DOI:
10.1039/C3RA47945E
(Review Article)
RSC Adv., 2014,
4, 11907-11918
Organoantimony and organobismuth complexes for CO2 fixation
Received
24th December 2013
, Accepted 14th February 2014
First published on 18th February 2014
Abstract
The utilization of organoantimony (bismuth) complexes in CO2 fixation is reviewed in this article. The efficient synthesis of cyclic carbonates from CO2 and epoxides over an organoantimony(V) catalyst was first reported in 1979. After that, several organoantimony(V) complexes were found to be active for CO2 fixation. In 2009, an organoantimony(III) complex was reported as an effective CO2 absorbent and it is the only example of this kind. The study of organobismuth complexes for CO2 fixation started in 2008, and the achievements are: (i) when organobismuth oxides, hydroxides and methoxides are used as CO2 absorbents, they are converted to carbonates, and the organobismuth carbonates can be renewed as organobismuth oxides; (ii) organobismuth complexes can be used as catalysts to transform CO2 into epoxides as cyclic carbonates at room temperature in the presence of a co-catalyst such as LiI and Bu4NI; (iii) there is the development of bimetallic organobismuth complexes that show cooperative catalytic action; (iv) there is the physical fixation of CO2 by inorganic–organic bismuth complexes; and (v) CO2 insertion into the Bi–C bond of an organobismuth complex is disclosed. Most of the above catalytic systems can be considered as “electrophile–nucleophile”. In general, the synthesis of cyclic carbonates from CO2 and epoxides catalyzed by an organoantimony (bismuth) complex follows the mechanism: the complex first reacts with CO2 to form an organometallic carbonate, then the carbonate reacts with an epoxide with ring-opening by a base (e.g., n-Bu4NI).
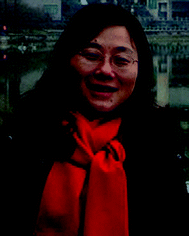 Yi Chen | Yi Chen was born in Hunan Province, China. She received her B.Sc. degree from Hunan Normal University in 1996, and received a Master's Degree from Hunan University of Chinese Medicine in 2003. From 1996 to present, she worked as a lecturer and later associate professor (2006) in Hunan University of Chinese Medicine. At present, she is a visiting researcher with Prof. S.F. Yin. Her main research interests include basic research of the cardiovascular and cerebrovascular diseases and organbismuth medicine. |
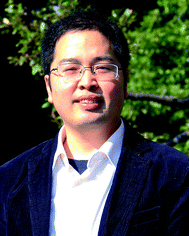 Renhua Qiu | Renhua Qiu was born in Hunan Province, China. He received a B.Sc. degree from Hunan Normal University in 2004, and received a Ph.D. from Hunan University in 2011 under the co-supervision of Professors Shuang-Feng Yin and Xinhua Xu. He was a research assistant (2010) with Prof. C.-T. Au in Hong Kong Baptist University and a visiting researcher (2011) with Dr L.-B. Han in AIST (Japan). From 2009 to present, he worked as an assistant researcher and later assistant professor (2011) in Hunan University. At present, he is visiting Osaka University (Japan) as a JSPS fellow (2012) with Prof. N. Kambe. Dr Qiu's main research interests include organometallic chemistry, Lewis acid catalysis, and C–H bond activation. |
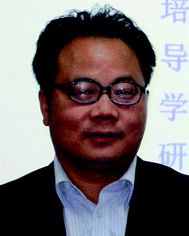 Xinhua Xu | Xinhua Xu was born in Hunan Province, China. He received his Master's Degree from Hunan Normal University in 1991, and received a Ph.D. from Zhejiang University with Prof. Xian Huang in 1998. He did postdoctoral research in Nankai University from 1998 to 2000 with Prof. Ruyu Chen. From 2000 to present, he worked as an Associate professor and later full professor (2006) in Hunan University. In 2002, he visited Japan as a visiting researcher with Prof. J. Otera. Prof. Xu's main research interests include organometallic chemistry, heteroatom chemistry, and homogeneous catalysis. |
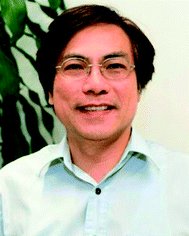 Chak-Tong Au | Chak-Tong Au was born in Hong Kong. In 1981, he received a Ph.D. from the University of Bradford, UK. From 1980 to 1986, he did research at the University College, Cardiff, Wales. In 1986, he joined Xiamen University, China as an associate professor and was promoted to professor in 1987. From 1990 to present, he worked as a lecturer and later full professor in Hong Kong Baptist University. He was awarded a D.Sc. degree by the University of Liverpool in 2003. Prof. Au has published over 400 papers in areas of heterogeneous catalysis and novel materials. At present, he serves as editor of Appl. Catal., A. |
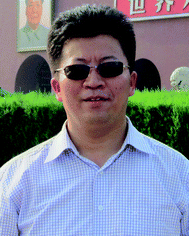 Shuang-Feng Yin | Shuang-Feng Yin was born in Hunan Province, China. He received a Ph.D. from Tsinghua University in 2003. He did postdoctoral research with Prof. C.-T. Au from 2002 to 2004 in HKBU. From 2004 to present, he worked as a lecturer and later full professor (2006) in Hunan University. From 2004 to 2006, he visited Japan as a JSPS fellow with Dr S. Shimada. Prof. Yin's main research interests include organometallic chemistry, CO2 chemistry, catalysis, new energy, nanomaterials. |
1. Introduction
1.1 CO2 challenge and opportunity
Atmospheric CO2 is at its highest level ever recorded, currently amounting to around 390 ppm by volume (590 ppm by mass).1 The absolute mass of atmospheric CO2 amounts to an alarming value of 3 × 1015 tons. It is estimated that human activities currently produce an additional 1.3 × 1010 tons of CO2 per year. The impacts of further increase of atmospheric CO2 on world climate and oceans could be severe as well as uncontrollable. Nonetheless, despite the notorious image, CO2 can be the ultimate C1 feedstock. As far as the chemical fixation of CO2 is concerned, there are significant developments in the past decades. It appears that the capture of CO2 at source (e.g., at the power plants that use coal as fuel) is a suitable strategy for the mitigation of greenhouse effect.2–5 For such a goal, effective substrates are needed as absorbents. It is of economic significance if valuable chemicals such as cyclic carbonates can be synthesized in the course of fixing CO2.6–14
The CO2 molecule is linear, showing C–O distances of 1.16 Å. Although overall nonpolar, CO2 contains polar bonds due to the difference in electronegativity between C and O. Its electronic structure is best represented as O−δ–C+2δ–O−δ, disclosing susceptibility to nucleophilic attack at the carbon and electrophilic attack at the oxygen atoms. Reactions of CO2 in biological systems tend to be a combination of nucleophilic and electrophilic interactions. Without the combined interactions, powerful electrophiles or nucleophiles are needed to initiate a CO2 reaction. With an ionization potential of 13.78 eV, CO2 is nonbasic and interacts only weakly with Brønsted and Lewis acids. It is considered that the major obstacle preventing efficient conversion of CO2 into useful chemicals is the lack of appropriate catalysts.18–27
1.3 CO2 fixation with organometallic complexes
Recently, there are reports on the use of transition metal (e.g., Ni,28–31 Rh,32 Ir, Fe, Cu, Re, and Co) complexes,33–40 for CO2 transformation. Potentially, the use of them could result in better utilization of CO2 in chemical industry as well as having certain existing processes replaced by ones that are more energy-benign. In real practice, these complexes are not applicable because under mild conditions they do not perform satisfactorily when CO2 association or dissociation is a matter of concern. For the activation of CO2 with homogeneous molecular catalysts, there are three major mechanistic steps: (i) CO2 coordination with the metal or ligand of catalyst, (ii) CO2 reaction with neighboring ligands, and (iii) release of product and the restoration of catalyst.41–45
1.4 Organoantimony and organobismuth complexes for CO2 fixation
Organobismuth and organoantimony complexes have been investigated for chemical fixation of CO2 since 1979. Bismuth and antimony are group 15 elements. The former is known to be nontoxic and noncarcinogenic while the latter is a boundary element showing metal as well as non-metal features. The compounds of both metals are studied because of their versatile roles (acting as reaction reagents, oxidizing agents, catalysts, and medicine).46–57 In the past decade, there have been researches on novel cationic organobismuth and organoantimony complexes. We present herein representative results of using this kind of material as absorbents for CO2 capture and/or as catalysts for cycloaddition of CO2 to epoxides.
