DOI:
10.1039/C3RA46896H
(Paper)
RSC Adv., 2014,
4, 10295-10302
Novel oxovanadium(IV) complexes with 4-acyl pyrazolone ligands: synthesis, crystal structure and catalytic activity towards the oxidation of benzylic alcohols†
Received
22nd November 2013
, Accepted 20th December 2013
First published on 20th December 2013
Abstract
A series of oxovanadium(IV) complexes of 4-acylpyrazolone ligands were synthesized and characterized by elemental analyses, FT-IR, UV-Vis, EPR spectroscopy and single crystal XRD. The single-crystal X-ray analysis of the complex VO(L1)2 shows that the ligands were coordinated with the vanadium atom in a twisted form to create a distorted octahedral geometry and two O,O-chelating acylpyrazolonate ligands constitute two six-membered rings with the vanadium atom. The catalytic activity of all the complexes was evaluated for the oxidation of benzylic alcohols with H2O2 as an oxidant. The conditions for maximum conversion as well as selectivity for the desired product were optimized by varying different parameters such as the molar ratio of substrate to H2O2, the amount of the catalyst, reaction time and solvent. A possible pathway for the oxidation of benzylic alcohols was also proposed on the basis of the spectral evidence.
Introduction
Vanadium compounds have attracted much attention because of their involvement in various biological processes1 and for their use as homogeneous and heterogeneous catalysts in various reactions and in industrial processes.2 Vanadium based oxidants are effectively used for various oxidation reactions such as the epoxidation of alkenes,3 the oxidation of sulfides,2b,4 hydro and oxidative amination5 and the oxidation of alcohols6 to aldehydes and ketones, thus showing their influence on the yield and selectivity in chemical transformations. Oxo and peroxo derivatives of vanadium complexes play an important role in such catalytic oxidations, acting as oxo-transfer agents.7 Aqueous hydrogen peroxide is a highly attractive oxidant because it is a cheap, mild and environmentally benign reagent with a high content of ‘active’ oxygen, and water is the only by-product. Thus, many catalytic systems were studied for selective oxidation of alcohols with H2O2.8 The catalytic species resulting from the interaction of hydrogen peroxide with suitable transition metal ions, are considered among the most active oxidants9 toward a number of organic and inorganic substrates.
The selective oxidation of alcohols to carbonyl compounds is one of the most important organic transformations, with recognized importance to fundamental organic synthesis, but also to the fine chemical industry.6,10 Selective oxidation of benzyl alcohol to benzaldehyde is a practically important reaction for the production of chlorine-free benzaldehyde, required in the perfumery and pharmaceutical industries.11 Benzaldehyde is an attractive target because it is an important intermediate in the production of derivatives for perfumery, pharmaceutical, dyestuff and agrochemical industries.
Recently, we have reported the synthesis and crystal structure of a novel oxovanadium(IV) complex with 1-phenyl-3-methyl-4-toluoyl-5-pyrazolone ligand and immobilized it over hydrous zirconia to use as a heterogeneous catalyst for the oxidation of styrene with H2O2.12 To the best of our knowledge no report is available in the literature for the selective oxidation of alcohols with the oxovanadium(IV) complexes of acylpyrazolone ligands. The objective of the present study is to emphasis the geometry of oxovanadium(IV) complexes of acylpyrazolone ligands and to establish the viability of the newly synthesized oxovanadium(IV) complexes (Scheme 1) in oxidation reactions. The catalytic properties of the new mononuclear complexes have been thoroughly studied, in the hydrogen peroxide promoted oxidation of benzylic alcohols, under homogeneous conditions. To investigate the effectiveness of the synthesized complexes in oxidation reactions, benzyl alcohol was chosen as a model substrate. The oxidation of benzyl alcohol was studied in more detail to optimize the reaction variables such as the amount of catalyst, alcohol/oxidant molar ratio, length of reaction time and solvent. To evaluate the scope and limitations of the current procedure, oxidation reactions with a wide array of electronically diverse benzylic alcohols were examined using the oxovanadium(IV) complex of 1-toluoyl-3-methyl-4-phenyl-5-pyrazolone ligand.
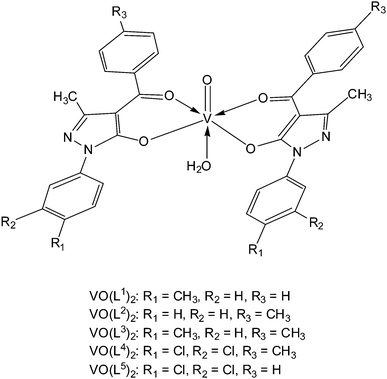 |
| Scheme 1 General structure for the synthesized complexes (1–5). | |
Experimental section
Materials and methods
All reagents and solvents were purchased from commercial sources and were further purified by standard methods, if necessary. Pyrazolone derivatives were obtained from Nutan Dye Chem. Sachin, Surat. Vanadyl sulfate, acetonitrile, benzyl alcohol, hexane and 30% aqueous H2O2 were purchased from Merck and used as received. 2-Methoxy benzyl alcohol, 3-methoxy benzyl alcohol, 4-methoxy benzyl alcohol, 2-chloro benzyl alcohol, 3-chloro benzyl alcohol, 4-methyl benzyl alcohol, and 4-nitro benzyl alcohol were purchased from Sigma-Aldrich.
