DOI:
10.1039/C4RA00971A
(Paper)
RSC Adv., 2014,
4, 24240-24247
Thermo-sensitive zwitterionic block copolymers via ATRP
Received
4th February 2014
, Accepted 12th May 2014
First published on 14th May 2014
Abstract
Novel thermo-sensitive zwitterionic ABC-type triblock copolymers poly(ethylene glycol)-block-[2-(methacryloyloxy)ethyl]dimethyl(3-sulfopropyl)-ammonium hydroxide-block-poly[2-(dimethylamino)ethyl methacrylate] (MPEG-b-PSBMA-b-PDMAEMA) were synthesized by atom transfer radical polymerization (ATRP). The bromide-terminated diblock copolymers poly(ethylene oxide)-block-[2-(methacryloyloxy)ethyl]dimethyl(3-sulfopropyl)-ammonium hydroxide (MPEG-b-PSBMA-Br) were prepared by the ATRP of methacryloxyethyl sulfobetaine initiated by the macroinitiator MPEG-Br, which was obtained by the esterification of poly(ethylene glycol) monomethyl ether (MPEG) with 2-bromoisobutyryl bromide. Then, poly(2-(diethylamino)ethyl methacrylate) (PDMAEMA) was introduced by a sequential ATRP process to obtain the triblock copolymers. These copolymers with varied compositions, molecular weights and polydispersities were characterized by 1H NMR and GPC. Variable temperature ultraviolet analysis was employed to test their stimuli-sensitive properties. These block copolymers exhibited distinct thermo-sensitivity under different molecular compositions or solution conditions. The resistance to the non-specific protein adsorption of the triblock copolymers was evaluated, and excellent antifouling property occurred. This can be attributed to surface hydration via hydrogen bonds between PEG and water molecules and the surface hydration via ionic-induction between PSBMA and water molecules. The synergistic effect resulted in an effective reduction of protein adsorption. These copolymers have potential applications as antifouling and antibacterial agents.
Introduction
Stimuli-responsive or “smart” materials capable of conformational and chemical changes upon response to external signals have attracted significant attention due to their potential applications in various areas such as biotechnology, pharmacology, and materials science.1 Materials capable of resisting long-term biofilm formation in complex media while maintaining nonfouling properties are highly desirable for several applications. Nonfouling coatings are the most commonly used approaches to prevent the attachment and spreading of microorganisms onto surfaces.2 It can be anticipated that the integration of stimuli-responsiveness and nonfouling may endue materials with more features such as separation, purification, encapsulation, controlled release, etc. Especially, the design and fabrication of such materials is promising in biomedicine.
There have been considerable studies on designing and fabricating antifouling materials.3 Copolymers containing non-fouling poly(ethylene glycol) (PEG) or low-fouling poly(hydroxyethyl methacrylate) (PHEMA) segments have been prepared for controlling cell and tissue responses. PEG-based polymers, as one of the most common nonfouling materials, can suppress the accumulation of microorganisms onto surfaces.4 It has been shown that PEG-coated surfaces can delay biofilm formation for 24 h.5 Over the past few years, zwitterionic polymers have received increasing attention in the new generation of nonfouling materials due to their excellent protein resistance.6–24 Recent studies demonstrated that sulfobetaine-containing copolymers can effectively reduce platelet adhesion and protein adsorption.9
Poly(sulfobetaine methacrylate) (PSBMA) with a poly(methacrylate) main chain and an analogue of the taurine betaine pendant group (CH2CH2N+(CH3)2CH2CH2CH2SO3−) has become the most widely studied zwitterionic polymer due to its ease of preparation.19 Sulfobetaines are a special class of zwitterions with ammonium cation and sulfonic anion isolated with an alkyl, usually propyl or butyl, group. One of the characteristic features of these complexes is that they are soluble in water and exhibit an upper critical solution temperature (UCST), which is sensitive to changes in the molecular weight and concentration of the polymer, ionic strength, and species of added salts.25–30 It is expected that zwitterions are capable of binding significant quantities of water, and are potentially excellent candidates for nonfouling materials, which is attributed to their strong capability to form a hydration layer via electrostatic interaction between zwitterions and water molecules. To further develop the zwitterionic-based materials for biomedical applications, smart polymer systems having both controllable biocompatibility and stimuli-responsive functions have been inspired to research. Recently, the physical micellization of synthesized sulfobetaine-containing diblock copolymers with thermo-responsive and zwitterionic properties was reported,31–34 in which the thermo-responsive poly(N-isopropylacryl amide) (PNIPAM) or poly(N,N-diethylacryl amide) (PDEA) with a lower critical solution temperature (LCST) was introduced. These block copolymers were found to exhibit the double thermosensitive phase transition of LCST and UCST behaviors in water. It is worth noting that most of the dual-thermoresponsive phase behaviors derive from the nonionic/zwitterionic block self-assembly in aqueous solution. A recent study on the complex formation of zwitterionic PSBMA with other polyelectrolytes have shown to cause a dramatic increase in viscosity as a result of the formation of a network structure by electrostatic interaction, thereby decreasing the UCST of PSBMA.33a
