DOI:
10.1039/C4RA02450H
(Paper)
RSC Adv., 2014,
4, 24777-24785
Electrospun polycaprolactone membranes incorporated with ZnO nanoparticles as skin substitutes with enhanced fibroblast proliferation and wound healing
Received
20th March 2014
, Accepted 27th May 2014
First published on 27th May 2014
Abstract
ZnO nanoparticles are well known for their ability to generate Reactive Oxygen Species (ROS) which have a potential role in biological systems. ROS can enhance wound healing by improved cell adhesion and cell migration through growth factor mediated pathways. Here we report the fabrication of electrospun polycaprolactone scaffolds incorporated with ZnO nanoparticles and their ability to perform as skin substitute materials which promote the healing process. The plain or ZnO nanoparticle incorporated PCL membranes were implanted subcutaneously in guinea pigs. Immunological, macroscopical and histological evaluations have shown that the use of membranes containing ZnO nanoparticles enhances the cell adhesion and migration. The ZnO nanoparticle embedded membranes do not show any significant sign of inflammation. The implant also enhanced the wound healing without any scar formation.
1 Introduction
The process of electrospinning enables the production of continuous fibers with dimensions on the scale of nanometers or on submicron scale from a wide range of natural and synthetic polymers.1 High surface area to volume ratio and the high porosity on the sub-micrometer length scale make these materials suitable to be used in many biomedical applications.2–6 Electrospun membranes are extensively used for tissue engineering as well as wound dressing applications.7 Electrospun membranes of polycaprolactone (PCL) and its composites are tried by many workers for various tissue engineering applications.8,9 A large variety of wound dressings have been applied to wounds since ancient times, for effective healing of the wound as well as to prevent wound infection.10 Kim and Yoon have successfully used electrospun PCL membrane as wound dressing material with bacterial barrier and sufficient mechanical properties.11
Role of Reactive Oxygen Species (ROS) like hydrogen peroxide (H2O2) in the cell proliferation and wound healing through the activation of growth factors has been already reported.11–14 Recent studies show that ROS produced in certain situations at optimal level can contribute to increased fitness.15 H2O2 is known for its ability to enhance fibroblast proliferation through mitogen-activated protein kinases (MAPK), Jun-N-terminal kinase (JNK) and p38 MAPK activation.40,41 Further at lower concentrations, ROS could induce smooth muscle as well as epithelial cell proliferation and migration.42,43 H2O2 has been shown to induce pro-MMP-2 activation and subsequent cell motility.44 Further a previous report suggests that ZnO nanoflowers could induce proliferation and migration of endothelial cells and led to the formation of new blood vessels.19 Patra et al. also suggested reactive oxygen species driven angiogenesis by europium hydroxide [EuIII(OH)3] nanorods.20–22 A previous study suggests that the wound site contains micromolar concentrations of H2O2 which will support the healing process where as higher doses of H2O2 adversely influenced healing.13 Hydrogen peroxide (H2O2) enhanced the wound healing in mice by the expression of VEGF.23
Our preliminary study had shown that PCL membranes containing ZnO nanoparticles improved the mechanical property of the membrane, imparted antibacterial property and enhanced cell adhesion property in vitro.16
Zinc oxide nanoparticles (ZnO) are n-type semiconductors, having free electrons associated with anion vacancies in the crystal lattice. A feature of these oxides is that oxygen is chemisorbed as O2− taking electrons from the oxides16–18 as follows,
H2O2 will be formed from O2− as follows,
Thus we hypothesized that ZnO nanoparticles can act as the generators of H2O2 in the tissue engineering scaffold which will act as key regulators at import pathways which enhance cell proliferation and wound healing. ZnO nanoparticles in PCL matrix can play an important role in the generation of ROS, especially H2O2, which may trigger fibroblast proliferation and wound healing through the polymeric membrane. Thus, in this study we are envisaged to develop an electrospun membrane with wound healing ability by incorporating ZnO nanoparticles in the polycaprolactone matrix. Because fibroblast proliferation is necessary for wound healing, their induction will be beneficial in skin tissue engineering for achieving fast wound closure.
2 Experimental
2.1 Materials used
Polycaprolactone (Mw 70
000) used in this study was purchased from Sigma Aldrich, St. Luis, USA. ZnO nanoparticles are of an average particle size of 60 nm and purchased from Sigma Aldrich, St. Luis, USA. Acetone, hematoxylin monohydrate, eosin, glacial acetic acid, NaCl, KCl, Na2HPO4, K2HPO4 and xylene were purchased from Merck, India. Hydrochloric acid, lithium carbonate and mercuric oxide were obtained from Otto Chemie, India. Formaldehyde was purchased from Fisher Scientific, India. C-reactive protein (CRP) assay kit was purchased from Agappe Diagnostics, India. All the reagents used in this study were of analytical grade quality and therefore used without further purification.
2.2 Electrospinning of polycaprolactone with ZnO nanoparticles
PCL membranes incorporated with ZnO nanoparticles were prepared by electrospinning 15 wt% PCL in acetone after dispersing ZnO nanoparticles. The electrospinning apparatus was assembled by Holmarc, India and consisted of a syringe pump, a high voltage power supply and a 10 ml syringe attached with a 21 G diameter needle. The tip to collector distance was maintained as 15 cm and a DC voltage of 18 kV was applied. The feed rate of the solution was precisely controlled by a syringe pumping system which was adjusted to a flow rate of 1 ml h−1. A thin aluminum sheet of 7 cm2 was used as the collector.
Polycaprolactone solutions with different concentration of ZnO nanoparticles were prepared in acetone. Briefly ZnO nanoparticles were accurately weighed and ultrasonicated for 15 minutes to properly disperse in acetone. Then known quantity of PCL was added into the above solution, so that, the final solution contains 15 wt% PCL and stirred using a magnetic stirrer for 12 h for the dissolution of the pellets and proper mixing. 10 ml of the prepared solutions with different weight percent of ZnO nanoparticles were taken in plastic syringes and electrospun individually on aluminum sheets. After drying, the membranes were peeled out from the aluminum sheets for further studies.