The synthesized ligands and their oxovanadium(IV) complexes were characterized by 1H and 13C NMR, EI-MS, single crystal XRD, FT-IR, EPR and ESI-MS. 1H and 13C NMR spectra of ligands were recorded with an Avance-III 400 MHz Bruker FT-NMR instrument. Elemental analyses of C, H, and N were determined using a Perkin Elmer series-II 2400 elemental analyzer. GC-MS analysis was carried out using a gas chromatograph mass spectrometer (Agilent 5975 GC/MSD with 7890A GC system) having a HP-5 capillary column of 60 m length and 250 μm diameter with a programmed oven temperature from 50 to 280 °C, at 1 mL min−1 flow rate of He as a carrier gas and ion source at 230 °C. X-ray intensity data of 14753 reflections (of which 6979 unique) were collected on a Bruker CCD area-detector diffractometer equipped with graphite monochromated MoKα radiation (λ = 0.71073 Å). FT-IR spectra of ligands and complexes were recorded using KBr pellets on a Perkin Elmer Fourier transform (FT-IR) spectrum RX 1 spectrometer. Electronic spectra were recorded on a Perkin Elmer Lambda 35 UV-Vis spectrometer. EPR spectrum was recorded on the X-band instrument in the EPR laboratory, SAIF, IIT, Bombay, at liquid nitrogen temperature. Electrospray ionization mass spectrometry (ESI-MS) spectra were recorded on a Waters Q-ToF micromass at SAIF, Panjab University, Chandigarh.
Synthesis of oxovanadium(IV) complex VO(L1)2. To a well-stirred hot ethanolic solution of ligand L1 (0.292 g, 1 mmol), an aqueous solution of VOSO4·5H2O (0.127 g, 0.5 mmol) was added dropwise. The reaction mixture was refluxed with stirring for 6 hours. A green precipitate was formed which was filtered, washed with hot distilled water and then washed with ethanol and dried under vacuum yielding 0.207 g (71%) green solid. ESI-MS calcd for C36H30N4O5V [M]+ 649.17; found 650.14 [M + H]+. C36H32N4O6V [VO(L1)2·H2O] (667.6): calcd C 64.77, H 4.83, N 8.39; found C 64.82, H 4.89, N 8.39.
Synthesis of complex VO(L2)2. The complex was synthesized by the previously reported procedure.12 Yield 0.210 g (72%). ESI-MS calcd for C36H30N4O5V [M]+ 649.17; found 650.03 [M + H]+. C36H32N4O6V [VO(L2)2·H2O] (667.6): calcd C 64.77, H 4.83, N 8.39; found C 64.71, H 4.87, N 8.40.
Synthesis of complex VO(L3)2. The complex was synthesized by a similar procedure to VO(L1)2. Yield 0.205 g (67%). ESI-MS calcd for C38H34N4O5V [M+] 677.20; found 678.21 [M + H]+. C38H36N4O6V [VO(L3)2·H2O] (695.21): calcd C 65.61, H 5.22, N 8.05; found C 65.68, H 5.27, N 8.07.
Synthesis of complex VO(L4)2. The complex was synthesized by a similar procedure to VO(L1)2. Yield 0.245 g (68%). ESI-MS calcd for C34H22Cl4N4O5V [M]+ 756.98; found 757.91 [M + H]+. C34H24Cl4N4O6V [VO(L4)2·H2O] (774.99): calcd C 52.53, H 3.11, N 7.21; found C 52.58, H 3.15, N 7.20.
Synthesis of complex VO(L5)2. The complex was synthesized by a similar procedure to VO(L1)2. Yield 0.231 g (67%). ESI-MS calcd for C36H26Cl4N4O5V [M]+ 785.01; found 787.92 [M + 2H]+. C36H28Cl4N4O6V [VO(L5)2·H2O] (803.02): calcd C 53.69, H 3.50, N 6.96; found C 53.72, H 3.56, N 7.02.
Crystallography
The complex VO(L1)2 was dissolved in acetonitrile and heated till the solution become clear and then it was allowed to crystallize at room temperature. Blue crystals of single crystal X-ray diffraction quality were obtained within a week. The crystal used for data collection was of dimensions 0.3 × 0.2 × 0.2 mm3. The cell dimensions were determined by least-square fit of angular settings of 5156 reflections in the θ range 3.37–28.95°. The intensities were measured by ø and ω scan mode for θ ranges 3.37 to 26.00. Data were corrected for Lorentz, polarization and absorption factors. The structure was solved by direct methods using SHELXS97.13 All non-hydrogen atoms of the molecule were located in the best E-map. Full-matrix least-squares refinement was carried out using SHELXL97.13
All hydrogen atoms except water hydrogen were included as idealized atoms riding on the respective carbon atoms with C–H bond lengths appropriate to the carbon atom hybridization. Water hydrogen atoms were located from the difference Fourier map and included in the refinement. The final refinement cycles converged to an R = 0.0562 and wR(F2) = 0.1795 for the observed data. Residual electron densities ranged from −0.427 to 0.425 e Å−3. Atomic scattering factors were taken from International Tables for X-ray Crystallography (1992, vol. C, Tables 4.2.6.8 and 6.1.1.4). The crystallographic data are summarized in Table 1. H atoms are shown as small spheres of arbitrary radii. The geometry of the molecule was calculated using the WinGX14 and PARST15 software.
Table 1 Crystal structure data and structure refinement details for VO(L1)2
|
[VO(L1)2(H2O)]CH3CN |
Empirical formula |
C36H32N4O6V1·C2H3N1 |
Fw |
708.65 |
Wavelength (Å) |
0.71073 |
Crystal system |
Monoclinic |
Space group |
P21/c |
Crystal colour and size (mm) |
Blue, 0.3 × 0.2 × 0.2 |
a (Å) |
17.2079(7) |
b (Å) |
11.4667(5) |
c (Å) |
18.6877(8) |
α (°) |
90.00 |
β (°) |
105.196(4) |
γ (°) |
90.00 |
V (Å3) |
3558.5(3) |
Z |
4 |
Dcalcd (g/cm3) |
1.323 |
F(000) |
1476 |
θ range for data collection |
3.37 < θ < 26.00 |
Index ranges |
−14 ≤ h ≤ 21, −14 ≤ k ≤ 13, −23 ≤ l ≤ 17 |
Reflections collected/unique |
14753/6979 |
Refinement method |
Full-matrix least-squares on F2 |
Final R |
0.0522 |
Goodness-of-fit on F2 |
1.022 |
Final residual electron density (e Å−3) |
−0.428 < Δρ < 0.303 |
Catalytic reaction
Catalytic oxidation of benzyl alcohol was carried out in a 50 mL round bottom flask fitted with a water circulated condenser using oxovanadium(IV) complexes [VO(L1−5)2] of 4-acylpyrazolone ligands as a catalyst. In a typical reaction, benzyl alcohol, catalyst and 30% H2O2 were intensively stirred at 90 °C for the whole duration of the reaction. After completion of the reaction, the reaction mixture was concentrated on a rotary evaporator. Hexane was added to the system, and the organic phase was separated. By this simple procedure, isolation of the carbonyl product in the organic phase was achieved.