2. CO2 fixation using organoantimony complexes
2.1 Organoantimony(V) complexes
The chemical fixation of CO2 using organoantimony complexes as catalysts was first reported by Matsuda et al. in 1979.58 They used pentavalent organoantimony compounds to catalyze the reaction between CO2 and a number of epoxides (Table 1).59 In all cases, the corresponding cyclic carbonates are generated in almost quantitative amounts.
Table 1 Reaction of CO2 with epoxides catalyzed by pentavalent organoantimony compoundsa,59
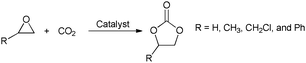
|
Catalysts |
Epoxides (R) |
Temp. (°C) |
Time (min) |
Yieldb (%) |
Reaction conditions: epoxides, 0.1 mol; CO2, 0.2 mol (50 kg cm−2); catalysts, 0.001 mol. Based on epoxides. Catalysts were recovered. Trace of poly(styrene oxide) was obtained besides the carbonate. Poly(styrene oxide) was obtained in ca. 10% yield besides the carbonate. |
Ph5Sb (1a) |
H |
120 |
330 |
82 |
CH3 |
120 |
130 |
87 |
Ph4SbBr (1b) |
H |
120 |
12 |
96 |
CH3 |
120 |
8 |
92c |
100 |
20 |
98c |
60 |
120 |
93c |
CH2Cl |
120 |
24 |
97 |
Ph |
120 |
16 |
91d |
Ph3SbBr2 (1c) |
H |
120 |
38 |
95 |
CH3 |
120 |
16 |
97c |
100 |
50 |
92c |
60 |
390 |
97c |
CH2Cl |
120 |
80 |
94 |
Ph |
120 |
24 |
82e |
Ph3SbCl2 (1d) |
H |
120 |
180 |
99 |
100 |
480 |
96 |
CH3 |
120 |
150 |
96c |
Me3SbBr2 (1e) |
H |
120 |
15 |
72 |
100 |
40 |
79 |
CH3 |
120 |
60 |
94c |
100 |
150 |
91c |
Ph3SbO (1f) |
CH3 |
120 |
120 |
94 |
Ph3Sb (1g) |
CH3 |
120 |
360 |
7 |
Ph2SbCl (1h) |
CH3 |
120 |
360 |
5 |
SbCl3 (1i) |
CH3 |
120 |
360 |
4 |
It was observed that the catalysts with halogen atoms and phenyl substituents are more active than those without halogen atoms and with methyl substituent. The complexes halogenated with bromine are more active than those with chlorine. The catalytic activities of the complexes decrease in the order of 1b > 1d > 1e > 1a, 1c, 1f ≫ the trivalent compounds (1g, 1h, 1i). The catalysts 1b–e can be recovered from the crude products in good yields. It was reported that catalysts 1a and 1f cannot be recovered, and the corresponding crude products are light brown and light yellow in color. Such phenomena could be a result of catalyst instability. As reflected by the time of reaction, the epoxides (R) show reactivity that follows the order of CH3 > Ph > H > CH2Cl.
Using tetraphenyistibonium halides (Ph4SbCI and Ph4SbBr) as catalysts for the hydration of ethylene oxide in the presence of CO2 or ethylene carbonate at 120 °C, Matsuda et al. gained better control of product distribution.60 With a small excess of water in the reaction system, ethylene glycol is generated as the predominant product. When the molar ratio of water to ethylene oxide is 1 or less than 1, the stibonium halides selectively give diethylene glycol as the major product.
As proposed by Matsuda et al., the catalytic mechanism can be described as shown in Scheme 1. Basically, there is the coordination of epoxide with the Sb atom followed by interaction with CO2.59 In the reaction with styrene oxide, polystyrene oxide is formed as by-product (up to a yield of ca. 10%). It is noted that the catalytic activities of organoantimony compounds are superior to those of organotin compounds despite the Lewis acid strength of Sn being stronger than that of Sb.59 In the case of organoantimony complexes, effects such as reaction acceleration due to preheating and polymerization of epoxides as inferred by epoxide–antimony coordination are not observed. In addition, there is no obvious change of IR and 1H NMR spectra upon the mixing of epoxides with the Sb catalysts (1 mol% of catalyst in epoxide solution). All the facts point to the absence of epoxide–zinc coordination. It is hence reasonable to consider that the activation of CO2 follows the cyclic mechanism shown in Scheme 1.
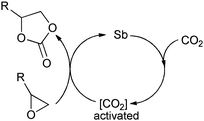 |
| Scheme 1 Possible mechanism of the reaction of CO2 with epoxides catalyzed by pentavalent organoantimony compounds.59 | |
It was also reported that Ph4SbBr is an effective catalyst for the synthesis of 3-phenyloxazolidin-2-one from CO2 and 1-phenylaziridines. At 80 °C in polar aprotic solvents such as hexamethylphosphoramide (HMPA) and dimethyl sulfoxide (DMSO), the yield is up to 93% and 85%, respectively (Scheme 2).61
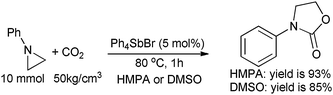 |
| Scheme 2 Reaction of carbon dioxide with 1-phenylaziridine catalyzed by organoantimony compound Ph4SbBr. | |
In 1992, Nomura et al. reported an efficient catalytic system that involves the combined use of tetraphosphorus decasulfide (P4S10) and triphenylstibine oxide (Ph3SbO, 1f) for the synthesis of urea through amine carbonylation with CO2.62 In the Ph3SbO/P4S10 system, 1,3-dialkylurea (RNHCONHR, where R = Bu, i-Bu, s-Bu, t-Bu, allyl, Ph) and tetramethylurea were successfully synthesized from the corresponding amines at 80 °C and 4.9 MPa initial CO2 pressure. Based on the results of 13C NMR monitoring, it was concluded that the course of reaction involves the successive thiolation of carbamic acid to intermediate antimony carbamate species, followed by the aminolysis of carbamothioic acid to the final product (Scheme 3). Similarly, cyclic ureas can be synthesized through the carbonylation of diamines (RNHCH2CH2NHR′, where R, R′= H, H; Me, H; Ph, H; HOCH2CH2, H; HOCHMeCH2, H; Me, Me) (Table 2). Using the Ph3SbO/P4S10 catalyst system, trisubstituted ureas such as l-butyl-3,3-diethylurea can be generated through the selective cocarbonylation of butylamine and diethylamine.