Poly(2-dimethylaminoethyl methacrylate) (PDMAEMA) is a pH- and temperature-sensitive polymer. It is a weak base with a pKa of about 7.0, and is water-soluble within a wide pH range.35 PDMAEMA exhibits a phase transfer between hydrophilic and hydrophobic characteristics below and above its pKa.36–38 The chains of PDMAEMA are expanded in aqueous solution when the tertiary amine groups of PDMAEMA are protonated and hydrated below the pKa. In contrast, the polymeric chains are collapsed by the deprotonation and dehydration of the amine group above the pKa. PDMAEMA is also a thermoresponsive polymer with an LCST, which is dependent on pH, ionic strength and molecular weight. There are also conformational changes in PDMAEMA below and above its LCST.39–41 PDMAEMA was selected as a candidate polymer for electrografting because it can be modified to polymers with zwitterionic character.42 It has been introduced into biocompatible block copolymers and used as antifouling coatings.43–45 Most recently, Hoogenboom and coworkers reported a triple thermoresponsive schizophrenic diblock copolymer based on PDMAEMA and poly(methoxy oligo-ethyleneglycol methacrylate) (PmOEGMA), which undergoes transitions from conventional micelles via unimers to reverse micelles and finally to a precipitated state upon heating.46
The combination of the LCST of the PDMAEMA with the UCST of the zwitterionic polymers displays an intriguing temperature-induced self-assembly behavior of different types of polymeric aggregates in aqueous solution.47 The objective of this work is to develop novel thermo-sensitive zwitterionic ABC-type triblock copolymers, where A represents a MPEG block, B a PSBMA block, and C a PDMAEMA block, and is schematically shown in Scheme 1. To the best of our knowledge, this is the first example of block copolymers containing zwitterionic PSBMA and nonionic/cationic PDMAEMA polyelectrolyte. In additional, the presence of hydrophilic PEG may stabilize the polymer chains or the formed micelles in aqueous solution. These materials can be very promising for a wide range of applications in medical diagnostics, biomaterials/tissue engineering, and drug delivery.
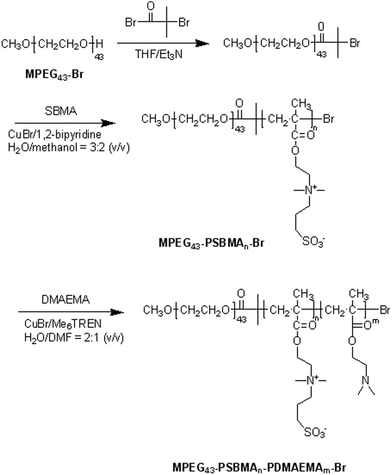 |
| Scheme 1 Synthesis of the thermo-sensitive zwitterionic block copolymers by atrp. | |
Experimental section
Materials
Poly(ethylene oxide) monomethyl ether (MPEG) with number-average molecular weight of 1900 g/mol, was purchased from Fluka. [2-(Methacryloyloxy)ethyl]dimethyl(3-sulfopropyl)ammonium hydroxide (SBMA, 99%), tris[2-(dimethylamino)ethyl]amine (Me6TREN) and 1,2-bipyridine were purchased from Changzhou Yipintang Chemical Company of China. 2-Bromoisobutyryl bromide was purchased from Creator Chemicals of China. 2-(Dimethylamino)ethyl methacrylate (DMAEMA) and 3-chloropropyltriethoxysilane (IPTMOS) were purchased from Aladdin Reagents Co. Ltd. CuBr (99.99%) was purchased from Aldrich and used as received. MPEG-based macroinitiator, denoted as MPEG43-Br, was synthesized according to the literature method.48 1H NMR for MPEG43-Br (CDCl3, 400 MHz): δ = 4.32 (t, 2H, –COOCH2), 3.65–3.58 (m, 158H, –CH2–), 3.38 (s, 3H, –OCH3), 1.94 (s, 6H, –Br(CH3)2).
Diblock copolymer MPEG-b-PSBMA
A typical process for the synthesis of MPEG43-b-PSBMA30-Br is described as follows. The macroinitiator MPEG43-Br (205 mg, 0.1 mmol), SBMA (0.84 g, 3 mmol), CuBr (17 mg, 0.12 mmol), 1,2-bipyridine (15.6 mg, 0.1 mmol), and deionized water–methanol mixture (3
:
2, v/v, 2.0 mL) as solvent were charged to a dry Schlenk tube. After three freeze–pump–thaw cycles to remove oxygen, the tube was sealed and placed in a thermostat oil bath at room temperature for 5 h. The polymerization was then quenched by merging the tube in liquid nitrogen. The product was diluted with ultrapure water and dropped into a dialysis bag with a 3500 Da molecular weight cutoff (MWCO) for removing the remaining monomers and other small molecules. This purification procedure was continued for two days. Then, the water in the dialysis bag was evaporated under reduced pressure, and the collected sample was dried in a vacuum oven for 24 h at room temperature in a 80.6% yield.