Results and discussion
Synthesis and characterization of oxovanadium(IV) complexes (1–5)
The ligands (L1 to L5) were synthesized according to a previously reported procedure.12,16 The ligands were characterized by 1H and 13C NMR and ESI-MS. The oxovanadium(IV) complexes here reported have the general composition [VO(L)2(H2O)]. The complexes (1–5) were synthesized by a general procedure based on the mixing of an ethanolic solution of ligand with an aqueous solution of VOSO4·5H2O in 2
:
1 molar ratio and isolation of the final product by filtration. The complexes were characterized by elemental analyses, FT-IR, TG-DTA, mass spectrometry, UV-Vis, EPR spectroscopy and single crystal XRD analysis. All the complexes were stable to air and moisture without any kind of decomposition even after several months. The complexes were insoluble in water, hexane and petroleum ether, but soluble in DMF, DMSO, acetonitrile and chlorinated solvents. The obtained complexes were designated as VO(L1−5)2.
Blue block-shaped single crystals of the complex VO(L1)2 suitable for X-ray diffraction analysis were obtained by slow evaporation of VO(L1)2 in acetonitrile. The crystal structure of the complex was solved by single-crystal XRD in the space group P21/c of the monoclinic system and refined to give the formula [VO(L1)2·H2O](CH3CN). Data collection parameters and refinement results are summarized in Table 1.
Single crystal data reveal that the two O,O-chelating acylpyrazolonate ligands and one water molecule are coordinated to the vanadium centre creating a distorted octahedral geometry. Thus both the ligands form a six membered chelate ring with the vanadium metal center. One acetonitrile molecule has also been trapped in the crystal lattice. An ORTEP view of the title complex with atomic labeling is shown in Fig. 1. It is reported from the crystal structure evidence that oxovanadium(IV) complexes of acylpyrazone ligands exist in octahedral geometry, where the ligands are coordinated in syn12 or anti17 conformations and the V–O(oxo) group is located trans to the coordinated water molecule. Somewhat surprisingly, in the present complex VO(L1)2, the coordination mode of ligands with the centre metal were changed, the V–O(oxo) group is located cis to the coordinated water molecule and the ligands are in twisted geometry.
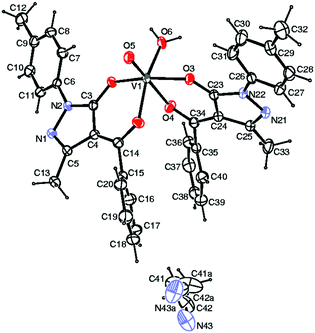 |
| Fig. 1 ORTEP view of the VO(L1)2 molecule with displacement ellipsoids drawn at 50% probability level. | |
The VIV has a distorted {O6} octahedral environment with six different V–O bond lengths. The shortest bond length (1.594(2) Å) was found for the oxovanadium bond (V1–O5). The longest bond length (2.205(2) Å) was found for the oxygen of the coordinated acylpyrazolone ligand and metal centre (V1–O4), located trans to the V–O(oxo) group. The middle range (1.982(2)–2.022(3) Å) was found for the oxygen atoms of coordinated acylpyrazolone ligands (V1–O1, V1–O2 and V1–O3) and coordinated water molecule (V1–O6). The bond length to the coordinated water molecule (V1–O6) was 2.022(3) Å, which is in contrast to the previously reported bond lengths in syn12 (2.247 Å) and anti17 (2.262 Å) configurations. The bond length V1–O4 was increased from 1.999 (syn) and 2.013 (anti) to 2.205(2). Bond length V1–O3 was also increased from 1.969 (syn) and 1.989 (anti) to 2.018(2) Å. Selected bond lengths and bond angles of [VO(L1)2] are listed in Table 2.
Table 2 Selected bond lengths (Å) and bond angles (°)
V1–O1 |
1.982(2) |
V1–O4 |
2.205(2) |
V1–O2 |
2.021(2) |
V1–O5 |
1.594(2) |
V1–O3 |
2.018(2) |
V1–O6 |
2.022(3) |
|
O1–V1–O2 |
91.06(9) |
O2–V1–O3 |
88.48(9) |
O3–V1–O4 |
83.19(8) |
O3–V1–O6 |
89.1(1) |
O5–V1–O6 |
98.8(1) |
O4–V1–O6 |
83.0(1) |
O1–V1–O4 |
82.22(8) |
O1–V1–O5 |
99.1(1) |
O1–V1–O6 |
86.8(1) |
O2–V1–O5 |
99.0(1) |
O2–V1–O6 |
162.1(1) |
O3–V1–O5 |
95.5(1) |
O2–V1–O4 |
79.13(9) |
O4–V1–O5 |
177.8(1) |
O1–V1–O3 |
165.23(9) |
|
|
Examination of nonbonded contacts reveals O–H⋯N and C–H⋯O intermolecular hydrogen bonds (Table 3), which are responsible for the stability of the molecules within the unit cell. The H atoms of a coordinated water molecule are creating two hydrogen bonds, one with the N atom of the acetonitrile molecule and another one with the N atom of the pyrazolone ring of another molecule. Due to the hydrogen bonding geometry, the molecule has been twisted from its usual geometry (syn or anti).