2.3 Characterization
The ZnO nanoparticles used in this study were characterized by techniques like UV visible spectroscopy, FTIR analysis, XRD analysis and TEM imaging. The fabricated PCL/ZnO nanocomposite membranes were characterized using SEM and EDX analysis. Animal implantation to evaluate the fibroblast attachment and proliferation, CRP assay for the determination of inflammation and wound healing study were carried out.
2.3.1 UV-Vis spectra analysis. The optical properties (absorbance) of colloidal ZnO nanoparticle solution were evaluated using a Shimadzu double-beam spectrophotometer (model 1700) at a resolution of 1 nm, between 200 and 600 nm.
2.3.2 Fourier transform infrared spectroscopy. FTIR spectra were obtained with a Perkin Elmer, spectrum 400 FTIR spectrometer. The powder of ZnO nanoparticles was subjected to IR analysis. The spectra were collected over a range of 400–4000 cm−1.
2.3.3 X-ray diffraction analysis. X-Ray Diffraction (XRD) was used to understand the crystallinity and the structure of the obtained ZnO nanoparticles. XRD was recorded in the 2θ range of 20°–90° using a model D8-Advance of Bruker (Germany), of CuKα radiation, the energy of which was 8.04 keV and wavelength was 1.54 Å. The applied voltage was 40 kV and current was 25 mA.
2.3.4 TEM analysis of ZnO nanoparticles. To understand the morphology and size distribution, the obtained nanoparticles were imaged using JEOL JEM 2100 high resolution transmission electron microscope. The sample was prepared by air-drying drops of diluted dispersions of the nanoparticles on carbon films supported by copper grids. The particle size was measured using ImageJ software and the average particle size was determined.
2.3.5 Scanning electron microscopy. The morphological features of the fabricated membranes were observed by SEM. The neat PCL membrane as well as PCL/ZnO nanocomposite membranes were carefully sectioned with an approximate size of 3 mm length and 0.5 mm width using a sharp scissor and mounted onto an SEM grid. Prior to the examination, each sample was coated with platinum using a JEOL JFC 1600 Autofine coater. A JEOL JSM 6390 Scanning Electron Microscope at 10 kV was used to analyze the samples.
2.3.6 In vivo implantation. Six 3 month-old American satin guinea pigs were housed in sterilized cages with sterile food and water and filtered air, and were handled with aseptic techniques. All the animal experiments were carried out in accordance with the guidelines of Committee for the Purpose of Control and Supervision of Experiments on Animals (CPCSEA), India with the approval of Institutional Animal Ethics committee, Pushpagiri Research Centre (PRC), Tiruvalla, India. Before implantation, all the membranes were treated with 70% alcohol for 20 minutes and then with ultraviolet radiation for 20 minutes to sterilize them. A small area on the left abdomen of the guinea pigs were shaved, wiped with alcohol, and anesthetized with ketamine hydrochloride injection. A full thickness skin incision wound was made and a skin flap was reflected to expose the subcutaneous tissue, PCL membranes (neat PCL membrane and that contains 1 wt%, 2 wt% and 4 wt% ZnO nanoparticles) were implanted subcutaneously, and the flap was sutured to retain the membrane in the subcutaneous pouch. Visual inspection of the wound bed was carried out to identify any signs of inflammation caused by the membrane.
2.3.7 C-reactive protein (CRP) assay. CRP is a homopentameric protein produced in the liver in response to infection and inflammation.24 It is one of the most rapidly produced inflammatory biomarkers and has the potential to serve as a diagnostic marker in therapeutic settings.25 Qualitative assay of C-Reactive Protein (CRP) was carried out according to the manufacturer's protocol. The CRP sensitivity of the reagent was 0.6 mg dl−1. Blood was collected on each fifth day from the ears of the animal implanted with the neat PCL membranes and that contains ZnO nanoparticles in ethylenediaminetetraacetic acid (EDTA) treated vials. To confirm that there was no inflammation or infection to the animals, CRP assay was carried out to all animals before implantation also. Blood plasma was separated from the blood by centrifugation at 3000 RPM for 10 minutes. The CRP plate (Agappe Diagnostics, India) was cleaned with alcohol and 20 μl of CRP antibody (Agappe Diagnostics, India) was added to each field as per the requirement. 20 μl each of positive and negative CRP control solutions were added to the first and second well respectively. 20 μl of the blood plasma collected from animals implanted with neat and ZnO nanoparticles incorporated membrane were added into each field which contains the antibody. All the controls and samples were mixed thoroughly by rotating the plates in rocking movement such that the aggregate is formed as an outline to the added controls and samples. The samples are then compared with the positive control and those samples which display aggregation near to or than the positive control is considered as affected by a systemic inflammation.
2.3.8 Blood parameters. The blood was collected on each fifth day from the animal implanted with the neat PCL membranes and that contains ZnO nanoparticles were subjected to blood cell counting. Blood was taken from the ears of guinea pigs in ethylenediaminetetraacetic acid (EDTA) treated vials and aspirated into an automatic hematology analyzer (MINDRAY BC-280Vet). The obtained results were expressed as mean with ±standard deviation.