Triblock copolymer MPEG-b-PSBMA-b-PDMAEMA
The following procedure considered the synthesis of MPEG43-b-PSBMA30-b-PDMAEMA45 as an example. Me6TREN (28 μL, 0.1 mmol), CuBr (17 mg, 0.12 mmol), MPEG43-b-PSBMA30-Br (0.13 g, 0.012 mmol) as the macroinitiator, 2-(dimethyl-amino)ethyl methacrylate (DMAEMA, 0.3 g, 1.9 mmol) as the monomer, and deionized water–DMF mixture (2
:
1, v/v, 2.0 mL) as solvent were added to a dry Schlenk tube. After three freeze–pump–thaw cycles to remove oxygen, the tube was sealed and placed in preheated oil bath at 90 °C for 6 h. The polymerization was then quenched by immersing the tube in liquid nitrogen. The product was diluted with ultrapure water and dropped into a dialysis bag with 8000 to 14
000 Da MWCO for removing the remaining monomer and other small molecules. This purification procedure was continued for two days. Then, the water in the dialysis bag was evaporated under reduced pressure, and the collected samples were dried in a vacuum oven for 24 h at room temperature in a 45.6% yield.
Characterization
1H NMR spectra were recorded on a Bruker ARX 400M spectrometer (400 MHz) using D2O as the solvent and tetramethylsilane as the internal standard. Molecular weights and polydispersities of the prepared copolymers were determined by aqueous gel permeation chromatography (GPC) using one column of Agilent Technologies 1260 Infinity (molecular weight range from 500 Da to 800 kDa) connected to a Waters 2414 refractive index detector at a 1.0 mL min−1 flow rate and a column temperature of 30 °C and 70 °C. Poly(ethylene oxide) (PEO) standards were used for the calibration.
Transmittance measurements
Stimuli-responsive behavior of the zwitterionic copolymers was determined by turbidity analysis on a Shimadzu UV-1700 spectrophotometer equipped with a thermo-regulator with the heating/cooling rate at 1 °C per 10 min, in which 500 nm wavelength was set for the polymer solution. The UCST or LCST values were determined at 50% transmittance.
Preparation of polymeric coatings
The glass substrates were cut into the size of 1 × 2 cm, and cleaned with piranha solution (30 wt% hydrogen peroxide–98 wt% sulfuric acid, 3
:
7, v/v). After washing by water and ethanol, the glass was dried and heated to 80–100 °C for 1 h, and then cooled at room temperature. The casting solution was prepared by dissolving the triblock copolymer MPEG43-b-PSBMA30-b-PDMAEMA45 (1.2 g) and IPTMOS (2 mL) in absolute methanol (15 mL). Then, the solution was heated at 50 °C for 24 h, and the obtained homogeneous solution was cast on a glass sheet by double-sided, and dried under reduced pressure for 24 h. The reactive IPTMOS was incorporated into the PDMAEMA chains by the sequential quaternization of the tertiary amino groups in PDMAEMA with 3-chloropropyltriethoxysilane, schematically shown in Scheme 2.
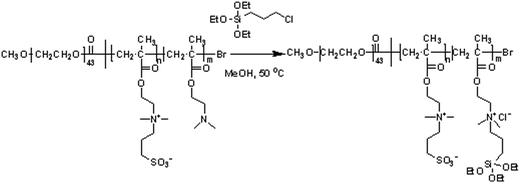 |
| Scheme 2 Incorporation of triethoxysilyl groups into pdmaema. | |
Protein adsorption measurement
Bovine serum albumin (BSA) was used as a model protein to evaluate the protein-resistant characteristics of the prepared polymeric coatings. The coatings with 2 cm2 of external surface area were immersed in ethanol for 10 min, followed by phosphate buffer solution (PBS) to pre-wet the coating surface for 30 min. Each sample was put into a tube with 5 mL of BSA solution of 1 mg mL−1 in PBS. The mixture was incubated at 30 °C for 24 h with a shaking speed of 100 rpm to reach adsorption equilibrium. Scanning electron microscopy (SEM) images were obtained on a Quanta 200 (Philips-FEI, Holland).
Results and discussion
Synthesis and characterization of the block copolymers
ATRP is one of the most powerful polymerization techniques for synthesizing block copolymers.49 The triblock copolymers were prepared by a successive ATRP process, as shown in Scheme 1. The macroinitiator MPEG43-Br with narrow molecular weight distribution of 1.03 was prepared by the esterification of poly(ethylene oxide) monomethyl ether (MPEG) and 2-bromoisobutyryl bromide.48 First, the macroinitiator was used to initiate the ATRP of the monomer SBMA using CuBr/1,2-bipyridine as a catalyst system at room temperature, resulting in the diblock copolymer MPEG-PSBMA-Br. The polymerization degree of SBMA is in accordance with the feed molar ratio of SBMA/MPEG43-Br, indicating the completeness of the ATRP of SBMA initiated by the macroinitiator. The yield of ∼80% can be attributed to the loss of several purifications. Then, the bromine-terminated diblock copolymer was used to initiate the ATRP of DMAEMA using Me6TREN/CuBr as a catalyst system at 90 °C, and the triblock copolymers MPEG-PSBMA-PDMAEMA-Br were obtained with comparatively low yields of ∼40%, which was possibly due to the low initiator activity.
Typical 1H NMR spectra, shown in Fig. 1, confirmed the successful preparation of the diblock and triblock copolymers. The number-average compositions of the copolymers were calculated by comparing the relative intensities of the signal for –OCH2CH2– protons of the PEG block (3.6 ppm), for –NCH3+– protons of the SBMA block (3.2 ppm) and for –OCH2– protons of the DMAEMA block (4.1 ppm). Aqueous GPC curves of the typical diblock and triblock copolymers are displayed in Fig. 2. The molecular weight distributions of the synthesized copolymers from GPC are shown in Table 1. The polydispersities are relatively low (PDI < 1.5), which indicates that these polymerizations were living and controllable.