Table 3 Hydrogen bonding geometrya
D–H⋯A |
D–H (Å) |
D⋯A (Å) |
H⋯A (Å) |
DH⋯A (°) |
Symmetry codes: (i) −x + 2, −y, −z + 1, (ii) x, +y − 1, +z, (iii) x, −y − 1/2, +z − 1/2. |
O6–H61⋯N21i |
0.82(1) |
2.744(3) |
1.94(1) |
169(1) |
C41–H41C⋯O2ii |
0.96 |
3.210(7) |
2.579(2) |
123.4(4) |
C38–H38⋯O5ii |
0.93 |
3.416(5) |
2.543(2) |
156.5(3) |
C41–H41B⋯O5ii |
0.96 |
3.097(7) |
2.427(2) |
126.6(4) |
O6–H62⋯N43iii |
0.81(2) |
2.665(9) |
1.87(2) |
168(2) |
A comparison with the published crystal structure data for other oxovanadium(IV) complexes of acylpyrazolone ligands shows that the O5–V1–O6 angle within the VO(L1)2 complex has been decreased from 175–179° to 98.8(1)°. The angle O1–V1–O3 has been increased from 87.4–87.9° to 165.23(9)°, while the angle O2–V1–O4 has been decreased from 89.5–89.76° to 79.13(9)°.12,17 It is obvious from the crystal structure data that the geometry of the VO(L1)2 differs from the previous reports. The acylpyrazolone ligands are coordinated with a vanadium atom in a twisted form to create a distorted octahedral geometry, where the two oxygen donors of two acylpyrazolones constitute a six-membered ring with the metal atom. The resulting bite angles O1–V1–O2 and O3–V1–O4 are 91.06(9)° and 83.19(8)°, respectively.
The mass spectrum of VO(L1)2 shows a molecular ion peak (C36H30N4O5V) at m/z = 550.0336 [M + H]+, which indicate that it is a mono vanadium species having two 1-toluoyl-3-methyl-4-phenyl-5-pyrazolone ligands. The base peak at m/z = 293.164 is attributed to ligand L1 [M + H]+. Fragmentation of weakly coordinated water/solvent molecules in ESI mass spectrum is not observed. Mass spectra of complexes VO(L2)2, VO(L3)2, VO(L3)2 and VO(L5)2 showed molecular ion peaks at m/z: 650.03 [M + H]+, 678.21 [M + H]+, 757.91 [M + H]+ and 787.92 [M + 2H]+, respectively, which are in good agreement with the proposed structures. FT-IR spectra of the complexes show absorptions between 1565 to 1585 cm−1 and 1475–1497 cm−1 which are assigned to νC
N of the pyrazolone ring and νC–O, respectively. Additional information from IR spectra arises from the strong band observed in the range 945–970 cm−1 due to νV
O stretching. The bands within 470–485 cm−1 are assigned to the νV–O stretching. The IR spectra of complexes exhibit a band in the range 3390–3435 cm−1, which can be assigned to the coordinated water molecule. The oxovanadium complexes show three absorption bands in the electronic spectra. The bands in the range 392–401 nm were assigned to ligand to metal charge transfer (LMCT). The two bands in the ranges 616–632 nm and 772–783 nm were assigned to d → d transitions. The electronic spectra reveal that in the complexes vanadium is present in +4 oxidation state.18
An EPR spectrum of a frozen (77 K) solution (in DMF) of VO(L1)2 was recorded. The spectrum of VO(L1)2 is a 8-line pattern of free electron (3d1) with the magnetic nuclear moment of 51V (I = 7/2), which shows that a single vanadium is present in the molecule, i.e., it is mononuclear. The values of giso = 1.981 and Aiso = 104.5 × 10−4 confirm the presence of vanadium (V4+, 3d1) in distorted octahedral geometry of the complex. The g value was computed from the spectrum using a tetracyanoethylene free radical as the g marker.
Catalytic activity studies
The oxidation of benzyl alcohol catalyzed by oxovanadium(IV) complexes was carried out using H2O2 as an oxidant to give benzaldehyde, benzoic acid and benzyl benzoate as products. The formation of all these products is represented by Scheme 2. These are common products and have been identified by others as well.8,10d
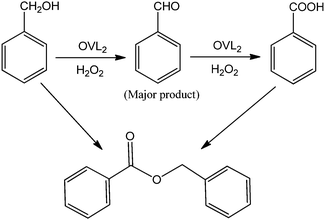 |
| Scheme 2 Representation of benzyl alcohol oxidation and its products. | |
The optimization was carried out by varying different parameters, such as molar ratio of H2O2, amount of catalyst, reaction time and solvent. The progress of the reaction was monitored by GC-MS analysis and products formed in the reactions were matched with those reported in the literature. The complex VO(L1)2 was chosen for the optimization. The optimizations of catalyst amount, reaction time and molar ratio of H2O2 were done without solvent.
Effect of catalyst amount
The amount of catalyst has a significant effect on the oxidation of benzyl alcohol. Four different amounts of catalyst, viz. 0.015, 0.03, 0.045 and 0.06 mmol, were used, keeping all other parameters fixed: namely, temperature (90 °C), benzyl alcohol (10 mmol), 30% H2O2 (30 mmol) and reaction time (24 h). The results are shown in Fig. 2, indicating 68, 78, 93 and 94% conversion corresponding to 0.015 mmol, 0.03 mmol, 0.045 mmol and 0.06 mmol, respectively. The maximum percentage conversion was observed with 0.06 mmol catalyst but there was no remarkable difference in the reaction when 0.045 or 0.06 mmol catalyst was employed. Therefore, 0.045 mmol catalyst was taken to be optimum.