2.3.9 H&E staining. The skin pouch where the membranes implanted was surgically opened on day 5, 10 and 20 to evaluate the tissue integration to the membranes. We have limited this study to the neat PCL membranes and to the membrane containing 1 wt% ZnO nanoparticles based on our in vitro optimization studies.16 Membranes with attached subcutaneous tissue were excised for histological evaluation. The wound was sutured and then allowed to heal naturally.The membranes collected from the animals were fixed in paraformaldehyde solution and dehydrated in a graded series of ethanol before embedding in paraffin. 3 micrometer-thick sections were cut with a rotary microtome (Leica Micron). Hematoxylin and eosin stained sections were investigated for overall morphology, adhesion, migration and proliferation of cells. The stained sections were examined by at least two independent and blinded investigators with a Leica light microscope. The fixed and dehydrated samples were used for SEM analysis also. To get quantitative information regarding the number of cells present on the scaffolds, the H&E stained sections were observed under Polarized Optical Microscope (Leica DM4500 P LED) and images were taken. Obtained images were analyzed using ImageJ software; number of cells per square millimeter area were calculated and expressed as the mean of 6 observations ±S.D.
2.3.10 Wound healing activity. Wound healing study was also carried out as per the guidelines and the approval of ethical committee as given previously. A small area on the dorsal cervical region of the guinea pigs were shaved, wiped with alcohol, anesthetized with ketamine hydrochloride injection and a 2 × 2 cm full thickness skin excision was made. The PCL membranes with 2 × 2 cm dimensions were sutured on the wound. Povidone-iodine ointment treated (each day) wounds and bare wounds were kept as positive and negative controls respectively. The photographs were taken each day until the wounds were perfectly healed. The percentage of wound healing was calculated using the formula,
where W% is the percentage of wound healing, WA0 is the area of wound at 0th day and WAt is the area of wound after different days of healing.
3 Results
3.1 Characterization of nanoparticles
The ZnO nanoparticles used in this study were characterized by techniques like UV-Visible spectroscopy, FTIR analysis, XRD analysis and TEM imaging and the results are given in Fig. 1. The UV-Visible spectroscopic result shows that the ZnO nanoparticles have two absorption peaks at 251 and 349 nm wavelength regions. Presence of two peaks is due to the elongated morphology of nanoparticles. FTIR shows that the nanoparticles have nearly 100% transmittance which is a characteristic feature of ZnO nanoparticles. Further, it did not show any other peaks which indicate the high level of purity of the nanoparticles. Fig. 1(c) represents the X-ray diffraction pattern of ZnO nanopowder. A definite line broadening of the XRD peaks indicates that the prepared material consist of particles in nanoscale range. The diffraction peaks located at 31.84°, 34.52°, 36.33°, 47.63°, 56.71°, 62.96°, 68.13°, and 69.18° have been keenly indexed as hexagonal wurtzite phase of ZnO (JPCDS card number: 36-1451) and further it also confirms the nanopowder was free of impurities as it does not contain any characteristic XRD peaks other than ZnO peaks. The TEM image shows that the nanoparticles are of elongated morphology and have an average particle size of 60 nm.
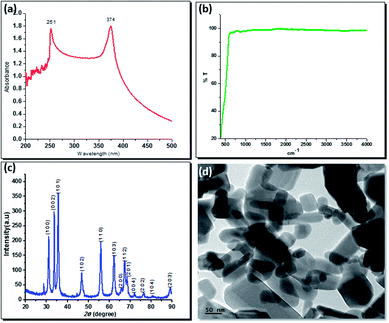 |
| Fig. 1 UV-visible spectrum (a), FTIR spectrum (b), XRD pattern (c) and TEM image (d) of ZnO nanoparticles used for the study. | |
3.2 Morphology of electrospun membranes
Representative morphological features of neat PCL membrane and its nanocomposite with ZnO nanoparticles using SEM are given in Fig. 2. It is evident from the figure that the fabricated membranes were highly porous with randomly oriented fibers. The higher magnification image of PCL membrane containing 1 wt% ZnO nanoparticles confirms the presence of well dispersed nanoparticles on the surface of the fibers (Fig. 2(c)). At higher concentrations aggregates of nanoparticles were formed due to the high surface energy of the nanoparticles (Fig. 2(d)).
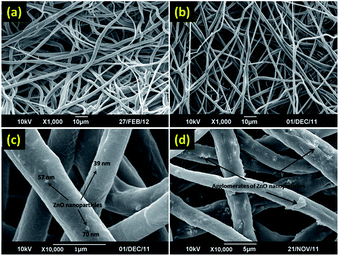 |
| Fig. 2 Representative SEM micrograph of neat PCL membrane (a) and PCL membrane containing 1 wt% ZnO nanoparticles. (c) is the higher magnification micrograph of PCL membrane containing 1 wt% ZnO nanoparticles and (d) is the higher magnification micrograph of PCL membrane containing 6 wt% ZnO nanoparticles. | |
3.3 CRP assay
Determination of CRP in blood samples as an acute phase protein, giving a measure of possible immunological rejection or inflammatory response directed against the tissue engineered constructs, was performed at different time intervals (before implantation, 5, 10 and 20 days after implantation). No increased CRP levels were found in animals implanted with neat PCL membrane or PCL membranes with a ZnO nanoparticle concentration up to 2 wt% (Fig. 3). To get semi-quantitative information about the variation in the CRP level in the blood collected from guinea pigs implanted with the membranes, serial dilution method was adopted and the results are presented in Table 1. In fact, the animals implanted with PCL membrane with 4 wt% ZnO nanoparticles have shown some sign of inflammation at 5th and 20th day of implantation. Since a slight agglutination of latex particles only observed, CRP levels were only slightly higher than the normal range. On 5th day of implantation, PCL membrane containing 4 wt% ZnO nanoparticles produced a CRP level in the range of 0.6–1.2 mg dl−1. This increase in CRP level could be due to the release of excess amount of ZnO nanoparticles present on the surface of the PCL fibers soon after implantation. On the 20th day of implantation, animals implanted with PCL membranes containing 4 wt% ZnO nanoparticles shown to have a CRP level in the range of 1.2–2.4 mg dl−1. This was due to the higher level of ZnO nanoparticles released during degradation of PCL membranes in the animal body.
