956(vs), 2926(vs), 2854(s), 1462(s), 1275(m), 165(m) cm−1.
N,N-Dioctylpiperidinium bromide
White powder. Yield: 4.09 g (94%). m.p. 149–151 °C (lit. 144–146 °C (ref. 65)). Tdec 202 °C. 1H NMR (CDCl3): δ 3.31 (t, 4H, 3JHH = 6 Hz, NCH2), 3.07 (m, 4H, NCH2 ring), 1.53 (m, 4H, NCH2CH2), 1.44 (m, 2H, NCH2CH2CH2 ring), 1.27 (m, 4H, NCH2CH2), 0.96 (m, 8H), 0.85 (m, 18H), 0.46 (t, 6H, 3JHH = 7 Hz, CH3) ppm. 13C{1H} NMR (CDCl3): δ 58.83, 57.70, 30.84, 28.33, 28.24, 25.66, 21.78, 20.97, 19.98, 19.27, 13.27 ppm. m/z (ESI+): 310 (M+, 100%), 701 ([2M + Br]+, 11). IR (thin film, NaCl): ν 2953(vs), 2926(vs), 2856(s), 1475(m), 1454(m), 1280(m), 1265(s), 733(vs) cm−1.
N,N-Dibenzylmorpholinium bis(trifluoromethanesulfonyl)imide
White powder. Yield: 4.75 g (78%). m.p. 149–151 °C. Tdec 265 °C. 1H NMR (CDCl3): δ 7.60–7.40 (m, 10H, ArH), 4.64 (s, 4H, NCH2Ph), 4.12 (m, 4H, NCH2 ring), 3.42 (m, 4H, OCH2 ring) ppm. 13C{1H} NMR (CDCl3): δ 133.56, 131.46, 129.86, 125.60, 119.98 (q, 1JCF = 321 Hz, CF3), 64.51, 60.49, 53.78 ppm. 19F NMR (CDCl3): δ −78.74(s) ppm. m/z (ESI+): 268 (M+, 100%), 815 ([2M + NTf2]+, 2). IR (thin film, NaCl): ν 3069(s), 3039(s), 3019(s), 2983(m), 2941(m), 2917(m), 2885(s), 1499(s), 1454(vs), 1330(vs), 1266(s), 1185(vs), 1137(vs), 740(s) cm−1.
N,N-Dibutylmorpholinium bis(trifluoromethanesulfonyl)imide
Pale yellow oil. Yield: 4.66 g (87%). Tg −48 °C. Tdec 327 °C. 1H NMR (CDCl3): δ 3.95 (m, 4H, CH2N ring), 3.43 (m, 3JHH = 7 Hz, 4H, CH2N ring), 3.33 (m, 4H, CH2O ring), 1.62 (m, 4H, CH2CH2N), 1.39 (m, 4H, CH2CH3), 0.99 (t, 3JHH = 7 Hz, CH3) ppm. 13C{1H} NMR (CDCl3): δ 119.92 (q, 1JCF = 321 Hz, CF3), 60.38, 59.23, 58.44, 23.27, 19.52, 13.38 ppm. 19F NMR (CDCl3): δ −79.02(s) ppm. m/z (ESI+): 200 (M+, 100%), 680 ([2M + NTf2]+, 1). IR (thin film, NaCl): ν 2970(s), 2882(s), 1480(s), 1352(vs), 1197(vs), 1136(vs), 1057(vs), 969(w), 897(s), 790(s), 740(s) cm−1.