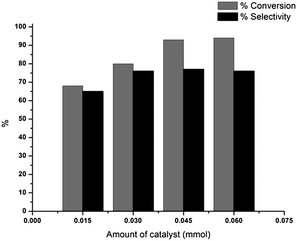 |
| Fig. 2 Effect of amount of catalyst; % conversion is based on benzyl alcohol and % selectivity with respect to benzaldehyde; reaction time = 24 h, temperature = 90 °C, benzyl alcohol = 10 mmol and H2O2 = 30 mmol. | |
Effect of H2O2 concentration
In order to determine the effect of H2O2 on the oxidation of benzyl alcohol to benzaldehyde, we studied using four different benzyl alcohol
:
H2O2 molar ratios (1
:
1, 1
:
2, 1
:
3 and 1
:
4) keeping other parameters fixed: namely, catalyst (0.045 mmol), temperature (90 °C) and reaction time (24 h). Benzyl alcohol to H2O2 molar ratios of 1
:
1 and 1
:
2 resulted in 24 and 53% conversion, respectively. When the benzyl alcohol to H2O2 molar ratio was changed to 1
:
3, the conversion increased to nearly 93%, keeping all other conditions similar. A further increase in the ratio to 1
:
4 resulted in a slight decrease in the conversion to 88% (Fig. 3). The reason for this may be due to the dilution of the reaction mixture by the presence of larger amounts of water molecules in H2O2 solution.
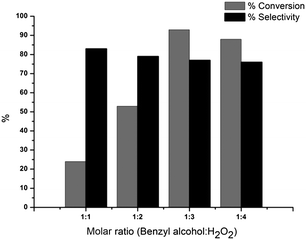 |
| Fig. 3 Effect of H2O2 concentration: % conversion is based on benzyl alcohol and % selectivity with respect to benzaldehyde; reaction time = 24 h, temperature = 90 °C, benzyl alcohol = 10 mmol and catalyst = 0.045 mmol. | |
Effect of reaction time
The time dependence of catalytic oxidation of benzyl alcohol was studied by performing the reaction of benzyl alcohol (10 mmol) with 30% H2O2 (30 mmol) in the presence of 0.045 mmol catalyst at 90 °C with constant stirring. It is seen from Fig. 4 that with an increase in reaction time, the % conversion also increases. Initial conversion of benzyl alcohol increased with the reaction time. This is due to the reason that more time is required for the formation of the reactive intermediate (substrate + catalyst) which is finally converted into the products. It was seen that 93% conversion was observed at 24 h; when the reaction was allowed to continue for more than 24 h, no appreciable changes in conversion or selectivity were observed.
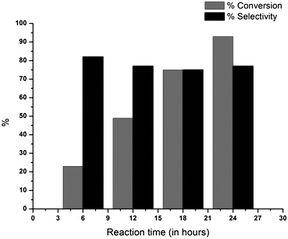 |
| Fig. 4 Effect of reaction time: % conversion is based on benzyl alcohol and % selectivity with respect to benzaldehyde; amount of catalyst = 0.045 mmol, temperature = 90 °C, benzyl alcohol = 10 mmol and H2O2 = 30 mmol. | |
Effect of solvent
The selectivity in oxidation of benzyl alcohol to benzaldehyde is always an issue in this experiment. To avoid or minimize the side reactions we carried out optimization in different solvent systems keeping other parameters fixed: namely, catalyst amount (0.045 mmol), reaction temperature (90 °C), benzyl alcohol (10 mmol), 30% H2O2 (30 mmol), reaction time (24 h) and solvent (5 mL). The reactions were carried out in toluene, chloroform, DMF, methanol, hexane, acetonitrile and cyclohexane (Fig. 5). DMF, methanol and chloroform do not lead to a remarkable % conversion of benzyl alcohol; however, toluene and hexane have some influence on the outcome of the reaction and lead to 57% and 54% conversion with 76% and 62% selectivity, respectively. The best results are obtained with acetonitrile which afforded a remarkable conversion (92%) of benzyl alcohol into benzaldehyde with 80% selectivity.
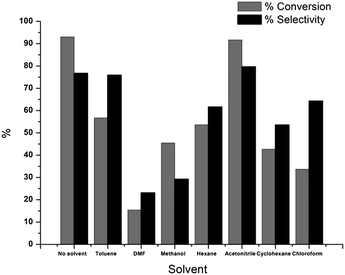 |
| Fig. 5 Effect of solvent: % conversion is based on benzyl alcohol and % selectivity with respect to benzaldehyde; reaction time = 24 h, temperature = 90 °C, benzyl alcohol = 10 mmol H2O2 = 30 mmol and catalyst = 0.045 mmol, solvent = 5 mL. | |
Thus, the optimum conditions for the maximum % conversion and selectivity for the oxidation of benzyl alcohol to benzaldehyde are as follows: reaction temperature (90 °C), benzyl alcohol (10 mmol), 30% H2O2 (30 mmol), catalyst amount (0.045 mmol), reaction time (24 h) and acetonitrile (5 mL) as a solvent.
Another goal of this work was to compare the catalytic activities of oxovanadium(IV) complexes of various derivatives of 4-acylpyrazolone ligands. Thus, all the synthesized complexes were used as catalysts for the oxidation of benzyl alcohol under the same optimized conditions. From the data reported in Table 4, we observed that VO(L1)2, VO(L2)2, VO(L4)2 and VO(L5)2 showed 92%, 91%, 91% and 92% conversion, respectively. However, VO(L3)2 showed a small decrease in conversion (86%) in comparison to the other catalysts. Among these catalysts VO(L1)2 showed good selectivity (80%) for benzaldehyde in comparison to catalysts VO(L3)2, VO(L4)2 and VO(L5)2. The catalyst VO(L2)2 resulted in 77% selectivity which is comparable with the selectivity obtained with VO(L1)2. The results show that selectivity decreases on increasing the amount of substitution over the phenyl ring of acylpyrazolone ligands in their oxovanadium(IV) complexes. As VO(L1)2 shows better catalytic performance resulting in higher conversion and selectivity compared to other complexes, further investigations were carried out using VO(L1)2 as an oxidizing catalyst.