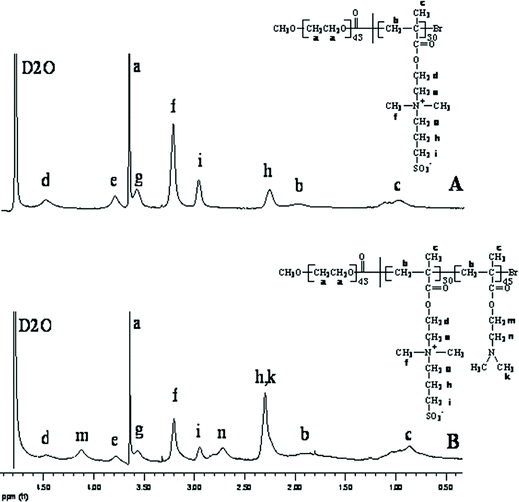 |
| Fig. 1 1 h NMR spectra of the diblock copolymer MPEG43-b-PSBMA30 (a) and the triblock copolymer MPEG43-b-PSBMA30-b-PDMAEMA45 (b). | |
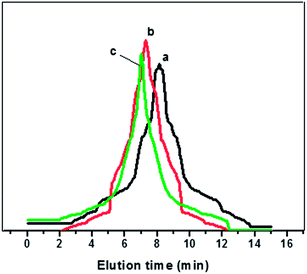 |
| Fig. 2 GPC curves of MPEG43-PSBMA30 (a), MPEG43-PSBMA30-PDMAEMA45 (b), and MPEG43-PSBMA30-PDMAEMA90 (c). | |
Table 1 Characteristics of the macroinitiator and the block copolymers
Samplea |
Yield (%) |
Mn,NMRb |
Mn,GPCc |
Mw/Mnd |
Molecular structure determined by NMR. Number-average molecular weight derived from 1H NMR. Number-average molecular weight measured by GPC. Molecular weight distribution measured by GPC. |
MPEG43-Br |
90.5 |
2050 |
2450 |
1.03 |
MPEG43-PSBMA30 |
80.6 |
10900 |
11100 |
1.37 |
MPEG43-PSBMA40 |
82.0 |
13900 |
12030 |
1.41 |
MPEG43-PSBMA50 |
70.5 |
16900 |
15250 |
1.28 |
MPEG43-PSBMA30-PDMAEMA45 |
46.5 |
18000 |
20150 |
1.23 |
MPEG43-PSBMA30-PDMAEMA90 |
41.1 |
25100 |
26700 |
1.08 |
Temperature-sensitive properties of the diblock copolymers
The homopolymer PSBMA at a concentration of 0.5 mg mL−1 in an aqueous solution exhibited an UCST between 16 and 18 °C, which decreases with the decreasing molecular weight of PSBMA.34b In the diblock copolymers, the hydrophilic PEG blocks endow stability to the polymer chains or the formed micelles in aqueous solution, resulting in an increase of UCST. Above the UCST of PSBMA block, the diblock copolymers are soluble in water at room temperature because of the electrostatic repulsion between the polymer intrachains and interchains. However, below the UCST the core–shell micelles form with a core of insoluble PSBMA association and a soluble shell, and consequently the precipitation occurs. This is attributed to the contribution of the level of interaction of mutual electrostatic attraction by ion pairings between ammonium cation and sulfoanion of the zwitterionic sulfobetaine groups.34b
In this case, the effect of the solution concentration and the polymerization degree of PSBMA was mainly focused. Consider MPEG43-b-PSBMA50 as an example. The copolymer was dissolved in ultrapure water with varied concentration. The phase transition temperatures were measured using a UV-vis spectrophotometer coupled with a temperature controller to heat or cool at 1 °C per 10 min from 10–50 °C. As shown in Fig. 2A, the transmittance of the polymer solution increased with increasing temperature. The corresponding temperature at 50% transmittance was defined as the upper critical solution temperature (UCST) of the solution. The UCST increased with an increase of the polymer solution concentration, and was determined to be 22, 21, and 15 °C for at concentrations of 5, 10, and 20 mg mL−1, respectively. The diblock copolymers with varying polymerization degree of PSBMA (n = 30, 40, or 50) were dissolved in ultrapure water at an identical concentration of 5 mg mL−1. The transmittance of the polymer solution upon heating and cooling at varied temperatures between 10–36 °C is illustrated in Fig. 2B. The UCST increased with the increasing polymerization degree of PSBMA, and was 11, 17, and 21 °C for n = 30, 40, and 50, respectively.
A possible explanation can be proposed as follows. In aqueous solution, a higher polymer concentration or polymerization degree of PSBMA exists; a higher charge density is generated, which enhances electrostatic repulsion and attraction. Micelle formation and aggregation can be easily realized, resulting in a higher USCT.