N,N-Dipropylmorpholinium bis(trifluoromethanesulfonyl)imide
Pale yellow oil which crystallized on standing. Yield: 4.35 g (87%). m.p. 50–52 °C. Tdec 301 °C. 1H NMR (CDCl3): δ 4.04 (m, 4H, CH2N ring), 3.51 (t, 3JHH = 7 Hz, 4H, NCH2), 3.40 (m, 4H, CH2O ring), 1.80 (m, 4H, CH2CH3), 1.12 (t, 3JHH = 7 Hz, CH3) ppm. 13C{1H} NMR (CDCl3): δ 120.06 (q, 1JCF = 321 Hz, CF3), 60.86, 60.42, 58.51, 15.04, 10.35 ppm. 19F NMR (CDCl3): δ −79.03(s) ppm. m/z (ESI+): 172 (M+, 100%), 624 ([2M + NTf2]+, 15). IR (thin film, NaCl): ν 3185(m), 2982(s), 2948(s), 2888(s), 1626(m), 1482(vs), 1466(vs), 1422(s), 1354(vs), 1272(m), 1185(vs), 1138(vs), 1115(s), 1058(vs), 954(s), 932(m), 879(s), 790(s), 762(m), 741(s) cm−1.
N,N-Diethylmorpholinium bis(trifluoromethanesulfonyl)imide
Pale yellow solid. Yield: 4.00 g (85%). m.p. 39–41 °C. Tdec 322 °C. 1H NMR (CDCl3): δ 3.89 (m, 4H, CH2N ring), 3.41 (q, 4H, CH2N), 3.32 (m, 4H, CH2O ring), 1.26 (t, 6H, CH3) ppm. 13C{1H} NMR (CDCl3): δ 119.97 (q, 1JCF = 321 Hz, CF3), 60.12, 57.45, 53.87, 6.52 ppm. 19F NMR (CDCl3): δ −79.34(s) ppm. m/z (ESI+): 144 (M+, 100%), 568 ([2M + NTf2]+, 2). IR (thin film, NaCl): ν 3636(w), 3561(w), 2995(m), 2888(m), 1483(s), 1466(s), 1453(s), 1398(m), 1352(vs), 1263(vs), 1196(vs), 1134(vs), 1099(s), 1088(s), 1057(s), 905(s), 850(w), 789(m), 763(w), 741(m) cm−1.
N-Butyl-N-methylmorpholinium bis(trifluoromethanesulfonyl)imide
White powder. Yield: 4.00 g (82%). m.p. 29–31 °C (lit. 29 °C;66 35 °C (ref. 67)). Tdec 294 °C. 1H NMR (CDCl3): δ 3.92 (m, 4H, CH2N ring), 3.38 (m, 6H, CH2O and CH2N), 3.10 (s, 3H, NCH3), 1.69 (m, 2H, NCH2CH2), 1.36 (CH2CH3), 0.98 (t, 3JHH = 7 Hz, CH3) ppm. 13C{1H} NMR (CDCl3): δ 119.97 (q, 1JCF = 321 Hz, CF3), 66.26, 60.58, 60.08, 46.55, 23.38, 19.43, 13.27 ppm. 19F NMR (CDCl3): δ −79.29(s) ppm. m/z (ESI+): 158 (M+, 100%), 596 ([2M + NTf2]+, 1). IR (thin film, NaCl): ν 3635(w), 3560(w), 2971(s), 2883(s), 1479(s), 1434(m), 1352(vs), 1272(m), 1198(vs), 1137(vs), 1118(s), 1085(vs), 983(w), 946(w), 912(m), 896(s), 790(s), 763(w), 741(s) cm−1.
8-Oxa-5-azaspiro[4.5]decan-5-ium bis(trifluoromethanesulfonyl)imide
Pale yellow solid. Yield: 3.12 g (66%). m.p. 73–75 °C. Tdec 369 °C. 1H NMR (CDCl3): δ 3.88 (m, 4H, CH2N ring), 3.63 (m, CH2O ring), 3.55 (m, 4H, CH2N ring), 2.16 (m, 4H, CH2 ring) ppm. 13C{1H} NMR (CDCl3): δ 119.72 (q, 1JCF = 321 Hz, CF3), 63.33, 62.02, 59.55, 21.19 ppm. 19F NMR (CDCl3): δ −79.25(s) ppm. m/z (ESI+): 142 (M+, 100%), 564 ([2M + NTf2]+, 3). IR (thin film, NaCl): ν 3066(w), 2983(m), 2883(m), 1468(s), 1410(w), 1353(vs), 1332(vs), 1191(vs), 1135(s), 1056(vs), 969(w), 933(s), 893(m), 790(m), 763(w), 741(s) cm−1.