Table 4 Oxidation of benzyl alcohol using various catalysts [oxovanadium(IV) complexes 1–5]
Catalyst |
% Conversion |
% Selectivity |
TON |
VO(L1)2 |
92 |
80 |
160 |
VO(L2)2 |
91 |
77 |
156 |
VO(L3)2 |
86 |
66 |
132 |
VO(L4)2 |
91 |
68 |
146 |
VO(L5)2 |
92 |
68 |
169 |
To explore the scope of the present catalyst VO(L1)2 to act as an oxidizing catalyst, the reactions of a wide array of substituted benzyl alcohols were also conducted under the same optimized conditions. The results of the oxidation of various benzylic alcohols to the corresponding aldehydes are shown in Table 5.
Table 5 Oxidation of alcohols using VOL2 catalysta
No. |
Substrate |
Product |
% Conversion |
% Selectivity |
TON |
Reaction conditions: catalyst = 0.045 mmol, benzylic alcohol = 10 mmol, 30% H2O2 = 30 mmol, acetonitrile solvent = 5 mL, temperature = 90 °C and reaction time = 24 h. |
1 |
Benzyl alcohol |
Benzaldehyde |
92 |
80 |
160 |
2 |
2-Methoxy benzyl alcohol |
2-Methoxy benzaldehyde |
66 |
85 |
120 |
3 |
3-Methoxy benzyl alcohol |
3-Methoxy benzaldehyde |
72 |
100 |
160 |
4 |
4-Methoxy benzyl alcohol |
4-Methoxy benzaldehyde |
94 |
100 |
209 |
5 |
4-Nitro benzyl alcohol |
4-Nitro benzaldehyde |
63 |
79 |
112 |
6 |
4-Methyl benzyl alcohol |
4-Methyl benzaldehyde |
90 |
100 |
199 |
7 |
2-Chloro benzyl alcohol |
2-Chloro benzaldehyde |
49 |
100 |
109 |
8 |
3-Chloro benzyl alcohol |
3-Chloro benzaldehyde |
40 |
100 |
89 |
The reactions of benzylic alcohols (entries 1–8) bearing electron-donating and electron-withdrawing substituents on the aromatic ring proceeded smoothly to give the corresponding aldehydes in good to high conversion as well as selectivity. The conversion with 4-methoxy benzyl alcohol was comparatively higher than those of 3-methoxy and 2-methoxy benzyl alcohol, and the selectivity for 4-methoxy benzaldehyde is 100%. The conversion of 4-methoxy benzyl alcohol was observed to be slightly higher than that of benzyl alcohol under identical experimental conditions. Conversion of 4-methyl benzyl alcohol is also comparable with that of 4-methoxy benzyl alcohol with 100% selectivity. The conversion of 4-nitro benzyl alcohol was lower in comparison with benzyl alcohol and methoxy derivatives of benzyl alcohol. 2-Chloro and 3-chloro benzyl alcohol show poor conversion but high selectivity for their corresponding aldehydes. The catalyst shows a higher turnover number (TON) for 4-methoxy benzyl alcohol while the lowest TON is obtained for 3-chloro benzyl alcohol.
Possible pathway of the catalyzed reactions
Several mechanisms have been proposed in the literature for the oxidation of alcohols catalyzed by vanadium complexes.7a,19 To establish the possible pathway of the present reaction and to evaluate intermediate species formed during catalytic oxidation, a 3 mmol solution of VO(L1)2 was prepared in acetonitrile and its electronic spectrum was recorded using a UV-Vis spectrophotometer. The electronic spectral data of the neat complex exhibit a band at 393 nm, which was assigned to ligand to metal charge transfer (LMCT). The lower energy and less intense bands appearing at 617 and 772 nm were assigned to d → d transitions. After that 30% H2O2 was added to the solution of VO(L1)2. The color of the solution rapidly changes from green to brown due to the change in the oxidation state of vanadium from +4 to +5. The electronic spectrum of this solution exhibits only one band at 398 nm (LMCT). As VV complexes have 3d0 configuration, d → d transitions are not expected to appear in the electronic spectrum (Fig. 6).
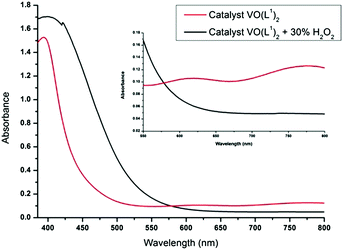 |
| Fig. 6 UV-Vis spectra of neat catalyst VO(L1)2 (in red) and catalyst VO(L1)2 with 30% H2O2 (in black). | |
The mechanism of alcohol oxidation catalyzed by VO(L1)2 may be viewed as an oxidative dehydrogenation involving the formation of a high valent peroxovanadium intermediate. The electronic spectra results show that a higher vanadium(V) species might be produced and for its formation, the presence of H2O2 is necessary.20 Thus, based on UV-Vis study and literature reports it is proposed that first, a vanadium(IV) species reacts with H2O2 to form a vanadium(V) species. This species reacts with benzylic alcohol to afford a vanadium alcoholate. Next, the product carbonyl compound is formed, and the vanadium(IV) species is reproduced.