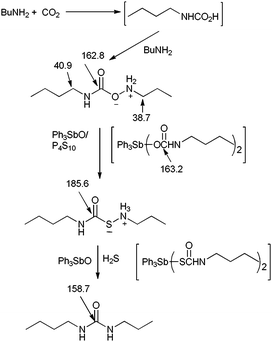 |
| Scheme 3 Synthesis of urea from amine and carbon dioxide (chemical shifts is δ(13C NMR)).62 | |
Table 2 Synthesis of cyclic urea from diamines and carbon dioxidea,62

|
R |
R′ |
Temp. (°C) |
Time (h) |
Yieldb (%) |
Reaction conditions: diamine/Ph3SbO/P4S10 = 20/1.0/2.0 mmol, benzene 20 mL, CO2 4.9 MPa. Isolated yields. Absence of the catalyst system. |
H |
H |
150 |
12 |
85 |
150 |
24 |
0c |
80 |
24 |
17 |
Me |
H |
120 |
12 |
60 |
Ph |
H |
100 |
24 |
40 |
HOCH2CH2– |
H |
60 |
24 |
95 |
HOCH(CH3)CH2– |
H |
120 |
24 |
54 |
Me |
Me |
120 |
24 |
75 |
Lermontov et al. reported that the Ph3SbF2 complex is a highly efficient catalyst for the synthesis of cyclic carbonates from epoxides and CO2 in HMPA at 180 °C, giving 100% yield in 5.5 h.63 They proposed that an efficient catalyst for this kind of reaction should be able to behave as a weak acid (for electrophilic activation of the oxirane ring) as well as a weak base (for nucleophilic activation of CO2). It is understandable because a strong acid means fast polymerization of oxirane and a strong base means irreversible binding of CO2. They proposed that β-fluoroalkoxide resulting from electrophilic opening of the oxirane ring (eqn (1)) serves as a base. To check this proposal, they carried out the reaction of propylene oxide with Ph3SbF2 in the absence of CO2, and detected fluoroalkoxide anions by 19F NMR spectroscopy (eqn (2)). In addition, the fluorocarbonate anion resulting from the reaction of fluoride with CO2 (eqn (3)) could also serve as a base. However, they did not observe any absorption band of FCOO– in the IR spectrum of a mixture of CsF, HMPA, and CO2; the result, nonetheless, does not exclude the possible formation of FCOO– under the adopted reaction conditions, viz. 140–180 °C and 70–100 atm CO2 pressure.
The results of Lermontov et al. showed that the catalytic mechanism of Ph3SbF2 is different from that of Ph4SbBr proposed by Nomura et al.62 The former involves CO2 activation by the organoantimony complex, followed by interaction with epoxide. As for the latter, it is epoxide that is first activated followed by interaction with CO2.
|
 | (1) |
|
 | (2) |
|
 | (3) |
2.2 Organoantimony(III) complexes
In 2009, Dostál et al. reported for the first time the chemical fixation of CO2 over a trivalent organoantimony(III) complex, showing clear evidence of CO2 insertion.64 The dimeric organoantimony(III) oxide (ArSbO)2 ((Ar) NCN chelating ligand C6H3-2,6-(CH2NMe2)2) was obtained by reacting ArSbCl2 with KOH (Scheme 4). The oxide bonds with CO2 to form air-stable monomeric carbonate ArSbCO3. In turn, CO2 can be released by heating the carbonate at 130 °C for (ArSbO)2 recovery (Scheme 4). The image reproduced from ref. 46, 47 and 91 with permission of Elsevier.
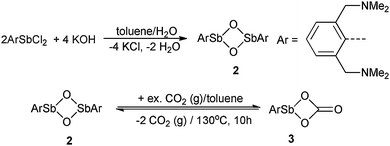 |
| Scheme 4 Preparation of (ArSbO)2 (2) and reversible fixation of carbon dioxide by 2 to form ArSbCO3 (3).64 | |
The molecular structure of (ArSbO)2 3 was unambiguously determined by X-ray analysis (Fig. 1). Single crystals of 3 were obtained through slow evaporation of CH2Cl2 from a (ArSbO)2/CH2Cl2 solution. The (ArSbO)2 compound was obtained as a solvate with two molecules of water. Despite the use of dried solvents and under an atmosphere of pure argon, it was not possible to obtain anhydrous (ArSbO)2 single crystals. The interesting feature of the molecular structure of 3 is the coordination of the carbonate moiety as a terminal ligand in a chelating fashion.
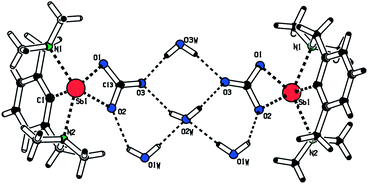 |
| Fig. 1 Pluton plot showing hydrogen bonding in 3. Selected O⋯H–O contacts (Å) and angles (deg.) characterizing respective hydrogen bonding: O(3)⋯H–O(3w) 2.829(5), 166.8; O(3)⋯H–O(2w) 2.773(5), 123.4; O(2)⋯H–O(1w) 2.969(5), 133.2; O(2w)–H–O(1w) 2.833(6), 175.9.64 (The image reproduced from ref. 64 with permission from the ACS.) | |
3. CO2 fixation using organobismuth complexes
3.1 Organobismuth complexes as CO2 absorbent
Compared to the use of organoantimony complexes, the use of organobismuth complexes for chemical fixation of CO2 is rare. For efficient interaction with CO2, the metal can either be a Lewis base, showing π-type interaction with one of the C
O bonds of CO2, or be a Lewis acid (or an oxophile) interacting with an oxygen atom of CO2. The use of organobismuth complex containing Bi–O bond for CO2 fixation was only reported recently.
In 2008, Breunig et al. firstly reported that exposure of a diethyl ether or a toluene solution of hypervalent diarylbismuth hydroxide [2-(Me2NCH2)C6H4]2BiOH (4) as well as that of the corresponding oxide [(2-(Me2NCH2)C6H4)2Bi]2O (5) to air led to CO2 adsorption and the formation of carbonate [(2-(Me2NCH2)C6H4)2Bi]2CO3(6) (Scheme 5).65 However, the authors did not mention whether carbonate 6 could be transferred back to hydroxide 4 or oxide 5 or not.
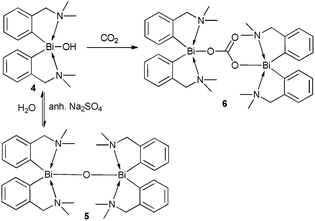 |
| Scheme 5 Chemical fixation of CO2 with hypervalent organobismuth(III) hydroxide (4) and oxide (5) to carbonate (6) with the pendant arm ligand of 2-(Me2NCH2)C6H4.65 | |
Later in the same year, Shimada and co-workers reported another kind of hypervalent organobismuth complexes with a 5,6,7,12-tetrahydrodibenz[c,f][1,5]azabismocine framework.66 The synthesis of organobismuth oxide (7), hydroxide (8) and alkoxide (9) are shown in Scheme 6.
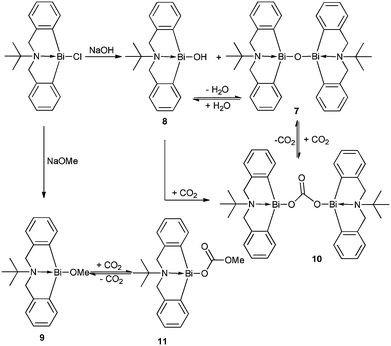 |
| Scheme 6 Chemical fixation of CO2 with hypervalent organobismuth(III) oxide (7), hydroxide (8), and alkoxide (9) to carbonate (10) and methyl carbonate (11) with a 5,6,7,12-tetrahydrodibenz[c,f][1,5]azabismocine framework.66 | |
The bismuth oxide 7 can be obtained by treating the bismuth chloride with aqueous sodium hydroxide. Analogous to the result of Breunig et al.,65 bismuth hydroxide 8 readily loses a water molecule to form oxide 7. In turn, oxide 7 can be completely converted back to hydroxide 8 by the inclusion of a water molecule. When a toluene solution of 8 is vigorously stirred in air at room temperature (RT) for 4.5 h, there is quantitative conversion of 7 to bismuth carbonate 9 (Scheme 6). Also, when an anhydrous CH2Cl2 solution of 7 or 8 is exposed to CO2 (1 atm), a quantitative amount of 10 is obtained readily. The thermal ellipsoid plot of 10 is shown in Fig. 2. It is noted that carbonate 10 is thermally rather stable, and in CH2Cl2 or under vacuum at RT, there is no decomposition of carbonate 10. Partial dissociation of carbonate 10 (ca. 30%) and quantitative regeneration of oxide 7 was observed when 10 was heated under vacuum at 100 °C for 10 h. For effective utilization of compounds 8 and 7, it is critical for them to show association as well as dissociation ability with CO2. Since it is possible to regenerate compounds 7 and 8 after CO2 absorption, the two are potential candidates for CO2 capture. It is observed that bismuth methoxide 9 in methanol, CH2Cl2, or toluene readily reacts with dry CO2 (1 atm) to form bismuth methyl carbonate 11, and the process is reversible (Fig. 3). Because methoxide 9 is sensitive to water, it is subject to hydrolysis and undergoes the subsequent reaction in the presence of water.