3-Oxa-6-azaspiro[5.5]undecan-6-ium bis(trifluoromethanesulfonyl)imide
Pale yellow solid. Yield: 3.10 g (64%). m.p. 119–121 °C. Tdec 359 °C. 1H NMR (CDCl3): δ 3.87 (m, 4H, CH2O ring), 3.40 (m, 8H, 2 × CH2N ring), 1.82 (m, 4H CH2 ring), 1.66 (m, 2H, CH2 ring) ppm. 13C{1H} NMR (CDCl3): δ 119.75 (q, 1JCF = 321 Hz, CF3), 60.32, 59.90, 58.48, 21.08, 19.05 ppm. 19F NMR (CDCl3): δ −79.22(s) ppm. m/z (ESI+): 156 (M+, 100%). IR (thin film, NaCl): ν 3065(w), 2955(m), 2883(m), 1466(s), 1352(vs), 1310(m), 1279(w), 1255(w), 1201(vs), 1136(vs), 1125(vs), 1060(vs), 997(m), 942(m), 918(m), 901(s), 854(m), 791(m), 763(w), 740(s) cm−1.
N,N-Dibenzyl-2,3-dihydro-1H-isoindolinium bromide
White powder. Yield: 3.04 g (72%). m.p. 257–259 °C (dec). Tdec 175 °C. 1H NMR (CDCl3): δ 7.66 (m, 4H, ArH), 7.38 (m, 6H, ArH), 7.24 (m, 4H, ArH), 5.36 (s, 4H, NCH2Ph), 5.12 (s, 4H, ArCH2) ppm. 13C{1H} NMR (CDCl3): δ 133.41, 132.71, 130.77, 129.40, 129.39, 127.97, 123.18, 64.76, 62.47 ppm. m/z (ESI+): 300 (M+, 100%), 680 ([2M + 80Br]+, 8). IR (thin film, NaCl): ν 3043(m), 2973(m), 2883(w), 1496(m), 1457(s), 1391(w), 1351(s), 1260(w), 1195(vs), 1137(m), 1056(m), 1028(s), 935(w), 876(m), 786(m), 749(vs) cm−1.
N,N-Dibutyl-2,3-dihydro-1H-isoindolinium bis(trifluoromethanesulfonyl)imide
Yellow oil. Yield: 5.46 g (96%). Tm −44 °C. Tdec 340 °C. 1H NMR (CDCl3): δ 7.57 (m, 4H, ArH), 5.04 (s, 4H, ArCH2), 3.66 (m, 4H, NCH2), 1.89 (m, 4H, NCH2CH2), 1.59 (m, 4H, CH2CH3), 1.16 (t, 6H, 3JHH = 7 Hz, CH3) ppm. 13C{1H} NMR (CDCl3): δ 132.12, 129.48, 122.91, 119.83 (q, 1JCF = 321 Hz, CF3), 67.58, 62.42, 25.23, 19.43, 13.14 ppm. 19F NMR (CDCl3): δ −79.40(s) ppm. m/z (ESI+): 232 (M+, 100%), 743 ([2M + NTf2]+, 25). IR (thin film, NaCl): ν 2966(s), 2939(s), 2880(m), 1466(m), 1342(s), 1227(vs), 1177(vs), 1138(vs), 1053(vs), 883(m), 789(m) cm−1.