Conclusions
A series of novel oxovanadium(IV) complexes with a variety of different substituted 4-acylpyrazolone ligands were synthesized, and characterized by various analytical and spectroscopic techniques. The geometry and molecular structure of the complex [VO(L1)2·H2O] were resolved by single-crystal XRD. Interestingly, different results were obtained compared with the previous reported geometries of the oxovanadium(IV) complexes of acylpyrazolone ligands. The coordination mode of ligands with the metal centre has changed and the V–O(oxo) group is located cis to the coordinated water molecule, thus forming a twisted octahedral structure with the metal centre. The synthesized oxovanadium(IV) complexes were used for the oxidation of benzyl alcohol using H2O2 as an oxidant and proved to be successful as oxidation catalysts. Various parameters such as catalyst amount, solvent, H2O2 concentration and reaction time were tested in order to optimize both the conversion and the selectivity. Under optimized conditions, VO(L1)2 provided high values of conversion (92%) and selectivity (80%) for benzaldehyde compared to the other complexes studied. The catalyst shows the high catalytic conversion and selectivity towards benzyl alcohol and substituted benzylic alcohols (4-methoxy and 4-methyl benzyl alcohols). To the best of our knowledge, the present catalytic system is the first example of the oxidation of alcohols by an oxovanadium(IV) complex of acylpyrazolone ligand. Mechanistic details were provided for the oxidation of benzylic alcohols by the oxovanadium(IV) complex using H2O2 as an oxidant.
Acknowledgements
The authors are thankful to the University Grants Commission, New Delhi, India, for financial assistance for this work in terms of a major research project [Project F.37-376/2009 12.01.2010(SR)] to R. N. Jadeja. S. Parihar is thankful to UGC-BSR, New Delhi, India, for financial assistance. They are also thankful to the Head of the Department of Chemistry for providing the necessary facilities required to carry out this work.
Notes and references
-
(a) D. Rehder, Coord. Chem. Rev., 1999, 182, 297–322 CrossRef;
(b) Y. Shechter and S. J. D. Karlish, Nature, 1980, 284, 556–558 CrossRef CAS;
(c) D. Rehder, Bioinorganic Vanadium Chemistry, John Wiley & Sons, Chichester, U.K., 2008 Search PubMed;
(d) R. R. Eady, Coord. Chem. Rev., 2003, 237, 23–30 CrossRef CAS;
(e) K. H. Thompson and C. Orvig, Coord. Chem. Rev., 2001, 219–221, 1033–1053 CrossRef CAS;
(f) I. Osinska-Krolicka, H. Podsiadly and A. Bukietynska, J. Inorg. Biochem., 2004, 98, 2087–2098 CrossRef CAS PubMed.
-
(a) T. Hirao, Chem. Rev., 1997, 97, 2707–2724 CrossRef CAS PubMed;
(b) C. Bolm, Coord. Chem. Rev., 2003, 237, 245–256 CrossRef CAS;
(c) A. G. J. Ligtenbarg, R. Hage and B. L. Feringa, Coord. Chem. Rev., 2003, 237, 89–101 CrossRef CAS;
(d) S. Takizawa, T. Katayama and H. Sasai, Chem. Commun., 2008, 4113–4122 RSC;
(e) F. Cavani, N. Ballarini and A. Cericola, Catal. Today, 2007, 127, 113–131 CrossRef CAS PubMed;
(f) S. K. Hanson, R. Wu and L. A. P. Silks, Org. Lett., 2011, 13, 1908–1911 CrossRef CAS PubMed;
(g) N. Mizuno and K. Kamata, Coord. Chem. Rev., 2011, 255, 2358–2370 CrossRef CAS PubMed.
-
(a) H.-L. Wu and B.-J. Uang, Tetrahedron: Asymmetry, 2002, 13, 2625–2628 CrossRef CAS;
(b) M. Bühl, R. Schurhammer and P. Imho, J. Am. Chem. Soc., 2004, 126, 3310–3320 CrossRef PubMed;
(c) M. L. Kuznetsova and J. C. Pessoa, Dalton Trans., 2009, 5460–5468 RSC.
-
(a) G. Santoni, G. Licini and D. Rehder, Chem.–Eur. J., 2003, 9, 4700–4708 CrossRef CAS PubMed;
(b) C. Drago, L. Caggiano and R. F. W. Jackson, Angew. Chem., Int. Ed., 2005, 44, 7221–7223 CrossRef CAS PubMed;
(c) A. Basak, A. U. Barlan and H. Yamamoto, Tetrahedron: Asymmetry, 2006, 17, 508–511 CrossRef CAS PubMed;
(d) S.-H. Hsieh, Y.-P. Kuo and H.-M. Gau, Dalton Trans., 2007, 97–106 RSC;
(e) S. L. Jain, B. S. Rana, B. Singh, A. K. Sinha, A. Bhaumik, M. Nandi and B. Sain, Green Chem., 2010, 12, 374–377 RSC.
-
(a) M. R. Maurya, U. Kumar, I. Correia, P. Adão and J. C. Pesoa, Eur. J. Inorg. Chem., 2008, 577–587 CrossRef CAS;
(b) M. R. Maurya, A. Arya, U. Kumar, A. Kumar, F. Avecilla and J. C. Pessoa, Dalton Trans., 2009, 9555–9566 RSC.
-
(a) P. J. Figiel, J. M. Sobczak and J. J. Ziólkowski, Chem. Commun., 2004, 244–245 RSC;
(b) T. Waters, G. N. Khairallah, S. A. S. Y. Wimala, Y. C. Ang, R. A. J. O'Hair and A. G. Wedd, Chem. Commun., 2006, 4503–4505 RSC;
(c) S. Kodama, Y. Ueta, J. Yoshida, A. Nomoto, S. Yano, M. Ueshima and A. Ogawa, Dalton Trans., 2009, 9708–9711 RSC;
(d) A. Dewan, T. Sarma, U. Bora and D. K. Kakati, Tetrahedron Lett., 2011, 52, 2563–2565 CrossRef CAS PubMed.