Temperature-sensitive properties of the triblock copolymers
Chang et al. reported doubly thermo-responsive copolymers comprising PSBMA and PNIPAM blocks. These copolymers exhibited an individual UCST and LCST.34 In our case, the coexistence of PSBMA and PDMAEMA blocks via ATRP can be expected to display intriguing temperature-induced behavior in aqueous solutions. Nonionic PDMAEMA exhibited an LCST in water between 64 and 68 °C. Above the LCST, PDMAEMA chains become hydrophobic and the hydrogen bonding with water molecules weakens. The precipitation of PDMAEMA derives from nonionic DMAEMA segment associations in water. The origin of the phase behavior of PDMAEMA arises from the release of ordering water molecules and the formation of intrachain hydrophobic interactions associated with the side chain isopropyl moieties when the temperature increases above a critical point. Below the UCST, PSBMA exists as a collapsed coil and precipitates with the zwitterionic associations in aqueous solution.
MPEG43-b-PSBMA30-b-PDMAEMA45 and MPEG43-b-PSBMA30-b-PDMAEMA90 dissolved in ultrapure water at a concentration of 5 mg mL−1 and 10 mg mL−1, respectively. The temperature dependence of transmittance between 28 and 68 °C is shown in Fig. 3. For MPEG43-b-PSBMA30-b-PDMAEMA45, the UCST decreased from 62 to 52 °C with an increase of the polymer concentration from 5 to 10 mg mL−1. A similar tendency was observed for MPEG43-b-PSBMA30-b-PDMAEMA90. At a concentration of 5 mg mL−1, the UCST of MPEG43-b-PSBMA30-b-PDMAEMA45 was higher than that of MPEG43-b-PSBMA30-b-PDMAEMA90 (50 °C), and a similar result was observed at a concentration of 10 mg mL−1. It is worth noting that the LCST did not occur because of the restriction of the aqueous media and the thermo-regulator.
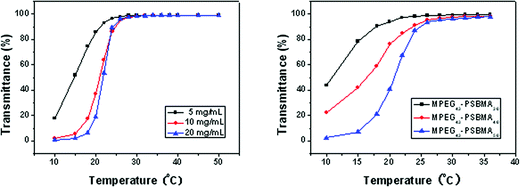 |
| Fig. 3 Temperature dependence of transmittance for MPEG43-b-PSBMA50 with different concentration in aqueous solutions (a) and for MPEG43-b-PSBMAn (n = 30, 40, or 50) at a concentration of 5 mg mL−1 in aqueous solutions (b). | |
The effects of pH on the UCSTs and LCSTs of the triblock copolymer solutions were further evaluated to investigate the solubility characteristics at a fixed polymer concentration of 10 mg mL−1. The pH of the aqueous medium was adjusted by phosphate buffer solution (PBS) at 4.0, 6.8, and 9.2, respectively. Temperature dependence of transmittance is shown in Fig. 4. The transmittance of the polymer solution increased with increasing temperature at pH values of 4.0 and 6.8. For MPEG43-b-PSBMA30-b-PDMAEMA45 (Fig. 5A), the UCST at pH 4.0 (52 °C) was much higher than at pH 6.8 (35 °C). However, at pH 9.2, a sharp change in transmittance at 40 °C demonstrated that both PSBMA and PDMAEMA blocks were soluble, exhibiting individual UCST (34 °C) and LCST (42 °C). For MPEG43-b-PSBMA30-b-PDMAEMA90 (Fig. 5B), the UCST at pH 4.0 (46 °C) was also higher than at pH 6.8 (38 °C). However, at pH 9.2, a sharp change in transmittance occurred at 38 °C.
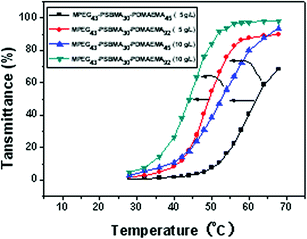 |
| Fig. 4 Temperature dependence of transmittance for MPEG43-b-PSBMA30-b-PDMAEMA45 and MPEG43-b-PSBMA30-b-PDMAEMA90 with different concentration in aqueous solutions. | |
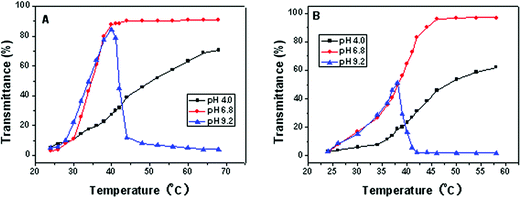 |
| Fig. 5 Temperature dependence of transmittance of MPEG43-b-PSBMA30-b-PDMAEMA45 (a) and MPEG43-b-psbma30-b-PDMAEMA90 (b) at a concentration of 10 mg mL−1 in aqueous solutions under different pH values. | |
PDMAEMA shows pH-responsive behavior due to the protonation of the tertiary amine group. The higher the protonation degree of PDMAEMA units, the better the solubility of PDMAEMA chains in water at low pH since pKa of PDMAEMA is 7.0. Thus, the steric hindrance of stretching PDMAEMA chains would prevent PEG-b-PSBMA-b-DMAEMA molecules from inter-molecular aggregation to form large micelles. In this case, PEG-b-PSBMA-b-DMAEMA molecules tend to form unimer micelles by the intramolecular collapse of PSBMA chains. When pH increases to 7.0, PDMAEMA becomes partially deprotonated and less hydrophilic. At pH 9.2, PDMAEMA chains become highly deprotonated. Thus, the increase of block lengths of PSBMA or PDMAEMA contributes to the formation of copolymers in soluble unimer states, resulting in the expansion of the intermediate temperature range (i.e., above the UCST and below the LCST). In the intermediate temperature range, copolymers were observed to exist as soluble unimers in water. However, in the solution temperature range below UCST or above LCST, morphological changes of PSBMA-b-PDMAEMA copolymers might preferentially expose one polymer block extended in the solution phase, while the other is buried inside micelle cores, resulting in precipitation as collapsed associations in water. The doubly thermoresponsive transition behavior of the prepared copolymer MPEG-b-PSBMA-b-PDMAEMA results from the switchable nonionic/zwitterionic block self-assembly driven by the formation of inter and intramolecular electrostatic interactions by SBMA segments of zwitterionic sulfobetaine groups and intramolecular hydrophobic interactions by DMAEMA segments of nonionic isopropyl groups, as illustrated in Scheme 3.