N,N-Dipropyl-2,3-dihydro-1H-isoindolinium bis(trifluoromethanesulfonyl)imide
Pale yellow oil. Yield: 5.11 g (95%). Tm −43 °C. Tdec 365 °C. 1H NMR (CDCl3): δ 7.40–7.25 (m, ArH), 4.82 (s, 4H, ArCH2), 3.40 (m, 4H, NCH2), 1.71 (m, 4H, CH2CH3), 0.97 (t, 3JHH = 7 Hz, 6H, CH3) ppm. 13C{1H} NMR (CDCl3): δ 132.11, 129.63, 122.93, 119.85 (q, 1JCF = 321 Hz, CF3), 67.64, 64.29, 17.06, 10.40 ppm. 19F NMR (CDCl3): δ −78.91(s) ppm. m/z (ESI+): 204 (M+, 100%), 688 ([2M + NTf2]+, 20). IR (thin film, NaCl): ν 2980(s), 2947(s), 2888(m), 1479(s), 1466(s), 1350(vs), 1268(m), 1185(vs), 1139(vs), 1057(vs), 1003(m), 959(m), 949(m), 870(m), 790(s), 742(vs) cm−1.
N,N-Diethyl-2,3-dihydro-1H-isoindolinium bis(trifluoromethanesulfonyl)imide
White powder. Yield: 4.25 g (84%). m.p. 81–83 °C. Tdec 354 °C. 1H NMR (CDCl3): δ 7.40–7.32 (m, 4H, ArH), 4.78 (s, 4H, ArCH2), 3.54 (q, 4H, 3JHH = 7 Hz, NCH2CH3), 1.35 (t, 6H, 3JHH = 7 Hz, CH3) ppm. 13C{1H} NMR (CDCl3): δ 132.10, 129.72, 123.10, 119.90 (q, 1JCF = 321 Hz, CF3), 66.86, 57.30, 8.89 ppm. 19F NMR (CDCl3): δ −79.04(s) ppm. m/z (ESI+): 176 (M+, 100%), 631 ([2M + NTf2]+, 1). IR (thin film, NaCl): ν 2995(m), 2955(m), 2887(w), 1466(vs), 1407(s), 1351(vs), 1185(vs), 1139(vs), 1057(vs), 989(s), 945(m), 872(w), 817(m), 792(s), 760(s), 741(s) cm−1.
N-Butyl-N-methyl-2,3-dihydro-1H-isoindolinium bis(trifluoromethanesulfonyl)imide
Pale yellow oil. Yield: 4.79 g (92%). m.p. −54 °C; Tg −17 °C. Tdec 363 °C. 1H NMR (CDCl3): δ 7.41–7.35 (m, 4H, ArH), 4.83 (d, 2H, 2JHH = 15 Hz, ArCH), 4.72 (d, 2H, 2JHH = 15 Hz, ArCH), 3.54 (m, 2H, NCH2), 3.18 (s, 3H, NCH3), 1.77 (m, 2H, NCH2CH2), 1.40 (m, 2H, CH2CH3), 0.96 (t, 3H, 3JHH = 7 Hz, CH2CH3) ppm. 13C{1H} NMR (CDCl3): δ 131.95, 129.68, 123.47, 119.82 (q, 1JCF = 321 Hz, CF3), 69.41, 65.03, 50.11, 25.76, 19.46, 13.26 ppm. 19F NMR (CDCl3): δ −79.01(s) ppm. m/z (ESI+): 190 (M+, 100%), 660 ([2M + NTf2]+, 1). IR (thin film, NaCl): ν 3080(br m), 2970(s), 2943(m), 2881(m), 1483(s), 1476(s), 1428(m), 1351(vs), 1189(vs), 1139(vs), 1057(vs), 972(w), 942(w), 929(m), 913(m), 882(w), 790(s), 742(s) cm−1.