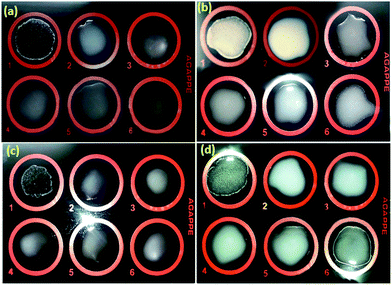 |
| Fig. 3 Slides showing the CRP latex agglutination test of blood taken from guinea pigs before subcutaneous implantation (a) and after 5 days (b), 10 days (c) and 20 days (d) of implantation. In each slide (3) represents neat PCL membrane, (4) represents PCL membrane containing 1 wt% ZnO nanoparticles, (5) represents PCL membrane containing 2 wt% ZnO nanoparticles, (6) PCL containing 4 wt% ZnO nanoparticles (6). In all slides 1 and 2 are positive control and negative control respectively. | |
Table 1 Semi-quantitative results of CRP level in the blood collected from guinea pigs implanted with PCL membranes containing varying amounts of ZnO nanoparticles after different implantation periods
Sample |
CRP level (mg dl−1) |
Before implantation |
5th day |
10th day |
20th day |
PCL alone |
<0.6 |
<0.6 |
<0.6 |
<0.6 |
PCL/ZnO-1% |
<0.6 |
<0.6 |
<0.6 |
<0.6 |
PCL/ZnO-2% |
<0.6 |
<0.6 |
<0.6 |
<0.6 |
PCL/ZnO-4% |
<0.6 |
0.6–1.2 |
<0.6 |
1.2–2.4 |
3.4 Blood parameters
During systemic inflammation or infection, the number of blood cells of animals tends to vary. During inflammation total WBC, neutrophil, lymphocyte, eosinophil and basophil counts normally increase. RBC count may reduce if there is a chronic systemic inflammation. To evaluate the systemic inflammation if it is due to the implantation of the fabricated materials, differential cell counting has been carried out and the results are given in Table 2. The results show that there was no significant variation in number of various blood cells between the neat PCL membrane and the ZnO nanoparticle containing membrane implanted groups.
Table 2 Result of complete blood cell count for the blood taken from the animal after subcutaneous implantation of neat PCL membrane and that containing 1 wt% ZnO nanoparticles for 5, 10 and 30 days
Cell type |
Blood cell count (cells l−1) |
5th day |
10th day |
30th day |
Control |
Neat PCL |
PCL/ZnO-1% |
Control |
Neat PCL |
PCL/ZnO-1% |
Control |
Neat PCL |
PCL/ZnO-1% |
WBC (total) |
8.12 ± 6.4 × 109 |
7.5 ± 9.5 × 109 |
8.52 ± 8.4 × 109 |
10.12 ± 5.2 × 109 |
9.57 ± 8.5 × 109 |
8.22 ± 8.7 × 109 |
8.77 ± 5.4 × 109 |
9.5 ± 7.6 × 109 |
8.62 ± 71 × 109 |
RBC |
4.67 ± 2.1 × 1012 |
4.49 ± 3.6 × 1012 |
5.63 ± 2.1 × 1012 |
4.17 ± 3.1 × 1012 |
4.13 ± 2.6 × 1012 |
4.93 ± 2.1 × 1012 |
5.17 ± 2.9 × 1012 |
4.53 ± 3.5 × 1012 |
5.3 ± 3.1 × 1012 |
Lymphocytes |
4.8 ± 3.5 × 109 |
3.8 ± 2.8 × 109 |
4.05 ± 3.1 × 109 |
5.1 ± 3.3 × 109 |
4.2 ± 2.6 × 109 |
4.7 ± 3.3 × 109 |
3.9 ± 2.4 × 109 |
5.2 ± 2.8 × 109 |
4.12 ± 3.8 × 109 |
Monocytes |
0.38 ± 0.24 × 109 |
0.4 ± 0.17 × 109 |
0.57 ± 0.24 × 109 |
0.48 ± 0.21 × 109 |
0.37 ± 0.14 × 109 |
0.49 ± 0.23 × 109 |
0.31 ± 0.28 × 109 |
0.43 ± 0.09 × 109 |
0.54 ± 0.15 × 109 |
Granulocytes |
3.8 ± 2.4 × 109 |
3.3 ± 2.9 × 109 |
4.9 ± 2.2 × 109 |
4.3 ± 3.4 × 109 |
3.1 ± 2.3 × 109 |
5.1 ± 4.2 × 109 |
3.7 ± 3.1 × 109 |
4.7 ± 2.5 × 109 |
3.8 ± 2.3 × 109 |
Platelets |
240 ± 69 × 109 |
186 ± 56 × 109 |
210 ± 67 × 109 |
190 ± 64 × 109 |
246 ± 71 × 109 |
193 ± 68 × 109 |
220 ± 74 × 109 |
180 ± 77 × 109 |
178 ± 63 × 109 |
3.5 Histological analysis
The effect of PCL membrane containing ZnO nanoparticles on the induction of fibroblast cell proliferation in vivo is shown in Fig. 4 and 5.