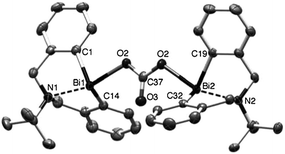 |
| Fig. 2 A thermal ellipsoid plot (50% probability level) of 10. Hydrogen atoms are omitted for clarity.66 (The image reproduced from ref. 66 with permission from the Wiley.) | |
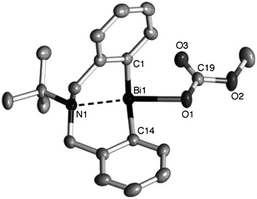 |
| Fig. 3 A thermal ellipsoid plot (50% probability level) of 11. Hydrogen atoms are omitted for clarity.66 (The image reproduced from ref. 66 with permission from the Wiley.) | |
3.2 Organobismuth complexes as CO2-fixation catalysts
Yin et al. investigated the possible application of 7 as a catalyst for 2-oxazolidinone synthesis through the fixing of CO2 into 2-aminoethanol.66 When an N-methylpyrrolidone (NMP) solution of 2-aminoethanol under CO2 (1 atm) was heated at 170 °C for 16 h in the presence of 5 mol% of 7, approximately 5% (determined by 1H NMR spectroscopy) of 2-oxazolidinone was formed, whereas there was only a trace (less than 0.5%) of 2-oxazolidinone detected in the absence of 7 under the same conditions. Despite the low activity of 7, it is considered that the presence of 7 has positive effect on the formation of 2-oxazolidinone. After the reaction, there is a small amount of black deposit in the reaction mixture. The 1H NMR spectrum of the reaction solution after the reaction shows only one kind of bismuth species. The results suggest partial decomposition (less than 14%) of the bismuth compound. On the other hand, there was complete decomposition of bismuth oxide [((2,4,6-Me3C6H2)2Bi)2O] under the same reaction conditions, and the bismuth oxide did not show any positive effect on 2-oxazolidinone formation.
Later, Yin and Shimada synthesized two new bismuth compounds (12 and 13) bearing a sulfur-bridged bis(phenolato) ligand. They found that these complexes could be used in the solvent-free synthesis of propylene carbonate from CO2 and propylene oxide in the presence of LiI.67
Fig. 4 and 5 show the molecular structures of 12 and 13, respectively. In the solid state, 12 and 13 form dimers through intermolecular Bi–O and Bi–I interactions. The Bi atom in 12 is five-coordinate 12–Bi–5, and its geometry can be described as highly distorted square pyramidal, while that in 13 is six-coordinate 14–Bi–6 having a tetrahydrofuran (THF) molecule as an additional ligand, and its geometry is distorted octahedral. These structural features clearly show the Lewis-acid nature of the bismuth centers of 12 and 13, and are in contrast to those of the trivalent phosphorus compounds bearing 2,20-thiobis-(phenolato) ligands.
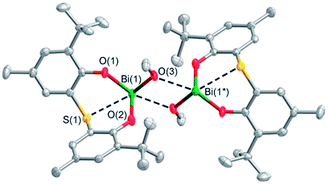 |
| Fig. 4 A thermal ellipsoid plot of compound 12 (50% probability level). Hydrogen atoms on the carbon atoms are omitted for clarity.67 (The image reproduced from ref. 67 with permission from the RSC.) | |
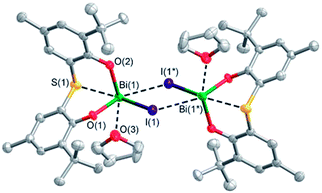 |
| Fig. 5 A thermal ellipsoid plot of compound 13 (50% probability level). Hydrogen atoms on the carbon atoms are omitted for clarity.67 (The image reproduced from ref. 67 with permission from the RSC.) | |
Results of initial attempts of using 7 or 10 as catalyst for the synthesis of propylene carbonate (PC) from propylene oxide (PO) and CO2 showed that these compounds are catalytically active. Nonetheless, bismuth methoxide 12 (catalyst loading = 0.24 mol%) shows low catalytic activity, giving a GC yield of only 29% in 10.5 h at 120 °C with initial CO2 pressure of 35 atm.
However, in the presence of an iodide salt as co-catalyst, compound 12 exhibits remarkable catalytic activity even under mild reaction conditions. As shown in Table 3, compound 13 is slightly superior to 12 in catalytic performance. The combined use of 13 and LiI results in nearly quantitative yield within 24 h (Table 3, entry 11, yield 98%). The catalytic activity of 13 is much higher than those (at RT and 1 atm CO2) reported by Ratzenhofer and Kisch.68 With 1H NMR monitoring, it was found that the reaction of 12 with CO2 does not proceed with the formation of a bismuth carbonate. In the solution, the monomers and dimers of 12 are at equilibrium, having composition depending on solvent polarity. In the case of 13, the compound exists mostly as monomers even in a C6D6 solution. It is deduced that both 12 and 13 act as monomeric Lewis acid catalysts, and their mechanistic action is similar to that of the commonly proposed acid–base or electrophile–nucleophile bifunctional mechanism.20 Hence the high efficiency of the catalyst systems can be attributed to the hypervalent structures of 12 and 13. The intramolecular coordination of the sulfur atom to the bismuth atom is extremely flexible, and the flexibility enables the adjustment of Lewis acidity of the bismuth atom. The outcome is facile exchange of product and reactant.
Table 3 PC synthesis from PO and CO2 catalyzed by 12 (or 13)/iodide catalyst systema,67
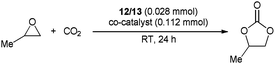
|
Entry |
Catalyst |
Conversionb (%) |
PC yieldb (%) |
The coupling reaction was conducted in a glass flask. The product was analyzed by NMR and GC-MS spectroscopies. The conversion and the PC yield were estimated by 1H NMR spectroscopy. |
1 |
12 |
0 |
0 |
2 |
13 |
0 |
0 |
3 |
Me3PhPI |
0 |
0 |
4 |
13 + Me3PhPI |
76 |
76 |
5 |
Bu4NI |
0 |
0 |
6 |
13 + Bu4NI |
77 |
77 |
7 |
NaI |
14 |
14 |
8 |
13 + NaI |
91 |
91 |
9 |
LiI |
26 |
26 |
10 |
12 + LiI |
92 |
92 |
11 |
13 + LiI |
98 |
98 |
To achieve high and efficient conversion of CO2 into valuable chemicals, and to exploit new applications of organobismuth compounds, Yin et al. developed another efficient catalytic system for the synthesis of cyclic carbonate from epoxide and CO2.69 They studied for the first time the cationic organobismuthcomplex (14) with the 5,6,7,12-tetrahydrodibenz[c,f][1,5]azabismocine framework for the transformation of CO2 into epoxide as cyclic carbonates, using terminal epoxides as substrates and tetrabutylammonium halide as co-catalyst under mild conditions (Table 4). It is a solvent-free system and the catalyst exhibits high activity and selectivity for the coupling reaction of CO2 with a wide range of terminal epoxides. The selectivity to propylene carbonates could reach 100%, and the maximum turnover frequency was up to 7800 h−1 at 120 °C and 3 MPa initial CO2 pressure when tetrabutylammonium iodide (1
:
1) was used as co-catalyst (entry 1). Moreover, the catalyst is environment-benign, resistant to air and water, and can be readily reused and recycled without any loss of activity (Fig. 6). In other words, the catalyst has great potential for industrial application.