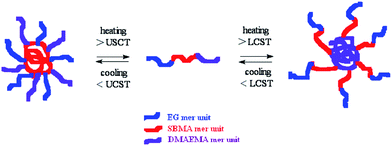 |
| Scheme 3 Simplified model of temperature dependence of the polymer conformation in aqueous solution. | |
Resistance to protein adsorption of the triblock copolymers
The extent of protein adsorption is mainly determined by the delicate balance of the interaction between protein molecules and membrane surface. The factors affecting protein adsorption include hydrophilicity/hydrophobicity balance, surface change, and surface roughness. It is believed that PEG uses a “steric repulsion” and a hydration layer via hydrogen bonding around the molecular chain to prevent nonspecific protein adsorption.50 PEG is usually used as the hydrophilic component in the polymers since it has been recognized as a material uniquely suited for preventing protein adsorption. It is generally accepted that the repulsive force between PEG and protein are created by favorable H2O–PEG interaction and the mobility of the PEG chains in aqueous solution. The hydrophilicity and unique coordination with the surrounding water molecules of PEG facilitate the resistance to protein adsorption.51 PSBMA can bind to water molecules even more strongly via electrostatically induce hydration, such sulfobetaine-based polymers or surfaces have been shown to greatly reduce nonspecific protein adsorption.52 The quaternized PMDAEMA has antibacterial activity.53
Fig. 6 shows the results of BSA adsorption on the surface of a sheet glass and the polymeric coating of the quaternized MPEG43-b-PSBMA30-b-PDMAEMA45. In contrast with the sheet glass surface, the polymeric coating of the quaternized MPEG43-b-PSBMA30-b-PDMAEMA45 has a relatively low amount of BSA adsorption. The effective reduction in protein adsorption can be attributed to the SBMA segments, exhibiting antifouling character and the PEG segments that stretch out from the coating surface into the surrounding aqueous environment.
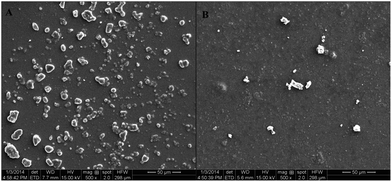 |
| Fig. 6 SEM images of BSA adsorption on the surface of a sheet glass (a) and on the polymeric coating of MPEG43-PSBMA30-PDMAEMA45 (b). | |
Conclusions
In summary, temperature-sensitive zwitterionic triblock copolymers have been successfully synthesized via atom transfer radical polymerization, following the idea that the conformation of MPEG-b-PSBMA and MPEG-b-PSBMA-b-PDMAEMA block copolymers in aqueous solution could change in response to temperature. A thermo-responsive morphological transition occurs from zwitterionic PSBMA-core micelle to unimer to nonionic PDMAEMA-core micelle when the solution temperature increases, and the process is reversible when the solution temperature decreases. The coverage of PEG on the polymeric coating effectively prohibits protein adsorption and cell adhesion. This dual thermo-responsive behavior provides the core–shell micelles promising potential in addressing related theoretical and practical problems such as drug delivery. This study also suggests that the quaternized zwitterionic MPEG-b-PSBMA-b-PDMAEMA copolymer is a novel candidate for the preparation of antifouling coatings and antifouling membranes with antibacterial activity.
Acknowledgements
The authors gratefully acknowledge financial support from the Shandong Provincial Natural Science Foundation of China (Grant no. ZR2010BM006) and Shandong Provincial Overseas Visiting Scholar Project of China (2011).
Notes and references
-
(a) P. Matteini, M. R. Martina, G. Giambastiani, F. Tatini, R. Cascella and R. Pini, J. Mater. Chem. B, 2013, 1, 1096 RSC;
(b) P. Schattling, F. D. Jochum and P. Theato, Polym. Chem., 2014, 5, 25 RSC;
(c) W. Tian, X. Wei, Y. Liu and X. Fan, Polym. Chem., 2013, 4, 2850 RSC;
(d) X. Jiang, G. Lu, C. Feng, Y. Li and X. Huang, Polym. Chem., 2013, 4, 3876 RSC.
- G. Cheng, G. Li, H. Xue, S. Chen, J. D. Bryers and S. Jiang, Biomaterials, 2009, 30, 5234 CrossRef CAS PubMed.
- Z. Zhao, H. Ni, Z. Han, T. Jiang, Y. Xu, X. Lu and P. Ye, ACS Appl. Mater. Interfaces, 2013, 5, 7808 CAS.
-
(a) L. Song, J. Zhao, S. Luan, J. Ma, J. Liu, X. Xu and J. Yin, ACS Appl. Mater. Interfaces, 2013, 5, 13207 CrossRef CAS PubMed;
(b) D. G. Kim, H. Kang, S. Han and J. C. Lee, J. Mater. Chem., 2012, 22, 6854 Search PubMed.