N-Butyl-N-ethyl-2,3-dihydro-1H-isoindolinium bis(trifluoromethanesulfonyl)imide
Pale yellow oil. Yield: 4.38 g (83%). Tm −54 °C; Tg −10 °C. Tdec 347 °C. 1H NMR (CDCl3): δ 7.41–7.31 (m, 4H, ArH), 4.80 (d, 4H, ArCH2), 3.43 (m, 4H, NCH2), 1.67 (m, 2H, NCH2CH2), 1.38 (m, 2H, CH2CH3), 1.35 (t, 3H, 3JHH = 7 Hz, CH3), 0.94 (t, 3H, 3JHH = 7 Hz, CH3) ppm. 13C{1H} NMR (CDCl3): δ 132.11, 129.68, 123.07, 119.89 (q, 1JCF = 321 Hz, CF3), 67.27, 61.49, 57.86, 25.29, 19.57, 13.32, 8.97 ppm. 19F NMR (CDCl3): δ −78.94(s) ppm. m/z (ESI+): 204 (M+, 100%), 688 ([2M + NTf2]+, 17). IR (thin film, NaCl): ν 2969(m), 2938(m), 2881(m), 1466(m), 1405(s), 1352(vs), 1340(s), 1197(vs), 1139(vs), 1057(vs), 942(w), 872(w), 790(m), 761(s), 741(s) cm−1.
Spiro[isoindolin-2,1′-pyrrolidin]-1′-ium bis(trifluoromethanesulfonyl)imide
Pale orange solid. Yield: 4.58 g (91%). m.p. 74–76 °C. Tdec 391 °C. 1H NMR (CDCl3): δ 7.37 (m, 4H, ArH), 4.79 (s, 4H, ArCH2), 3.69 (m, 4H, NCH2), 2.32 (m, 4H, NCH2CH2) ppm. 13C{1H} NMR (CDCl3): δ 132.42, 129.51, 123.33, 119.75 (q, 1JCF = 321 Hz, CF3), 67.52, 63.89, 21.52 ppm. 19F NMR (CDCl3): δ −79.09(s) ppm. m/z (ESI+): 174 (M+, 100%), 628 ([2M + NTf2]+, 15). IR (thin film, NaCl): ν 3061(m), 2998(m), 2900(w), 1482(m), 1466(s), 1351(vs), 1269(s), 1198(vs), 1140(vs), 1058(vs), 976(m), 941(w), 907(m), 790(s), 739(vs) cm−1.
Spiro[isoindolin-2,1′-piperidin]-1′-ium bis(trifluorometh-anesulfonyl)imide
Pale yellow solid. Yield: 4.63 g (89%). m.p. 58–60 °C. Tdec 386 °C. 1H NMR (CDCl3): δ 7.41–7.31 (m, 4H, ArH), 4.79 (s, 4H, ArCH2), 3.55 (m, 4H, NCH2), 1.94 (m, 4H, NCH2CH2), 1.75 (m, 2H, NCH2CH2CH2) ppm. 13C{1H} NMR (CDCl3): δ 131.68, 129.71, 123.70, 119.91 (q, 1JCF = 321 Hz, CF3), 67.70, 61.37, 21.35, 20.76 ppm. 19F NMR (CDCl3): δ −78.94(s) ppm. m/z (ESI+): 188 (M+, 100%), 656 ([2M + NTf2]+, 22). IR (thin film, NaCl): ν 3028(m), 2957(s), 2880(m), 1482(m), 1451(s), 1404(w), 1360(vs), 1341(vs), 1264(m), 1201(vs), 1137(vs), 1058(vs), 1037(s), 983(m), 952(m), 925(m), 788(s), 763(m), 738(m) cm−1.
Acknowledgements
The Australian Research Council supported this research. The authors would also like to thank John Morris Scientific Pty Ltd for the generous loan of the Biotage Initiator 2.5 microwave system.
Notes and references
- D. R. MacFarlane, M. Forsyth, E. I. Izgorodina, A. P. Abbott, G. Annat and K. Fraser, Phys. Chem. Chem. Phys., 2009, 11, 4962–4967 RSC.
- G. B. Appetecchi, M. Montanino, D. Zane, M. Carewska, F. Alessandrini and S. Passerini, Electrochim. Acta, 2009, 54, 1325–1332 CrossRef CAS PubMed.
- M. Galiński, A. Lewandowski and I. Stępniak, Electrochim. Acta, 2006, 51, 5567–5580 CrossRef PubMed.