Table 4 Coupling of CO2 with terminal epoxides catalyzed by cationic organobismuth complex (14) + nBu4NIa,69

|
Entry |
R |
R′ |
Conv.d (%) |
Sel.d (%) |
TOFd (h−1) |
Reaction conditions: epoxide, 120 mmol; Bi compound (14), 0.015 mmol; Bu4NI, 0.015 mmol; initial CO2 pressure, 3.0 MPa; 120 °C. Reaction time, 80 min. Reaction time, 3 h. GC analysis, conv.: epoxide conversion; sel.: cyclic carbonate selectivity; TOF: moles of epoxide converted per mole of Bi per hour. |
1 |
Me |
H |
97.5 |
>99.0 |
7800 |
2 |
Et |
H |
92.3 |
>99.0 |
7384 |
3 |
Bu |
H |
86.2 |
>99.0 |
6896 |
4 |
ClCH2 |
H |
90.7 |
>99.0 |
7256 |
5b |
Ph |
H |
87.9 |
>99.0 |
5274 |
6c |
–CH2(CH2)2CH2– |
|
90.3 |
>99.0 |
2408 |
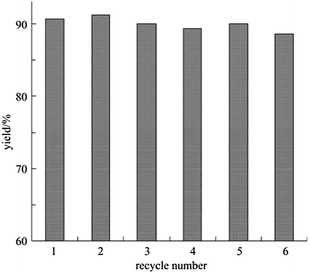 |
| Fig. 6 Catalyst recycling using epoxy chloropropane as substrate. For each cycle: epoxy chloropropane, 120 mmol; Bi compound (14), 0.015 mmol; Bu4NI, 0.015 mmol; CO2 pressure, 3 MPa; 120 °C; 1 h (ref. 69) (The image reproduced from ref. 69 with permission from the Springer). | |
Encouraged by the results, Yin et al. incorporated nBu4NI into binuclear organobismuth oxide complex 7 to generate a bifunctional Lewis acid–base system that shows cooperative catalytic effect for CO2 fixation (Scheme 6).70 Despite binuclear organobismuth oxide (7) having a framework similar to that of bimetallic Salen (Al) (complexes known to be catalytically highly efficient towards the reaction),71 complex 7 shows no catalytic activity when 2-methyloxirane is adopted as substrate (Scheme 7, Route A). Nonetheless, when 2-(chloromethyl)oxirane is adopted as substrate, cyclic carbonates are obtained almost quantitatively (Scheme 7, Route B). Furthermore, when Bu4NI is used as co-catalyst (0.1 mol% based on epoxide), CO2 insertion into 2-methyloxirane occurs in a quantitative manner (Scheme 7, Route C). In contrast to the results of monomeric organobismuth chlorides, methoxide and methanethiolate, the findings of binuclear organobismuth oxide shows cooperative action that enables high efficiency in the synthesis of cyclic carbonates from epoxides and CO2.
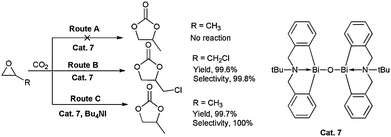 |
| Scheme 7 Selective insertion of CO2 into epoxides catalyzed by cat. 7 (0.5 mol%) with or without co-catalyst Bu4NI (0.1 mol%).70 | |
However, the binuclear organobismuth oxides 7, 18, 19 are sensitive to moisture and CO2, and the rigorous requirements for manipulation limit their utilization. For practical application in organic synthesis, a catalyst is better to be air-stable.49 Interestingly when the bridging atom is changed from oxygen to sulfur (Scheme 8), the binuclear organobismuth sulfides LBi–S–BiL (20–22) are air-stable. The compounds could remain as dry crystals or powder (slightly yellow in color) for more than one year in ambient environment. These sulfur-bridged binuclear organobismuth complexes represent a rare class of complexes that are air-stable.
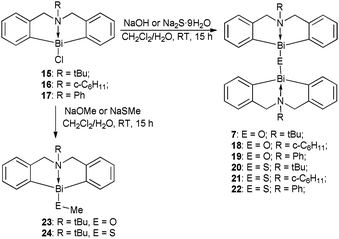 |
| Scheme 8 Synthetic routes of organobismuth complexes 7, 18–24 (ref. 70). | |
The crystal structures of 20–22 freshly obtained after recrystallization in toluene/hexane (CH2Cl2/hexane for 20) were determined by X-ray analysis. As shown in Fig. 7, the 5,6,7,12-tetrahydrodibenz[c,f][1,5]azabismocine framework of complexes 20–22 is bridged by a sulfur atom that occupies a vacant site of the bismuth centre. In the equatorially distorted trigonal bipyramidal geometry, the nitrogen and sulfur atoms occupy the apical positions whereas the carbon atoms occupy the equatorial positions. With sulfur bridging, the bismuth centre is exposed more efficiently than that of their precursors, making the two bismuth atoms of 20–22 closer to each other in comparison to the case of 7. Nonetheless, the distance between the two bismuth atoms of 20–22 is larger than that of a covalent Bi–Bi single bond (3.092(6)–3.2092(8))72 and Bi
Bi double bond (3.0648(2)).73 This can be considered as a favorable parameter for maintaining suitable stability of the bimetallic complexes. In addition, the five linked atoms N(1)–Bi(1)–S(1)–Bi(1A)–N(1A) are almost in the same plane (angle distortion <0.5°) with the plane bisecting the butterfly-shaped tetrahydrodibenz[c,f][1,5]azabismocine framework. One can see that the butterfly-shaped phenyl planes of 20–22 are cis-geometrically located to make the two bismuth atoms available for cooperative action. The N-substituents of 20–22 are positioned close to the bismuth centers, protecting the Bi–C bond and Bi–S–Bi bridge from infringers such as water and CO2. Hence, by using sulfur atom for bridging and varying the N-substituent, one can regulate the state suitability as well as the ligands of the bismuth centers to mimic those of natural metalloenzymes.
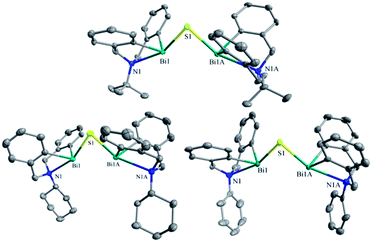 |
| Fig. 7 Thermal ellipsoid plots (50% probability level) of 20 (top), 21 (bottom left), 22 (bottom right). Hydrogen atoms on the carbon atoms are omitted for clarity.70 (The images reproduced from ref. 70 with permission from the RSC.) | |
To examine the cooperative action of the binuclear bismuth centers, complexes 7, 18–22 were examined for the synthesis of cyclic carbonates from epoxides and CO2 (Table 5), and their performance are compared with those of mononuclear organobismuth complexes 15–17 and 23, 24. One can see that most of them show good catalytic efficiency (entries 1–6, GC yield up to 100%). In terms of catalytic efficiency and air-stability, the N-substituent with flexible and electron-donating group (tBu, 20; Cy, 21) is superior to organobismuth oxides (7, 18–19) (entries 1–3) as well as to those with rigid and electron-withdrawing group (Ph, 22) (entries 4–6). In contrast to the mononuclear organobismuth complexes (entries 7–11), the binuclear organobismuth complexes show a significant cooperative effect on CO2 transformation. Without a catalyst, the product yield is only 3.7% (entry 12). The results indicate that by simple modification of the N-substituent and the bridging atom in the main framework of bimetallic organobismuth complexes, one can tune catalytic activity as well as achieving air-stability.