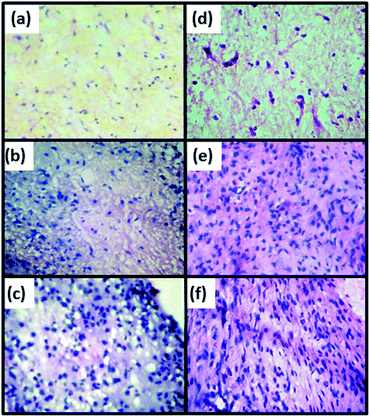 |
| Fig. 4 Histological images (H&E staining) of neat PCL membranes (a–c) and PCL membranes containing 1 wt% ZnO nanoparticles (d–f) after 5 days (a and d), 10 days (b and e) and 20 days (c and f) of subcutaneous implantation in guinea pigs. | |
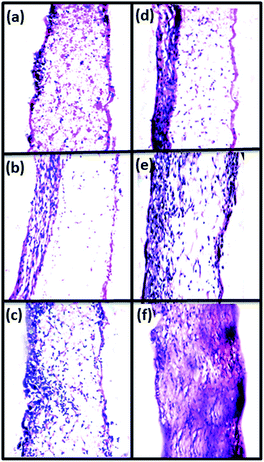 |
| Fig. 5 Cross sections (H&E stained) of the neat PCL membranes (a–c) and membranes containing 1 wt% ZnO nanoparticles (d–f) showing the migration and proliferation of fibroblasts after 5 days (a and d), after 10 days (b and e) after 20 days (c and f) of subcutaneous implantation in guinea pigs. | |
From the transverse sectional histological images, it is clear that the PCL membranes containing 1 wt% of ZnO nanoparticles enhanced the fibroblast proliferation compared to the neat PCL membrane. After 10 days of implantation, large number of fibroblast cells got attached and grown through the PCL membranes with 1 wt% ZnO nanoparticles. However, through neat PCL membranes comparatively less number of fibroblasts could only grow. At 20th day also, PCL membrane with 1 wt% ZnO nanoparticles has shown higher cell attachment and proliferation (Fig. 4(f)). Fibroblast cells have shown their characteristic elongated spindle shaped morphology on PCL membranes that contain ZnO nanoparticles where as in the neat PCL membrane the cells possessed spherical morphology (Fig. 4(c)).
Table 3 shows the number of cells present in the square millimetre area of the scaffold after different implantation periods. During the entire study period, ZnO nanoparticle containing membrane showed higher cell density than the neat membrane.
Table 3 Cell density on implanted membranes containing varying amounts of ZnO nanoparticles after different implantation periods
Sample |
Cell density (cells per mm2) |
After 5 days of implantation |
After 10 days of implantation |
After 20 days of implantation |
PCL alone |
20 ± 6 |
188 ± 4 |
212 ± 12 |
PCL/ZnO-1% |
38 ± 8 |
245 ± 11 |
316 ± 6 |
From the cross sectional images of the membranes the migration and proliferation of fibroblast cells through the membranes was clearly observable (Fig. 5). In both neat and nanoparticle incorporated membranes, the cells were migrated from the body wall side to the cutaneous side of the animal. On the fifth day of implantation, number of fibroblasts was very less in both membranes. On the 10th day of implantation considerably large number of cells migrated from the subcutaneous layer through the membrane towards the skin. In the case of neat PCL membrane, cells were seemed to be limited only to the body wall side whereas in the ZnO nanoparticles incorporated membranes the cells could successfully migrate towards the cutaneous side. On the 20th day, migrating cells could reach the cutaneous side in both membranes. In nanoparticles incorporated membranes, a homogeneous distribution of cells could be observed throughout the entire thickness of the membrane (Fig. 6(f)). This indicates that the ZnO nanoparticle incorporated membrane considerably enhanced fibroblast migration.
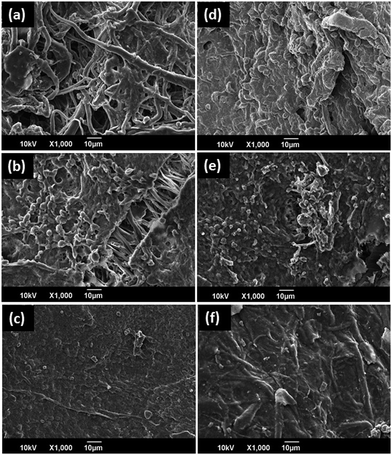 |
| Fig. 6 SEM micrographs of neat PCL membranes (a–c) and PCL membranes containing 1 wt% ZnO nanoparticles (d–f) after 5 (a and d), 10 (b and e) and 20 (c and f) days of subcutaneous implantation in guinea pigs. | |
Even though the cell growth and migration through the membrane were clearly observable from the histological images, the SEM images have been taken to assess the morphology of the cell growth on the surface of the membranes as shown in Fig. 6. On the fifth day of implantation, very less number of cells could be observed on the surface of the neat PCL membrane and the uncovered PCL fibers also could be observed (Fig. 6(a)). Large number of fibroblast cells were observed on the surface of nanoparticle incorporated membranes even on the 5th day, where fibrous surface was not visible due to cell attachment (Fig. 6(d)). On the 10th day of implantation, the number of fibroblast cells attached to the neat PCL membranes was comparatively lower than the same over nanoparticles incorporated membranes (Fig. 6(b) and (e)). On the 20th day, in the case of ZnO nanoparticles incorporated membranes the cells could not be distinguishable due to the higher proliferation and the formation of extracellular matrix (Fig. 6(f)).
3.6 Wound healing
To evaluate the ability of ZnO nanoparticle incorporated membrane on the wound healing, the neat PCL membranes as well as that containing 1 wt% ZnO nanoparticles were sutured in the full thickness wound in guinea pigs. On the day of implantation and each day after the implantation until the wound was completely healed, photographs were taken. Fig. 7 shows the photographs of the wound with both neat and nanoparticle containing membrane on the first day (Fig. 7(a) and (f)), on 5th day (Fig. 7(b) and (g)), on 10th day (Fig. 7(c) and (h)), on 20th day (Fig. 7(d) and (i)) and on 30th day (Fig. 7(e) and (j)) of implantation. On the initial days of wound healing, it was difficult to get a clear idea about the healing process since the wound was covered with the membranes. But the contraction rate was higher for the ZnO nanoparticle incorporated membranes. In between 15th and 20th day of implantation, outer regions of both neat membranes and nanoparticle incorporated membranes expelled from the healing wound along with the debris. After this the wound was clearly observable and there was a notable difference in healing between neat and nanoparticle containing membranes (Fig. 7(d) and (i)). Wounds covered with ZnO nanoparticles containing membrane were healed completely within this period. Both neat PCL membrane and PCL membrane containing ZnO nanoparticles have shown superior wound healing than both positive and negative controls. Animals were kept without any further wound coverage and keeping observed for 30 days. On the 30th day, the wounds were completely healed without any sign of scar formation in the case of nanoparticle incorporated membranes. In neat membrane implanted wounds, hair formation on the healed area was less compared to the other regions. But in the case of ZnO nanoparticle incorporated membranes normal skin with hairs as in other areas has been observed. In the case of povidone-iodine treated wounds, thick scar formation was observed. Negative controls did not heal completely even within 30 days of study period.