-
(a) A. Roosjen, H. C. van der Mei, H. J. Busscher and W. Norde, Langmuir, 2004, 20, 10949 CrossRef CAS PubMed;
(b) M. R. Nejadnik, H. C. van der Mei, W. Norde and H. J. Busscher, Biomaterials, 2008, 29, 4117 CrossRef CAS PubMed;
(c) V. E. Wagner, J. T. Koberstein and J. D. Bryers, Biomaterials, 2004, 25, 2247 CrossRef CAS PubMed.
- Y. Chang, S. Chen, Z. Zhang and S. Jiang, Langmuir, 2006, 22, 2222 CrossRef CAS PubMed.
- Z. Zhang, J. A. Finlay, L. F. Wang, Y. Gao, J. A. Callow, M. E. Callow and S. Jiang, Langmuir, 2009, 25, 13516 CrossRef CAS PubMed.
- P. Kasák, Z. Kroneková, I. Krupa and I. Lacík, Polymer, 2011, 52, 3011 CrossRef PubMed.
- H. Kitano, S. Tada, T. Mori, K. Takaha, M. Gemmei-Ide, M. Tanaka, M. Fukuda and Y. Yokoyama, Langmuir, 2005, 21, 11932 CrossRef CAS PubMed.
- S. Chen, J. Zheng, L. Li and S. Jiang, J. Am. Chem. Soc., 2005, 127, 14473 CrossRef CAS PubMed.
- W. Feng, J. L. Brash and S. Zhu, Biomaterials, 2006, 27, 847 CrossRef CAS PubMed.
- Z. Zhang, S. Chen, Y. Chang and S. Jiang, J. Phys. Chem. B, 2006, 110, 10799 CrossRef CAS PubMed.
- S. Chen and S. Jiang, Adv. Mater., 2008, 20, 335 CrossRef CAS.
- Z. Zhang, H. Vaisocherova, G. Cheng, W. Yang, H. Xue and S. Jiang, Biomacromolecules, 2008, 9, 2686 CrossRef CAS PubMed.
- K. Ishihara, T. Ueda and N. Nakabayashi, Polym. J., 1990, 22, 355 CrossRef CAS.
- Y. Iwasaki and K. Ishihara, Anal. Bioanal. Chem., 2005, 381, 534 CrossRef CAS PubMed.
- S. Yusa, K. Fukuda, T. Yamamoto, K. Ishihara and Y. Morishima, Biomacromolecules, 2005, 6, 663 CrossRef CAS PubMed.
- B. Yu, A. B. Lowe and K. Ishihara, Biomacromolecules, 2009, 10, 950 CrossRef CAS PubMed.
- E. Gizeli and C. R. Lowe, Biomolecular sensors, CRC Press, Boca Raton, 1st edn, 2002 Search PubMed.
- S. Jiang and Z. Cao, Adv. Mater., 2010, 22, 920 CrossRef CAS PubMed.
- Z. Zhang, S. Chen and S. Jiang, Biomacromolecules, 2006, 7, 3311 CrossRef CAS PubMed.
- Z. Zhang, T. Chao, S. Chen and S. Jiang, Langmuir, 2006, 22, 10072 CrossRef CAS PubMed.
- J. Ladd, Z. Zhang, S. Chen, J. C. Hower and S. Jiang, Biomacromolecules, 2008, 9, 1357 CrossRef CAS PubMed.
- N. D. Brault, H. S. Sundaram, Y. Li, C. Huang, Q. Yu and S. Jiang, Biomacromolecules, 2012, 13, 589 CrossRef CAS PubMed.
- A. B. Lowe, Chem. Rev., 2002, 102, 4177 CrossRef CAS PubMed.
- J. H. Li, M. Z. Li, J. Miao, J. B. Wang, X. S. Shao and Q. Q. Zhang, Appl. Surf. Sci., 2012, 258, 6398 CrossRef CAS PubMed.
- Y. Chang, W. J. Chang, Y. J. Shih, T. C. Wei and G. H. Hsiue, ACS Appl. Mater. Interfaces, 2011, 3, 1228 CAS.
-
(a) M. Tian, J. Wang, E. Zhang, J. Li, C. Duan and F. Yao, Langmuir, 2013, 29, 8076 CrossRef CAS PubMed;
(b) G. Chang, Z. Zhang, S. Chen, J. D. Bryers and S. Jiang, Biomaterials, 2007, 28, 4192 CrossRef PubMed.
- L. Chen, Y. Honma, T. Mizutani, D. J. Liaw, J. Gong and Y. Osada, Polymer, 2000, 41, 141 CrossRef CAS.
- D. N. Schulz, D. G. Peiffer, P. K. Larabee, J. J. Kaladas, L. Soni, B. Handwerker and R. T. Garner, Polymer, 1986, 27, 1734 CrossRef CAS.
-
(a) M. Arotcarena, B. Heise, S. Ishaya and A. J. Laschewsky, J. Am. Chem. Soc., 2002, 124, 3787 CrossRef CAS PubMed;
(b) J. Virtanen, M. Arotcarena, B. Heise, S. Ishaya, A. Laschewsky and H. Tenhu, Langmuir, 2002, 18, 5360 CrossRef CAS.