Table 5 Optimization of reaction condition in cyclic carbonate synthesis from 2-(chloromethyl)oxirane and CO2a,70
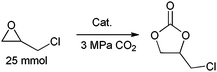
|
Entry |
Cat. (mol%) |
Time (h) |
Temp. (°C) |
Yield (%) |
Sel. (%) |
Reaction conditions: 2-(chloromethyl)oxirane, 2 mL (25 mmol); initial CO2 pressure, 3.0 MPa, GC yield. Blank experiment. |
1 |
7 (0.5) |
8 |
140 |
99.6 |
99.8 |
2 |
18 (0.5) |
8 |
140 |
100 |
100.0 |
3 |
19 (0.5) |
8 |
140 |
97.3 |
98.1 |
4 |
20 (0.5) |
8 |
140 |
99.4 |
99.7 |
5 |
21 (0.5) |
8 |
140 |
94.3 |
98.3 |
6 |
22 (0.5) |
8 |
140 |
65.8 |
98.0 |
7 |
15 (1.0) |
8 |
140 |
25.9 |
97.7 |
8 |
16 (1.0) |
8 |
140 |
41.3 |
98.3 |
9 |
17 (1.0) |
8 |
140 |
5.9 |
96.9 |
10 |
23 (1.0) |
8 |
140 |
32.5 |
98.3 |
11 |
24 (1.0) |
8 |
140 |
14.7 |
92.6 |
12b |
— |
8 |
140 |
3.7 |
98.2 |
The catalytic performance of 20 was also investigated under optimized conditions across a diversity of epoxides (Table 6). In most of the cases, including 2-phenyloxirane and 7-oxabicyclo[4.1.0]heptane of large steric hindrance, the catalyst is highly effective (entries 1–6). The co-presence of Bu4NI is favorable for CO2 insertion into the non-halide epoxides (entries 2 and 7).
Table 6 Synthesis of cyclic carbonates from CO2 and epoxidesa,70
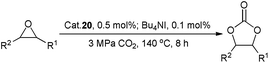
|
Entry |
R1 |
R2 |
Conv. (%) |
Yield (%) |
Reaction conditions: 2-(chloromethyl)oxirane, 2 mL; initial CO2 pressure 3.0 MPa, cat. 20, 0.5 mol%, 8 h, 140 °C. Without co-catalyst Bu4NI. Catalyst loading of 20 was 1.0 mol%. 12 h. |
1 |
H |
H |
100.0 |
98.2 |
2 |
CH3 |
H |
99.7 |
99.7 |
3b |
CH2Cl |
H |
100.0 |
99.8 |
4c |
CH3 |
CH3 |
76.2 |
76.0 |
5d |
Ph |
H |
99.5 |
99.5 |
6c |
–CH2(CH2)2CH2– |
62.3 |
62.1 |
99.3 |
7b |
CH3 |
H |
2.3 |
2.3 |
3.3 Bi–Fe bimetallic complexes for CO2 fixation
In addition to the organobismuthcomplexes with 5,6,7,12-tetrahydrodibenz[c,f][1,5]azabismocine framework, there are other organobismuth complexes that are catalytically active for CO2 fixation. It was reported by Wójcik et al. that the reaction of Fe2(CO)9 with Bi(OSiMe2tBu)3 would result in the formation of soluble [(CO)4FeBi(OSiMe2tBu)]2 (25) in moderate yield (Fig. 8). Complexes [(CO)4FeBi(OtBu)]n (26) and [(CO)3FeBi3(OtBu)4(OCO(OtBu))]2 (27) were obtained when Bi(OtBu)3 was used as starting material (Fig. 9).74 During the reaction of diiron nonacarbonyl with bismuth tert-butoxide, complex 27 was released upon the insertion of CO2 into a Bi-OtBu bond. The compounds were characterized by IR and 1H NMR spectroscopy as well as thermo-gravimetric analysis. Additionally, the molecular structures of compounds 25 and 27 were elucidated by single crystal X-ray diffraction. The core structure of [(CO)4FeBi(OSiMe2tBu)]2 (25) is a four-membered Bi2Fe2 ring. As for 27, the compound is composed of two cores of tetrahedral FeBi3 clusters that dimerise via the bridging of –OCO(OtBu) ligands. Analysis of the TGA residues by powder X-ray diffraction revealed that compound 26 is the best precursor for the generation of multi-ferroic BiFeO3 among the compounds studied, despite the detection of Bi25FeO39 as minor impurity.
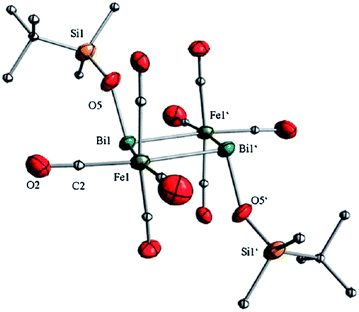 |
| Fig. 8 ORTEP diagram (50% probability level) of the molecular structure of [(CO)4FeBi(OSiMe2tBu)]2 (25), H atoms are omitted for clarity.74 (The image reproduced from ref. 74 with permission from the Elsevier.) | |
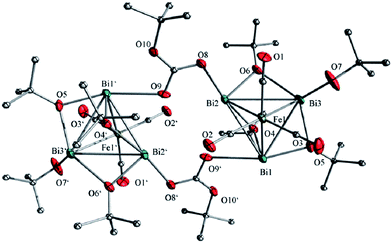 |
| Fig. 9 ORTEP diagram (50% probability level) of the molecular structure of [(CO)3FeBi3(OtBu)4(OCO(OtBu))]2 (27), H atoms are omitted for clarity.74 (The image reproduced from ref. 74 with permission from the Elsevier.) | |
3.4 CO2 insertion into Bi–C bond of an organobismuth complex
Just recently, Kindra et al. demonstrated yet another example of CO2 insertion into Bi–C bonds of bismuth NCN pincer complex bearing an oxyaryl dianionic ligand [2,6-(Me2NCH2)2C6H3]Bi(C6H2-tBu2O).75 The reactivity of the unusual oxyaryl dianionic ligand, (C6H2tBu2-3,5-O-4)2−, in the Bi3+ NCN pincer complex Ar′Bi(C6H2tBu2-3,5-O-4) (28) [Ar′ = 2,6-(Me2NCH2)2C6H3], was explored with substrates and electrophiles of small molecules. The first insertion reaction of CO2 into Bi–C bond was observed over this oxyaryl dianionic ligand complex (Scheme 9). The reactions generate new dianions that have quinoidal character similar to that of the oxyaryl dianionic ligand in 28. The oxyarylcarboxy dianionic ligand in Ar′Bi[O2C(C6H2tBu2-3-5-O-4)-κ2O,O′] (29) was identified by X-ray crystallography (Fig. 10).
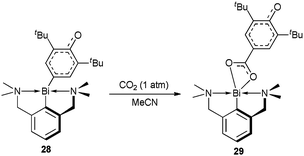 |
| Scheme 9 Insertion of CO2 into the Bi–C bond of bismuth NCN pincer complex of oxyaryl dianionic ligand [2,6-(Me2NCH2)2C6H3]Bi(C6H2-tBu2O).75 | |
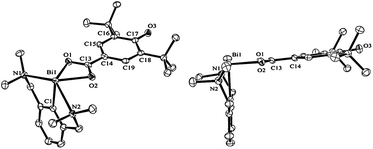 |
| Fig. 10 ORTEP representation of Ar′Bi[O2C(C6H2tBu2-3-5-O-4)-κ2O,O′] (29), from two different perspectives, with thermal ellipsoids drawn at t probability. Hydrogen atoms are omitted for clarity.75 (The image reproduced from ref. 75 with permission from the ACS.) | |
3.5 Physical fixation of CO2 with bismuth–organic frameworks
Cheetham's group76 reported two other bismuth complexes with metal–organic frameworks. They are inorganic–organic bismuth [LiBi(2,6-pdc)3(H2O)]·2(dma) (30) and Li5Bi(2,6-pdc)4(H2O)2 (31) with four Li+ cations per formula (where dma = dimethyl ammonium cation, dmf = dimethylformamide, and 2,6-pdc = pyridine 2,6-carboxylate). They were synthesized as efficient absorbents for CO2 absorption. The amount of CO2 that can be taken up by compound 31 is up to 140 cm3 g−1 (∼6 mmol g−1).