- J. L. Anthony, J. F. Brennecke, J. D. Holbrey, E. J. Maginn, R. A. Mantz, R. D. Rogers, P. C. Trulove, A. E. Visser, T. Welton, in Ionic Liquids in Synthesis, ed. P. Wasserscheid and T. Welton, Wiley-VCH, Weinheim, 2002, pp. 41–126 Search PubMed.
- T. Welton, Coord. Chem. Rev., 2004, 248, 2459–2477 CrossRef CAS PubMed.
- T. Welton, Chem. Rev., 1999, 99, 2071–2083 CrossRef CAS PubMed.
- Ionic Liquids in Synthesis, ed. P. Wasserscheid and T. Welton, Wiley-VCH, Weinheim, 2002 Search PubMed.
- L. E. Barrosse-Antle, A. M. Bond, R. G. Compton, A. M. O'Mahoney, E. I. Rogers and D. S. Silvester, Chem. –Asian J., 2010, 5, 202–230 CrossRef CAS PubMed.
- S. Ferrari, E. Quartarone, P. Mustarelli, A. Magistris, S. Protti, S. Lazzaroni, M. Fagnoni and A. Albini, J. Power Sources, 2009, 194, 45–50 CrossRef CAS PubMed.
- H. Matsumoto, H. Sakaebe and K. Tatsumi, J. Power Sources, 2005, 146, 45–50 CrossRef CAS PubMed.
- H. Nakagawa, Y. Fujino, S. Kozono, Y. Katayama, T. Nukuda, H. Sakaebe, H. Matsumoto and K. Tatsumi, J. Power Sources, 2007, 174, 1021–1026 CrossRef CAS PubMed.
- H. Sakaebe and H. Matsumoto, Electrochem. Commun., 2003, 5, 594–598 CrossRef CAS.
- H. Sakaebe, H. Matsumoto and K. Tatsumi, Electrochim. Acta, 2007, 53, 1048–1054 CrossRef CAS PubMed.
- J.-H. Shin, W. A. Henderson, S. Scaccia, P. P. Prosini and S. Passerini, J. Power Sources, 2006, 156, 560–566 CrossRef CAS PubMed.
- T. Sato, G. Masuda and K. Takagi, Electrochim. Acta, 2004, 49, 3603–3611 CrossRef CAS PubMed.
- C. Arbizzani, M. Biso, D. Cericola, M. Lazzari, F. Soavi and M. Mastragostino, J. Power Sources, 2008, 185, 1575–1579 CrossRef CAS PubMed.
- M. A. B. H. Susan, M. Yoo, H. Nakamoto and M. Watanabe, Chem. Lett., 2003, 32, 836–837 CrossRef CAS.
- W. Xu and C. A. Angell, Science, 2003, 302, 422–425 CrossRef CAS PubMed.
- H. Matsuoka, H. Nakamoto, M. A. B. H. Susan and M. Watanabe, Electrochim. Acta, 2005, 50, 4015–4021 CrossRef CAS PubMed.
- Q. Dai, D. B. Menzies, D. R. MacFarlane, S. R. Batten, S. Forsyth, L. Spiccia, Y.-B. Cheng and M. Forsyth, C. R. Chim., 2006, 9, 617–621 CrossRef CAS PubMed.
- N. Papageorgiou, Y. Athanassov, M. Armand, P. Bonhôte, H. Petterson, A. Azam and M. Grätzel, J. Electrochem. Soc., 1996, 143, 3099–3108 CrossRef CAS PubMed.
- P. Wang, S. M. Zakeeruddin, I. Exnar and M. Grätzel, Chem. Commun., 2002, 2972–2973 RSC.
- P. Wang, S. M. Zakeeruddin, M. Grätzel, W. Kantlehner, J. Mezger, E. V. Stoyanov and O. Scherr, Appl. Phys. A: Mater. Sci. Process., 2004, 79, 73–77 CrossRef CAS PubMed.
- P. Wang, S. M. Zakeeruddin, R. Humphry-Baker and M. Grätzel, Chem. Mater., 2004, 16, 2694–2696 CrossRef CAS.