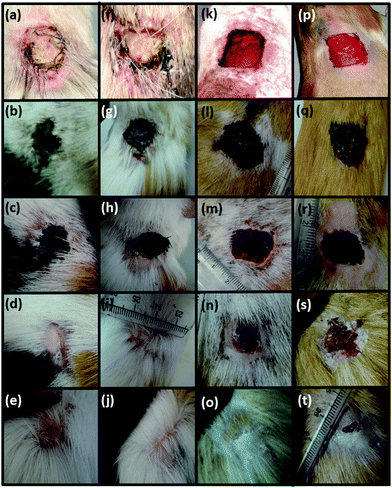 |
| Fig. 7 Wound healing activity of the membrane on the first day (a, f, k and p), on 5th day (b, g, l and q), on 10th day (c, h, m and r), on 20th day (d, I, n and s) and on 30th day (e, j, o and t) of implantation. The first column (a–e) indicates neat PCL membranes, the second column (f–j) indicates PCL membrane incorporated with 1 wt% ZnO nanoparticles, the third column (k–o) indicates povidone-iodine treated wounds (positive controls) and the fourth column (p–t) indicates negative controls. | |
The rate of wound healing was calculated from the area of healing wound until wound was completely healed and has expressed as the percentage of wound healing (Fig. 8). Up to 10th day of healing, there was only a slight variation in the area of wound in both neat and nanoparticle incorporated membranes. But after that onwards nanoparticle incorporated membranes have shown higher rate of wound healing. In the case of PCL membranes with ZnO nanoparticles, 100% of wound healing was achieved on the 25th day of study whereas neat membrane covered wound was still not completely healed. Interestingly both neat PCL membranes and ZnO nanoparticle containing membranes had shown higher rate of wound healing than both positive and negative controls.
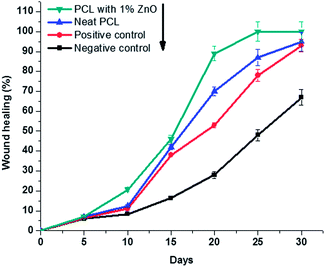 |
| Fig. 8 Percentage of wound healing after the implantation of neat PCL membrane and PCL membrane containing 1 wt% ZnO nanoparticles. | |
4 Discussion
Electrospinning of polycaprolactone,26 its blends,27 nanocomposites28 and their use in tissue engineering as biodegradable scaffolds is already reported. Even though good dispersion of ZnO nanoparticles was observed at lower concentrations, agglomeration of ZnO nanoparticles on the surface of PCL fibers was observed at higher concentrations. As the ZnO nanoparticle content in the electrospun fiber increases, the surface of the fibers becomes rougher due to the agglomerates of ZnO nanoparticles. At 4 wt% ZnO concentration onwards it was very evident which was in agreement with the previous report.28 This is due to the fact that, nanoparticles have high surface energy and are easy to aggregate, which led to the poor dispersion of them in polymer matrix at higher concentrations.29
Subcutaneous implantation of the membrane containing ZnO nanoparticles and subsequent CRP assay and the assessment of blood parameters revealed that there was no systemic inflammation due to the implantation. An elevated level of C-reactive protein in the blood circulation is an indication of systemic inflammation.30 PCL membranes with ZnO nanoparticles up to 2 wt% did not show even a minor sign of inflammation, a minor inflammation was observed at 5th day and 20th day of implantation in the case of 4 wt% ZnO nanoparticle containing membranes. This is due to the higher release of ZnO nanoparticles on the 5th day as well as on the 20th day of implantation. It is evident from the SEM images that agglomerates of ZnO nanoparticles were present on the surface of PCL fibers which contains 4 wt% ZnO nanoparticles. Thus these agglomerates were released faster into the implantation site and lead to inflammation. Higher level of reactive oxygen species is known to produce systemic inflammation.31–35 Thus higher concentration of ZnO nanoparticles can cause inflammation since it will generate higher level of reactive oxygen species.31
Photocatalytic production of reactive oxygen species like H2O2 is well established by previous works.36 Further the cells themselves produce reactive oxygen species in response to the stress induced by ZnO nanoparticles.37
During systemic inflammation the number of blood cells in the blood circulation tends to vary. If inflammation occurs, total WBC, neutrophil, lymphocyte, eosinophil and basophil count normally increases.38 RBC count may reduce if there is a chronic systemic inflammation.39 We did not observe any remarkable variation in the blood cell count during the entire period of implantation.