4. Summary
Organoantimony(bismuth) complexes have been used as absorbents and catalysts for CO2 fixation, giving organometallic carbonates and cyclic carbonates as products, respectively. The physical fixation of CO2 by inorganic–organic bismuth complexes and CO2 insertion into a Bi–C bond are new. Since 1979, efficient catalysts based on organoantimony (bismuth) have been developed, and most of them are electrophile–nucleophile or Lewis acid–Lewis base systems. The plausible catalytic mechanism involves the reaction of CO2 with a organoantimony(bismuth) catalyst to form an organometallic carbonate. With the opening of epoxide by a base such as nBu4NI, the organometallic carbonate reacts with the epoxide to give the cyclic carbonate. The organoantimony and organobismuth complexes have bright prospects in the field of CO2 fixation. Further work with organoantimony(bismuth) complexes as CO2 absorbent or catalyst should be focused on the following aspects: (i) simple and cheap CO2 absorbents whose CO2 association or dissociation is fast and efficient under mild condition, should be developed; (ii) air-stable, thermo-stable, recyclable, high Lewis acidic organoantimony(bismuth) complexes catalyst is still highly desirable; (iii) highly efficient synthetic method for the useful chemicals from CO2, such as polycyclic carbonate, dimethyl carbonate, and dimethyl ether is still not well established, especially in the industrial scale; (iv) the related catalytic mechanism for the generation of cyclic carbonates is unclear, lacking of direct evidence, such as NMR and X-ray evidence; and (v) the reactivity of the C–M bond with CO2 or other small molecules will be another challenge.
Acknowledgements
This work was supported by the NSFC (Grant nos 21373003, 21003040), the NSF of Hunan Province (14JJ7027), the program for New Century Excellent Talents in Universities (NCET-10-0371), Science Research Foundation for Higher Universities of Hunan Province (10A092), Research-Based Learning and Innovation Experiment Project for Undergraduates of Hunan Province (2011-483), the Fundamental Research Funds for the Central Universities, and the PCSIRT (IRT1238). The authors thank Dr S. Shimada and Dr L.-B. Han of AIST (Japan), Prof. W.-Y. Wong of Hong Kong Baptist University, Prof. N. Kambe and Dr T. Iwasaki of Osaka University, Profs J. Otera and A. Orita of Okayama University of Science for helpful discussion. C.T. Au thanks the Hunan University for an adjunct professorship.
Notes and references
- J. M. Lee, A. J. Clark and J. R. Roche, Grass Forage Sci., 2013, 68, 485–503 CrossRef.
- D. A. Castelo Branco, M. C. P. Moura, A. Szklo and R. Schaeffer, Energy Policy, 2013, 61, 1221–1235 CrossRef CAS PubMed.
- A. Gajewski, J. Siergiejuk and K. Szulborski, Energy and Buildings, 2013, 65, 197–204 CrossRef PubMed.
- T. Meriste, C. R. Yoeruek, A. Trikkel, T. Kaljuvee and R. Kuusik, J. Therm. Anal. Calorim., 2013, 114, 483–489 CrossRef CAS.
- I. Vorrias, K. Atsonios, A. Nikolopoulos, N. Nikolopoulos, P. Grammelis and E. Kakaras, Fuel, 2013, 113, 826–836 CrossRef CAS PubMed.
- L. Li, N. Zhao, W. Wei and Y. Sun, Fuel, 2013, 108, 112–130 CrossRef CAS PubMed.
- Y. Zhang, S. Luo and S. Yin, Prog. Chem., 2012, 24, 674–685 CAS.
- Y. Zhang and J. Y. G. Chan, Energy Environ. Sci., 2010, 3, 408–417 CAS.
- M. Mikkelsen, M. Jorgensen and F. C. Krebs, Energy Environ. Sci., 2010, 3, 43–81 CAS.
- P. Li and L. Liu, Prog. Chem., 2010, 22, 1940–1951 CAS.
- J. Ma, N. Sun, X. Zhang, N. Zhao, F. Mao, W. Wei and Y. Sun, Catal. Today, 2009, 148, 221–231 CrossRef CAS PubMed.
- D. Dong, L. Yang and W. Hu, Prog. Chem., 2009, 21, 1217–1228 CAS.
- J. M. Sun, S. Fujita and M. Arai, J. Organomet. Chem., 2005, 690, 3490–3497 CrossRef CAS PubMed.
- Y. M. Shen and M. Shi, Chin. J. Org. Chem., 2003, 23, 22–29 CAS.
- D. Chen and L. He, ChemCatChem, 2011, 3, 490–511 CrossRef CAS.
- B. Hu, K. Wang, L. Wu, S.-H. Yu, M. Antonietti and M.-M. Titirici, Adv. Mater., 2010, 22, 813–828 CrossRef CAS PubMed.
- G. Golemme and E. Drioli, J. Inorg. Organomet. Polym., 1996, 6, 341–365 CrossRef CAS.
- F. Jutz, J.-M. Andanson and A. Baiker, Chem. Rev., 2011, 111, 322–353 CrossRef CAS PubMed.
- J.-M. Saveant, Chem. Rev., 2008, 108, 2348–2378 CrossRef CAS PubMed.
- T. Sakakura, J.-C. Choi and H. Yasuda, Chem. Rev., 2007, 107, 2365–2387 CrossRef CAS PubMed.
- F. J. Millero, Chem. Rev., 2007, 107, 308–341 CrossRef CAS PubMed.
- P. G. Jessop and B. Subramaniam, Chem. Rev., 2007, 107, 2666–2694 CrossRef CAS PubMed.
- D. J. Darensbourg, Chem. Rev., 2007, 107, 2388–2410 CrossRef CAS PubMed.
- A. Stolow, A. E. Bragg and D. M. Neumark, Chem. Rev., 2004, 104, 1719–1757 CrossRef CAS PubMed.
- H. Arakawa, M. Aresta, J. N. Armor, M. A. Barteau, E. J. Beckman, A. T. Bell, J. E. Bercaw, C. Creutz, E. Dinjus, D. A. Dixon, K. Domen, D. L. DuBois, J. Eckert, E. Fujita, D. H. Gibson, W. A. Goddard, D. W. Goodman, J. Keller, G. J. Kubas, H. H. Kung, J. E. Lyons, L. E. Manzer, T. J. Marks, K. Morokuma, K. M. Nicholas, R. Periana, L. Que, J. Rostrup-Nielson, W. M. H. Sachtler, L. D. Schmidt, A. Sen, G. A. Somorjai, P. C. Stair, B. R. Stults and W. Tumas, Chem. Rev., 2001, 101, 953–996 CrossRef CAS PubMed.
- T. Y. Luh, M. K. Leung and K. T. Wong, Chem. Rev., 2000, 100, 3187–3204 CrossRef CAS PubMed.
- R. E. Weston, Chem. Rev., 1999, 99, 2115–2136 CrossRef CAS PubMed.
- D. Huang, O. V. Makhlynets, L. L. Tan, S. C. Lee, E. V. Rybak-Akimova and R. H. Holm, Inorg. Chem., 2011, 50, 10070–10081 CrossRef CAS PubMed.
- S. Kumar, S. L. Jain and B. Sain, Catal. Lett., 2012, 142, 615–618 CrossRef CAS.