- F. Endres, ChemPhysChem, 2002, 3, 144–154 CrossRef CAS.
- M. E. Van Valkenburg, R. L. Vaughn, M. Williams and J. S. Wilkes, Thermochim. Acta, 2005, 425, 181–188 CrossRef CAS PubMed.
- C. Ye, W. Liu, Y. Chen and L. Yu, Chem. Commun., 2001, 2244–2245 RSC.
- Q. Lu, H. Wang, C. Ye, W. Liu and Q. Xue, Tribol. Int., 2004, 37, 547–552 CrossRef CAS PubMed.
- A. E. Jiménez, M. D. Bermúdez, P. Iglesias, F. J. Carrión and G. Martínez-Nicolás, Wear, 2006, 260, 766–782 CrossRef PubMed.
- D. J. Tempel, P. B. Henderson and J. R. Brzozowski, US Pat. 7172646, 2007.
- D. J. Tempel, P. B. Henderson, J. R. Brzozowski, R. M. Pearlstein and D. Garg, US Pat. 2006/0060818 A1, 2006.
- J. L. Anderson, J. K. Dixon and J. F. Brennecke, Acc. Chem. Res., 2007, 40, 1208–1216 CrossRef CAS PubMed.
- J. L. Anthony, J. L. Anderson, E. J. Maginn and J. F. Brennecke, J. Phys. Chem. B, 2005, 109, 6366–6374 CrossRef CAS PubMed.
- C. Myers, H. Pennline, D. Luebke, J. Ilonich, J. K. Dixon, E. J. Maginn and J. F. Brennecke, J. Membr. Sci., 2008, 322, 28–31 CrossRef CAS PubMed.
- B. A. Voss, J. E. Bara, D. L. Gin and R. D. Noble, Chem. Mater., 2009, 21, 3027–3029 CrossRef CAS.
- A. E. Visser, R. P. Swatloski, W. M. Reichert, R. Mayton, S. Sheff, A. Wierzbicki, J. H. Davis Jr and R. D. Rogers, Chem. Commun., 2001, 135–136 RSC.
- S. Dai, Y. H. Ju and C. E. Barnes, J. Chem. Soc., Dalton Trans., 1999, 1201–1202 RSC.
- A. E. Visser, R. P. Swatloski, W. M. Reichert, S. T. Griffin and R. D. Rogers, Ind. Eng. Chem. Res., 2000, 39, 3596–3604 CrossRef CAS.
- V. Fábos, D. Lantos, A. Bodor, A.-M. Balint, L. T. Mika, O. E. Sielcken, A. Cuiper and I. T. Horváth, ChemSusChem, 2008, 1, 189–192 CrossRef PubMed.
- C. R. Allen, P. L. Richard, A. J. Ward, L. G. A. van de Water, A. F. Masters and T. Maschmeyer, Tetrahedron Lett., 2006, 47, 7367–7370 CrossRef CAS PubMed.
- K. Fukumoto, M. Yoshizawa and H. Ohno, J. Am. Chem. Soc., 2005, 127, 2398–2399 CrossRef CAS PubMed.
- A. K. Burrell, R. E. Del Sesto, S. N. Baker, T. M. McClesky and G. A. Baker, Green Chem., 2007, 9, 449–454 RSC.
- Y. Ju and R. S. Varma, J. Org. Chem., 2006, 71, 135–141 CrossRef CAS PubMed.
- C. O. Kappe, Angew. Chem., Int. Ed., 2004, 43, 6250–6284 CrossRef CAS PubMed.
- P. Lisdström, J. P. Tierney, B. Wathey and J. Westman, Tetrahedron, 2001, 57, 9225–9283 CrossRef.
- J. E. Baldwin, J. Chem. Soc., Chem. Commun., 1976, 743 Search PubMed.
- R. A. Aitken, E. F. Philip, F. G. Riddell and M. H. Smith, ARKIVOC, 2002, iii, 63–70 CrossRef.