Histological evaluation clearly shows that 1 wt% ZnO nanoparticle containing membrane possessed good cell adhesion, migration and proliferation property than the neat PCL membrane. These results support our previous in vitro studies where we have got enhanced fibroblast proliferation on ZnO nanoparticles containing PCL membranes.16 These superior properties might be due to the formation of H2O2 and the subsequent increase in the fibroblast proliferation through mitogen-activated protein kinases (MAPK), Jun-N-terminal kinase (JNK) and p38 MAPK activation.40,41 Further at lower concentrations, ROS induced smooth muscle as well as epithelial cell proliferation and migration.42,43 Specifically, H2O2 has been shown to induce pro-MMP-2 activation and cell motility.44
Our experiments revealed that PCL membranes with ZnO nanoparticles had shown higher wound healing activity. The increased wound healing might be due to the increased cell migration and cell proliferation. Further, previous reports suggest that H2O2 can enhance wound healing process. A previous study suggests that the wound site contains micromolar concentrations of H2O2 which will support the healing process where as higher doses of H2O2 adversely influence healing.13 Hydrogen peroxide (H2O2) enhanced the wound healing in mice by the expression of VEGF.23 Thus, we suppose that the H2O2 generated by the ZnO nanoparticles induced the expression of growth factors and/receptors which are the key regulators in fibroblast proliferation which played a significant role in the enhanced wound healing.
The ultimate goal of wound repair should be to regain the original in both form and function, but in mammals including humans, this is not the case. Usually, during wound healing a fibrotic scar which impairs the normal tissue organization and the aesthetics will be the outcome.45 Here we could not observe such a fibrotic scar after the complete healing of the wound which is an indication of regeneration.45
In neat membrane implanted wound, hair formation on the healed area was less compared to the other regions due to the lack of hair follicles which are the stem cells that continuously produce hair as well as other major components of the skin including sebaceous glands and the epidermis.46–49 But in the case of ZnO nanoparticle incorporated membranes normal skin with hairs as in other areas has been formed.
5 Conclusions
In this study, electrospun polycaprolactone (PCL) scaffolds incorporated with zinc oxide nanoparticles were successfully fabricated and characterized. The fabricated membranes were highly porous with randomly oriented fibers. SEM analysis confirmed the presence of ZnO nanoparticles in the electrospun scaffolds. CRP assay shows there was no sign of inflammation in animals where up to 2 wt% of ZnO nanoparticles incorporated while membrane contains 4 wt% ZnO possessed little inflammatory sign. PCL membrane with 1 wt% ZnO nanoparticles has shown excellent fibroblast attachment, cell migration and cell proliferation in vivo. Application of the ZnO nanoparticle containing membranes as skin substitute in full thickness excision wound revealed its ability to promote wound healing without any scar formation. Thus the fabricated material can be used as a successful skin substitute material which will promote fast wound healing by enhancing cell migration and proliferation.
Acknowledgements
The authors acknowledge Department of Science and Technology (DST) and Department of Biotechnology (DBT), Government of India, New Delhi for the financial support through Nanomission and MSUB IPLSARE programs respectively. Funding from UGC SAP, DST-FIST, SAARD-KSCSTE are also gratefully acknowledged.
References
- T. Subbiah, G. S. Bhat and R. W. P. Tock, J. Appl. Polym. Sci., 2005, 96(2), 557–569 CrossRef CAS.
- E. Kenawy, L. B. Gary, M. Kevin, L. John, G. S. David and H. S. Elliot, et al., J. Controlled Release, 2002, 81(1), 57–64 CrossRef CAS.
- H. Y. Yoshimoto, M. Shin, H. Terai and J. P. Vacanti, Biomaterials, 2003, 24(12), 2077–2082 CrossRef CAS.
- P. Wutticharoenmongkol, S. Neeracha, P. Prasit and S. Pitt, Macromol. Biosci., 2006, 6(1), 70–77 CrossRef CAS PubMed.
- B. M. Min, J. Lim, S. N. Young, M. K. Jin, Y. K. Jin and H. P. Won, Int. J. Biol. Macromol., 2004, 34(5), 223–230 CrossRef PubMed.
- S. Agarwal, H. W. Joachim and G. Andreas, Polymer, 2008, 49(26), 5603–5621 CrossRef CAS PubMed.
- E. J. Chong, T. T. Phan, I. J. Lim, Y. Z. Zhang, B. H. Bay and S. Ramakrishna, et al., Acta Biomater., 2007, 3(3), 321–330 CrossRef CAS PubMed.
- W. Zhao, M. J. Young, C. George, A. Anthony, J. Y. James and J. L. Sang, Biomaterials, 2013, 34(33), 8235–8240 CrossRef CAS PubMed.
- C. Erisken, M. K. Dilhan and W. Hongjun, Biomaterials, 2008, 29(30), 4065–4073 CrossRef CAS PubMed.
- R. Augustine, N. Kalarikkal and S. Thomas, in Diabetes Mellitus and Human Health Care: A Holistic Approach to Diagnosis and Treatment, ed. A. George, R. Augustine and M. Sebastian, Apple Academic Press, Oakville, 2014, pp. 273–314 Search PubMed.
- G. H. Kim and Y. Hyeon, Appl. Phys. A, 2008, 90(3), 389–394 CrossRef CAS.
- C. K. Sen, Wound Repair Regen., 2003, 11(6), 431–438 CrossRef.
- S. Roy, K. Savita, N. Kishore, K. H. Thomas and K. S. Chandan, Mol. Ther., 2006, 13(1), 211–220 CrossRef CAS PubMed.
- Y. Huo, Y. Q. Wen, P. Qing, F. Y. Yu, X. Kuiyi and F. L. Marjorie, Exp. Eye Res., 2009, 89(6), 876–886 CrossRef CAS PubMed.
- B. C. Dickinson and J. C. Christopher, Nat. Chem. Biol., 2011, 7(8), 504–511 CrossRef CAS PubMed.
- R. Augustine, H. N. Malik, D. K. Singhal, M. Mukherjee, D. Malakar, N. Kalarikkal and S. Thomas, J. Polym. Res., 2014, 21, 347 CrossRef.
- D. J. M. Bevan and J. S. Anderson, Discuss. Faraday Soc., 1950, 8, 238–246 RSC.