- Y. Maeda, H. Mochiduki and I. Ikeda, Macromol. Rapid Commun., 2004, 25, 1330 CrossRef CAS.
-
(a) K. Okawa, J. Gong and Y. Osada, Macromol. Rapid Commun., 2002, 23, 423 CrossRef CAS;
(b) J. Yin, J. Hu, G. Zhang and S. Liu, Langmuir, 2014, 30, 2551 CrossRef CAS PubMed.
-
(a) Y. Chang, W. Yandi, W. Y. Chen, Y. J. Shih, C. C. Yang, Y. Chang, Q. D. Ling and A. Higuchi, Biomacromolecules, 2010, 11, 1101 CrossRef CAS PubMed;
(b) Y. J. Shih, Y. Chang, A. Deratani and D. Quemener, Biomacromolecules, 2012, 13, 2849 CrossRef CAS PubMed;
(c) Y. Chang, W. Y. Chen, W. Yandi, Y. J. Shih, W. L. Chu, Y. L. Liu, C. W. Chu, R. C. Ruaan and A. Higuchi, Biomacromolecules, 2009, 10, 2092 CrossRef CAS PubMed.
-
(a) Y. Xu, S. Bolisetty, M. Drechsler, B. Fang, Y. Yuan and M. Ballauff, Polymer, 2008, 49, 3957 CrossRef CAS PubMed;
(b) N. Karanikolopoulos, M. Zamurovic, M. Pitsikalis and N. Hadjichristidis, Biomacromolecules, 2010, 11, 430 CrossRef CAS PubMed.
- O. Schepelina and I. Zharov, Langmuir, 2008, 24, 14188 CrossRef CAS PubMed.
- N. Nordgren and M. W. Rutland, Nano Lett., 2009, 9, 2984 CrossRef CAS PubMed.
- J. I. Amalvy, E. J. Wanless, Y. Li, V. Michailidou and S. P. Armes, Langmuir, 2004, 20, 8992 CrossRef CAS PubMed.
- S. H. Cho, M. S. Jhon, S. H. Yuk and H. B. Lee, J. Polym. Sci., Part B: Polym. Phys., 1997, 35, 595 CrossRef CAS.
- D. Fournier, R. Hoogenboom, H. M. L. Thijs, R. M. Paulus and U. S. Schubert, Macromolecules, 2007, 40, 915 CrossRef CAS.
-
(a) F. A. Plamper, M. Ruppel, A. Schmalz, O. Borisov, M. Ballauff and A. H. E. Müller, Macromolecules, 2007, 40, 8361 CrossRef CAS;
(b) H. Murata, C. S. Cummings, R. R. Koepsel and A. J. Russell, Biomacromolecules, 2013, 14, 1919 CrossRef CAS PubMed.
- S. West, J. P. Salvage, E. J. Lobb, S. P. Armes, N. C. Billingham, A. L. Lewis, G. W. Hanlon and A. W. Lloyd, Biomaterials, 2004, 25, 1195 CrossRef CAS PubMed.
- M. K. Park, J. H. Youk, S. Pispas, N. Hadjichristidis and R. Advincula, Langmuir, 2002, 18, 8040 CrossRef CAS.
- D. A. Barrett, M. S. Hartshorne, M. A. Hussain, P. N. Shaw and M. C. Davies, Anal. Chem., 2001, 73, 5232 CrossRef CAS.
- W. K. Cho, B. Kong and I. S. Choi, Langmuir, 2007, 23, 5678 CrossRef CAS PubMed.
- Q. Zhang, J. D. Hong and R. Hoogenboom, Polym. Chem., 2013, 4, 4322 RSC.
- C. M. Schilli, M. F. Zhang, E. Rizzardo, S. H. Thang, Y. K. Chong, K. Edwards, G. Karlsson and A. H. E. Muller, Macromolecules, 2004, 37, 7861 CrossRef CAS.
- K. Jankova, X. Chen, J. Kops and W. Batsberg, Macromolecules, 1998, 31, 538 CrossRef CAS.
-
(a) K. Matyjaszewski and J. Xia, Chem. Rev., 2001, 101, 2921 CrossRef CAS PubMed;
(b) M. Kamigaito, T. Ando and M. Sawamoto, Chem. Rev., 2001, 101, 3689 CrossRef CAS PubMed;
(c) X. D. Tang, X. C. Liang, L. C. Gao, Z. H. Shen, X. H. Fan and Q. F. Zhou, J. Polym. Sci., Part A: Polym. Chem., 2010, 48, 2564 CrossRef CAS.
- L. Y. Li, S. F. Chen, J. Zhang, B. D. Ratner and S. Y. Jiang, J. Phys. Chem. B, 2005, 109, 2934 CrossRef CAS PubMed.
- D. G. Kim, H. Kang, S. Han and J. C. Lee, J. Mater. Chem., 2012, 22, 8654 RSC.
- S. F. Chen, F. C. Yu, Q. Yu, Y. He and S. Y. Jiang, Langmuir, 2006, 21, 8186 CrossRef PubMed.
- H. J. Huang, H. Murata, R. R. Koepsel, A. J. Russell and K. Matyjaszewski, Biomacromolecules, 2007, 8, 1396 CrossRef PubMed.
|
This journal is © The Royal Society of Chemistry 2014 |