- Y. Abu-Lebdeh, E. Austin and I. J. Davidson, Chem. Lett., 2009, 38, 782–783 CrossRef CAS.
- H. Booth, A. H. Bostock, N. C. Franklin, D. V. Griffiths and J. H. Little, J. Chem. Soc., Perkin Trans. 2, 1978, 899–907 RSC.
- R. A. da Silva, I. H. S. Estevam and L. W. Beiber, Tetrahedron Lett., 2007, 48, 7680–7682 CrossRef CAS PubMed.
- M. Tajbakhsh, R. Hosseinzadeh, H. Alinezhad, S. Ghahari, A. Heydari and S. Khaksar, Synthesis, 2011, 490–496 CrossRef CAS PubMed.
- J. P. Adams, C. M. Alder, I. Andrews, A. M. Bullion, M. Campbell-Crawford, M. G. Darcy, J. D. Hayler, R. K. Henderson, C. A. Oare, I. Pendrak, A. M. Redman, L. E. Shuster, H. F. Sneddon and M. D. Walker, Green Chem., 2013, 15, 1542–1549 RSC.
- M. Deetlefs and K. R. Seddon, Green Chem., 2010, 12, 17–30 RSC.
- P. T. Anastas and J. C. Warner, Green Chemistry: Theory and Practice, Oxford University Press, New York, 1998 Search PubMed.
- G. R. Heal, in Principles of Thermal Analysis and Calorimetry, ed. P. J. Haines, Royal Society of Chemistry, Cambridge, 2002 Search PubMed.
- A. Martinelli, A. Martic, P. Jacobsson, L. Börjesson, A. Fernicola and B. Scrosati, J. Phys. Chem. B, 2009, 113, 11247–11251 CrossRef CAS PubMed.
- M. Furlani, I. Albinsson, B.-E. Mellander, G. B. Appetecchi and S. Passerini, Electrochim. Acta, 2011, 57, 220–227 CrossRef CAS PubMed.
- H. Tokuda, K. Ishii, M. A. B. H. Susan, S. Tsuzuki, K. Hayamizu and M. Watanabe, J. Phys. Chem. B, 2006, 110, 2833–2839 CrossRef CAS PubMed.
- H. Tokuda, S. Tsuzuki, M. A. B. H. Susan, K. Hayamizu and M. Watanabe, J. Phys. Chem. B, 2006, 110, 19593–19600 CrossRef CAS PubMed.
- T. Abdallah, D. Lemordant and B. Claude-Montigny, J. Power Sources, 2012, 210, 353–359 CrossRef PubMed.
- P. M. Dean, J. M. Pringle, C. M. Forsyth, J. L. Scott and D. R. MacFarlane, New J. Chem., 2008, 32, 2121–2126 RSC.
- Z.-B. Zhou, H. Matsumoto and K. Tatsumi, Chem.–Eur. J., 2006, 12, 2196–2212 CrossRef CAS PubMed.
- F. F. C. Bazito, Y. Kawano and R. M. Torresi, Electrochim. Acta, 2007, 52, 6427–6437 CrossRef CAS PubMed.
- A. Triolo, O. Russina, B. Fazio, G. Battista Appetecchi, M. Carewska and S. Passerini, J. Chem. Phys., 2009, 130, 164521 CrossRef PubMed.
- B. Brycki, A. Szulc and B. Kowalczyk, Molecules, 2010, 15, 5644–5657 CrossRef CAS PubMed.
- K.-S. Kim, S. Choi, D. Demberelnyamba, H. Lee, J. Oh, B.-B. Lee and S.-J. Mun, Chem. Commun., 2004, 828–829 RSC.
- M. Galinski and I. Stepniak, J. Appl. Electrochem., 2009, 39, 1949–1953 CrossRef CAS PubMed.
Footnote |
† Electronic supplementary information (ESI) available: Calculation of overall “greenness” scores. See DOI: 10.1039/c4ra03226h |
|
This journal is © The Royal Society of Chemistry 2014 |