- S. Sakamoto, T. Fujinami, K. Nishi, N. Matsurnoto, N. Mochida, T. Ishida, Y. Sunatsuki and N. Re, Inorg. Chem., 2013, 52, 7218–7229 CrossRef CAS PubMed.
- J. P. Wikstrom, A. S. Filatov, E. A. Mikhalyova, M. Shatruk, B. Foxman and E. V. Rybak-Akimova, Dalton Trans., 2010, 39, 2504–2514 RSC.
- H. Mizuno, J. Takaya and N. Iwasawa, J. Am. Chem. Soc., 2011, 133, 1251–1253 CrossRef CAS PubMed.
- H. V. Babu and K. Muralidharan, Dalton Trans., 2013, 42, 1238–1248 RSC.
- C. Chatterjee and M. H. Chisholm, Inorg. Chem., 2012, 51, 12041–12052 CrossRef CAS PubMed.
- S. Dalapati, S. Jana, R. Saha, M. A. Alam and N. Guchhait, Org. Lett., 2012, 14, 3244–3247 CrossRef CAS PubMed.
- L. Du, S. Zhang and Y. Ding, Z. Anorg. Allg. Chem., 2012, 638, 1039–1041 CrossRef CAS.
- X. Liu, S. Zhang and Y. Ding, Inorg. Chem. Commun., 2012, 18, 83–86 CrossRef CAS PubMed.
- S. Schulz, S. Schmidt, D. Blaeser and C. Woelper, Z. Anorg. Allg. Chem., 2012, 638, 1705–1710 CrossRef CAS.
- Z. Tasci and M. Ulusoy, J. Organomet. Chem., 2012, 713, 104–111 CrossRef CAS PubMed.
- D. Tian, B. Liu, L. Zhang, X. Wang, W. Zhang, L. Han and D.-W. Park, J. Ind. Eng. Chem., 2012, 18, 1332–1338 CrossRef CAS PubMed.
- Y.-W. Zhong, C.-J. Yao and H.-J. Nie, Coord. Chem. Rev., 2013, 257, 1357–1372 CrossRef CAS PubMed.
- W. Zhang and X. Lu, Chin. J. Catal., 2012, 33, 745–756 CrossRef CAS.
- Y. Tsuji and T. Fujihara, Chem. Commun., 2012, 48, 9956–9964 RSC.
- X. Sun, S. Chen, X. Zhang and G. Qi, Prog. Chem., 2012, 24, 1776–1784 CAS.
- M. Hoelscher, C. Guertler, W. Keim, T. E. Mueller, M. Peters and W. Leitner, Z. Naturforsch., B: J. Chem. Sci., 2012, 67, 961–975 CrossRef CAS.
- C. I. Rat, C. Silvestru and H. J. Breunig, Coord. Chem. Rev., 2013, 257, 818–879 CrossRef CAS PubMed.
- I. Caracelli, I. Haiduc, J. Zukerman-Schpector and E. R. T. Tiekink, Coord. Chem. Rev., 2013, 257, 2863–2879 CrossRef CAS PubMed.
- N. Tan, Y. Chen, S. Yin, R. Qiu, Y. Zhou and C. T. Au, Curr. Org. Chem., 2012, 16, 2462–2481 CrossRef CAS.
- R. Qiu, Y. Chen, S.-F. Yin, X. Xu and C.-T. Au, RSC Adv., 2012, 2, 10774–10793 RSC.
- P. Sharma, D. Perez, A. Cabrera, N. Rosas and J. L. Arias, Acta Pharmacol. Sin., 2008, 29, 881–890 CrossRef CAS PubMed.
- H. J. Breunig and L. Balazs, Organometallics, 2004, 23, 304–310 CrossRef CAS.
- L. Balazs and H. J. Breunig, Coord. Chem. Rev., 2004, 248, 603–621 CrossRef CAS PubMed.
- H. J. Breunig and R. Rosler, Chem. Soc. Rev., 2000, 29, 403–410 RSC.
- P. Sharma, A. Cabrera, S. Singh and N. K. Jha, Main Group Met. Chem., 1997, 20, 551–565 CAS.
- P. Sharma, A. Cabrera, N. K. Jha, N. Rosas, R. LeLagadec, M. Sharma and J. L. Arias, Main Group Met. Chem., 1997, 20, 697–710 CAS.
- C. Silvestru and I. Haiduc, Coord. Chem. Rev., 1996, 147, 117–146 CrossRef CAS.
- L. D. Freedman and G. O. Doak, J. Organomet. Chem., 1995, 486, 1–20 CrossRef CAS.
- H. Matsuda, A. Ninagawa and R. Nomura, Chem. Lett., 1979, 1261–1262 CrossRef CAS.
- R. Nomura, A. Ninagawa and H. Matsuda, J. Org. Chem., 1980, 45, 3735–3738 CrossRef CAS.
- R. Nomura, Y. Wada and H. Matsuda, J. Polym. Sci., Part A: Polym. Chem., 1988, 26, 627–636 CrossRef CAS.
- H. Matsuda, A. Ninagawa and H. Hasegawa, Bull. Chem. Soc. Jpn., 1985, 58, 2717–2718 CrossRef CAS.
- R. Nomura, Y. Hasegawa, M. Ishimoto, T. Toyosaki and H. Matsuda, J. Org. Chem., 1992, 57, 7339–7342 CrossRef CAS.
- S. A. Lermontov, S. V. Shkavror, A. S. Lermontov and S. L. Z. avorin, Russ. Chem. Bull., 1998, 47, 1607–1609 CrossRef CAS.
- L. Dostál, R. Jambor, A. Růžička, M. Erben, R. Jirásko, E. Cernošková and J. Holeček, Organometallics, 2009, 28, 2633–2636 CrossRef.
- H. J. Breunig, L. Konigsmann, E. Lork, M. Nema, N. Philipp, C. Silvestru, A. Soran, R. A. Varg and R. Wagner, Dalton Trans., 2008, 11831–11842 Search PubMed.
- S.-F. Yin, J. Maruyama, T. Yamashita and S. Shimada, Angew. Chem., Int. Ed., 2008, 47, 6590–6593 CrossRef CAS PubMed.
- S.-F. Yin and S. Shimada, Chem. Commun., 2009, 1136–1138 RSC.
- M. Ratzenhofer and H. Kisch, Angew. Chem., Int. Ed. Engl., 1980, 19, 317–318 CrossRef.
- X. Zhang, W. Dai, S. Yin, S. Luo and C.-T. Au, Front. Environ. Sci. Eng. China, 2009, 3, 32–37 CrossRef CAS.
- R. Qiu, Z. Meng, S. Yin, X. Song, N. Tan, Y. Zhou, K. Yu, X. Xu, S. Luo, C.-T. Au and W.-Y. Wong, ChemPlusChem, 2012, 77, 404–410 CrossRef CAS.
- M. North and R. Pasquale, Angew. Chem., Int. Ed., 2009, 48, 2946–2948 CrossRef CAS PubMed.
- L. Balazs, H. J. Breunig, E. Lork, A. Soran and C. Silvestru, Inorg. Chem., 2006, 45, 2341–2346 CrossRef CAS PubMed.
- S. Shimada, J. Maruyama, Y.-K. Choe and T. Yamashita, Chem. Commun., 2009, 6168–6170 RSC.
- K. Wójcik, T. Rüffer, H. Lang, A. A. Auer and M. Mehring, J. Organomet. Chem., 2011, 696, 1647–1651 CrossRef PubMed.
- D. R. Kindra, I. J. Casely, M. E. Fieser, J. W. Ziller, F. Furche and W. J. Evans, J. Am. Chem. Soc., 2013, 135, 7777–7787 CrossRef CAS PubMed.
- A. Thirumurugan, W. Li and A. K. Cheetham, Dalton Trans., 2012, 41, 4126–4134 RSC.
|
This journal is © The Royal Society of Chemistry 2014 |