-
(a) A. Butler, M. J. Clague and G. E. Meister, Chem. Rev., 1994, 94, 625–638 CrossRef CAS;
(b) V. Conte, F. Di Furia and S. Moro, J. Phys. Org. Chem., 1996, 9, 329–336 CrossRef CAS.
-
(a) C. Li, P. Zheng, J. Li, H. Zheng, Y. Cui, Q. Shao, X. Ji, J. Zheng, P. Zhao and Y. Xu, Angew. Chem., Int. Ed., 2003, 42, 5063–5066 CrossRef CAS PubMed;
(b) A. Jia, L.-L. Lou, C. Zhang, Y. Zhang and S. Liu, J. Mol. Catal. A: Chem., 2009, 306, 123–129 CrossRef CAS PubMed;
(c) A. Podgoršek, M. Zupan and J. Iskra, Angew. Chem., Int. Ed., 2009, 48, 8424–8450 CrossRef PubMed;
(d) V. K. Bansal, P. P. Thankachan and R. Prasad, Appl. Catal., A, 2010, 381, 8–17 CrossRef CAS PubMed;
(e) G. D. Faveri, G. Ilyashenko and M. Watkinson, Chem. Soc. Rev., 2011, 40, 1722–1760 RSC.
-
(a) Peroxide Chemistry. Mechanistic and Preparative Aspects of Oxygen Transfer, ed. W. Adam, Wiley-VCH Verlag GmbH, Weinheim, 2000 Search PubMed;
(b) The Chemistry of Peroxides-Transition Metal Peroxides. Synthesis and Role in Oxidation Reactions, ed. V. Conte, O. Bortolini and Z. Rappoport, Wiley Interscience, 2006, p. 1053, and references cited therein Search PubMed.
-
(a) R. A. Sheldon, I. W. C. E. Arends, G. J. Ten Brink and A. Dijksman, Acc. Chem. Res., 2002, 35, 774–781 CrossRef CAS PubMed;
(b) K. Yamaguchi and N. Mizuno, Chem.–Eur. J., 2003, 9, 4353–4361 CrossRef CAS PubMed;
(c) A. Dhakshinamoorthy, M. Alvaro and H. Garcia, ACS Catal., 2011, 1, 48–53 CrossRef CAS;
(d) R. Kwahara, K.-I. Fujita and R. Yamaguchi, J. Am. Chem. Soc., 2012, 134, 3643–3646 CrossRef PubMed.
-
(a) H.-J. Arpe, Ulmann's Encyclopedia of Industrial Chemistry, 5th edn, VCH, Weinheim, 1985, p. 469 Search PubMed;
(b) V. R. Choudhary, D. K. Dumbre, V. S. Narkhede and S. K. Jana, Catal. Lett., 2003, 86, 229–233 CrossRef CAS.
- S. Parihar, S. Pathan, R. N. Jadeja, A. Patel and V. K. Gupta, Inorg. Chem., 2012, 51, 1152–1161 CrossRef CAS PubMed.
- G. M. Sheldrick, SHELX97, University of Göttingen, Göttingen, Germany, 1997 Search PubMed.
- L. J. Farrugia, J. Appl. Crystallogr., 1999, 32, 837–838 CrossRef CAS.
- M. Nardelli, J. Appl. Crystallogr., 1995, 28, 659 CrossRef CAS.
-
(a) R. J. Yadav, K. M. Vyas and R. N. Jadeja, J. Coord. Chem., 2010, 63, 1820–1831 CrossRef CAS;
(b) R. N. Jadeja, S. Parihar, K. Vyas and V. K. Gupta, J. Mol. Struct., 2012, 1013, 86–94 CrossRef CAS PubMed.
- F. Marchetti, C. Pettinari, C. D. Nicola, R. Pettinari, A. Crispini, M. Crucianelli and A. D. Giuseppe, Appl. Catal., A, 2010, 378, 211–233 CrossRef CAS PubMed.
- R. N. Jadeja and J. R. Shah, Polyhedron, 2007, 26, 1677–1685 CrossRef CAS PubMed and references cited therein.
-
(a) Y. Maeda, N. Kakiuchi, S. Matsumura, T. Nishimura, T. Kawamura and S. Uemura, J. Org. Chem., 2002, 67, 6718–6724 CrossRef CAS PubMed;
(b) S. R. Reddy, S. Das and T. Punniyamurthy, Tetrahedron Lett., 2004, 45, 3561–3564 CrossRef CAS PubMed;
(c) S. K. Hanson, R. T. Baker, J. C. Gordon, B. L. Scott, L. A. Silks and D. L. Thorn, J. Am. Chem. Soc., 2010, 132, 17804–17816 CrossRef CAS PubMed;
(d) V. Conte, A. Coletti, B. Floris, G. Licini and C. Zonta, Coord. Chem. Rev., 2011, 255, 2165–2177 CrossRef CAS PubMed.
-
(a) D. Rehder, M. Ebel, C. Wikete, G. Santoni and J. Gätjens, Pure Appl. Chem., 2005, 77, 1607–1616 CrossRef CAS;
(b) M. R. Maurya, A. K. Chandrakar and S. Chand, J. Mol. Catal. A: Chem., 2007, 270, 225–235 CrossRef CAS PubMed;
(c) M. R. Maurya, A. K. Chandrakar and S. Chand, J. Mol. Catal. A: Chem., 2007, 274, 192–201 CrossRef CAS PubMed.
Footnote |
† Electronic supplementary information (ESI) available: Fig. S1 to S26. CCDC reference number 859107 (for complex VO(L1)2). For ESI and crystallographic data in CIF or other electronic format see DOI: 10.1039/c3ra46896h |
|
This journal is © The Royal Society of Chemistry 2014 |