- V. J. Norman, Oxygen chemisorbed on zinc oxide, Aust. J. Chem., 2005, 9(7), 1133–1141 Search PubMed.
- K. B. Ayan, V. S. M. Vimal, M. Joydeb, K. P. Ajay, P. Sujata and P. Krishnendu, et al., Nanoscale, 2012, 4(24), 7861–7869 RSC.
- C. R. Patra, B. Resham, P. Sujata, B. Sujit, M. Priyabrata and M. Debabrata, Clin. Chem., 2007, 53(11), 2029–2031 CAS.
- C. R. Patra, B. Resham, P. Sujata, E. V. Nicholas, G. Alexandra and K. Yuri, et al., Adv. Mater., 2008, 20(4), 753–756 CrossRef CAS.
- C. R. Patra, S. A. M. Soha, W. Enfeng, D. Shamit, P. Sujata and E. Michal, et al., Toxicol. Appl. Pharmacol., 2009, 240(1), 88–98 CrossRef CAS PubMed.
- C. K. Sen, S. Khanna, B. M. Babior, T. K. Hunt, E. C. Ellison and S. Roy, J. Biol. Chem., 2002, 277(36), 33284–33290 CrossRef CAS PubMed.
- D. Thompson, B. P. Mark and P. W. Steve, Structure, 1999, 7(2), 169–177 CrossRef CAS.
- E. M. Macy, E. H. Timothy and P. T. Russell, Clin. Chem., 1997, 43(1), 52–58 CAS.
- H. Yoshimoto, Y. M. Shin, H. Terai and J. P. Vacanti, Biomaterials, 2003, 24(12), 2077–2082 CrossRef CAS.
- M. L. Ghasemi, P. P. Molamma, M. Mohammad, H. N. E. Mohammad and R. Seeram, Biomaterials, 2008, 29(34), 4532–4539 CrossRef PubMed.
- P. Wutticharoenmongkol, S. Neeracha, P. Prasit and S. Pitt, Macromol. Biosci., 2006, 6(1), 70–77 CrossRef CAS PubMed.
- L. F. Cai, B. H. Xian, Z. R. Min, H. R. Wen and Q. Z. Ming, Polymer, 2006, 47(20), 7043–7050 CrossRef CAS PubMed.
- M. Visser, M. B. Lex, M. M. Geraldine, H. W. Mark and B. H. Tamara, JAMA, J. Am. Med. Assoc., 1999, 282(22), 2131–2135 CrossRef CAS.
- P. J. Barnes, Free Radical Biol. Med., 1990, 9(3), 235–243 CrossRef CAS.
- I. L. C. Chapple, J. Clin. Periodontol., 1997, 24(5), 287–296 CrossRef CAS PubMed.
- H. Wiseman and H. Barry, Biochem. J., 1996, 313(1), 17–29 CAS.
- B. Fubini and H. Andrea, Free Radical Biol. Med., 2003, 34(12), 1507–1516 CrossRef CAS.
- Y. Lin, A. H. Berg, P. Iyengar, T. K. Lam, A. Giacca and T. P. Combs, et al., J. Biol. Chem., 2005, 280(6), 4617–4626 CrossRef CAS PubMed.
- A. J. Hoffman, R. C. Elizabeth and R. H. Michael, Environ. Sci. Technol., 1994, 28(5), 776–785 CrossRef CAS PubMed.
- D. Guo, H. Bi, B. Liu, Q. Wu, D. Wang and Y. Cui, Toxicol. in Vitro, 2012, 27(2), 731–738 CrossRef PubMed.
- B. Vozarova, W. Christian, S. L. Robert, E. P. Richard and B. Clifton, et al., Diabetes, 2002, 51(2), 455–461 CrossRef CAS.
- K. J. Tracey, H. E. Wei, K. I. R. K. R. Manogue, Y. U. M. A. N. Fong and D. G. Hesse, et al., J. Exp. Med., 1988, 167(3), 1211–1227 CrossRef CAS.
- B. Y. Kim, J. H. Min and S. C. An, Free Radical Biol. Med., 2001, 30(6), 686–698 CrossRef CAS.
- E. Hatanaka, A. Dermargos, H. A. Armelin, R. Curi and A. Campa, Clin. Exp. Immunol., 2011, 163(3), 362–367 CrossRef CAS PubMed.
- P. Ranjan, A. Vikas, M. B. Peter, W. Kelly, L. J. David and H. H. Nicholas, Antioxid. Redox Signaling, 2006, 8(9–10), 1447–1459 CrossRef CAS PubMed.
- E. Nishio and W. Yasuhiro, Br. J. Pharmacol., 1997, 121(4), 665–670 CrossRef CAS PubMed.
- S. O. Yoon, S. J. Park, S. Y. Yoon, C. H. Yun and A. S. Chung, J. Biol. Chem., 2002, 277, 30271–30282 CrossRef CAS PubMed.
- G. C. Gurtner, S. Werner, Y. Barrandon, L. M. T. Sabine Werner and Y. Barrandon, et al., Nature, 2008, 453(7193), 314–321 CrossRef CAS PubMed.
- G. Taylor, M. S. Lehrer, P. J. Jensen, T. T. Sun and R. M. Lavker, Cell, 2000, 102(4), 451–461 CrossRef CAS.
- H. Yang, K. Johnathan, C. Justin and L. S. James, Sci. Rep., 2011, 1, 176 Search PubMed.
- H. Oshima, R. Ariane, K. Cécile, K. Koji and B. Yann, Cell, 2001, 104(2), 233–245 CrossRef CAS.
- C. C. Lo, M. A. Berta, K. M. Braun, M. Frye, S. Lyle and C. C. Zouboulis, et al., Stem Cells, 2008, 26(5), 1241–1252 CrossRef PubMed.
|
This journal is © The Royal Society of Chemistry 2014 |