DOI:
10.1039/C3RA46673F
(Paper)
RSC Adv., 2014,
4, 7760-7765
Comparison of the gelation behaviour of N-substituted tetradecanamide amphiphiles in organic liquids: effect of hydrogen-bonding ability of the head-group
Received
13th November 2013
, Accepted 3rd January 2014
First published on 6th January 2014
Abstract
In this work, we have investigated the role of intermolecular hydrogen-bonding (H-bonding) interaction of the head-groups of amphiphilic organogelators. For this we have designed, synthesized and studied the gelation behaviour of a series of amide amphiphiles having hydrocarbon chains with the same length (C14) but with –COOH, –OH, –NH2 or –N(CH3)2) functionalities as head-group. These gelators, except the one with –N(CH3)2 head-group, efficiently gelated aromatic solvents. The gelation in all the solvents employed was observed to be thermoreversible. The gels were characterized by XRD spectroscopy, electron microscopy, and rheology. The amphiphiles were observed to form ribbon-like aggregates in aromatic solvents. The gel-to-sol transition temperatures as well as mechanical strengths of the organogels were observed to increase with the spacer length. The results suggest that the intermolecular H-bonding interactions between head-groups were essential for gelation.
1. Introduction
In recent literature, over the last three decades, low-molecular-weight gelators (LMWGs) have attracted a great deal of attention because of their impacts in various fields including molecular self-assembly (SA), smart materials, and controlled drug release.1–13 These gelators form gels via formation of a network of fibers by different non-covalent interactions, for example, ion–ion, H-bonding, π–π stacking, van der Waals forces etc. In most of the cases of organogelation, it has been reported that H-bonding is the dominant factor. For the gelation of organic liquids by amide compounds, such as amino acids,14–18 urea,19–21 and sugars22–25 gelator–gelator H-bonding interaction is responsible. Previously it was reported that N-alkanoyl-L-alanine amphiphiles gelate a series of organic solvents26,27 in which the driving force for gelation process was the amide H-bonding between two adjacent molecules. On the other hand, a rare example of organogelation by some amino-acid based gelators containing urea linkage which gelate organic liquids in the presence of a small amount of H-bonding additives (known to destroy the network structure via formation of competitive H-bonding) such as water, methanol, urea, acetonitrile, and tetrahydrofuran was reported by us.28 The same gelators, however, failed to gelate organic liquids in the presence of tetrabutylammonium fluoride (TBAF), which is known to be a strong H-bond breaker. This means that when H-bonds are completely broken, gel structure is destroyed.
The H-bonding at the head-group of an amphiphile also modulates the gelation capacity of the amphiphile. Thus ester derivatives of N-alkanoyl-L-alanine, where the –COOH group has been replaced by a methyl ester group, cannot gelate organic solvent due to the lack of H-bonding interaction at the head-group, which leads to the formation of a three-dimensional (3D) network structure forming gel.27 On the other hand, N-2-hydroxyethyl dodecanamide (NHD), a long-chain amide amphiphile with an alcohol (–OH) group at one end of the molecule has been shown to form thixotropic organogel in toluene solvent.29 Recently, we have shown that N-2-carboxyethyl tetradecanamide (NCT) having a –COOH group at the end also acts as a good oganogelator.30 Thus it is evidenced that H-bonding interactions at the head-group of this class of amphiphiles also play an important role in the gelation process. In contrast, we have shown that the methyl ester of N-(n-tetradecylcarbamoyl)-L-alanine gelates organic solvents,28 which mean H-bonding at the head-group is not essential for gelation. Therefore, one can conclude that perhaps failure to gel organic solvents by N-alkanoyl-L-alanine methyl ester amphiphile could be due to steric hindrance at the amino acid head-group, which prevents close approach of the molecules and thus inhibits amide H-bonding.
In order to examine the role of H-bonding at the head-group of the amphiphile on the gelation process, we have designed and synthesized three structurally similar amphiphiles in which the –COOH group of NCT has been replaced by a primary amine (–NH2), a tertiary amine (–N(CH3)2), or a hydroxyl (–OH) group to give N-2-aminoethyl tetradecanamide (NAT), N-(2-N′,N′-dimethylaminoethyl) tetradecanamide (NDMAT), and N-2-hydroxyethyl tetradecanamide (NHT). The chemical structures of the amphiphiles are shown in Fig. 1. Though a number of amphiphilic molecules derived from diaminoethane have been synthesized31–40 by others, especially for various commercial applications, mono-chain derivatives of diaminoethane (where there is an –NH2 group at the end of the amphiphile) acting as LMWGs are not reported. The –NH2 group is a poor H-bond donor but a strong H-bond acceptor. On the other hand, –N(CH3)2 though can act as strong acceptor of H-bond, but it cannot donate any H-bond. Therefore, the gelation behavior of NAT, NHT, and NDMAT amphiphiles in four selected aromatic solvents was studied and compared with those of NCT. The organogels were characterized by scanning electron microscopy (SEM), X-ray diffraction (XRD), and rheology.
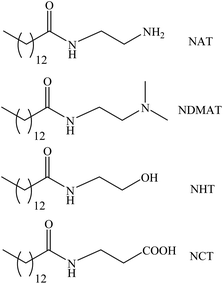 |
| Fig. 1 Chemical structure of substituted tetradecanamides. | |
2. Experimental sections
2.1 Materials
Tetradecanoyl chloride, 2-aminoethanol, 1,2-diaminoethane (DAE), N,N-dimethyldiaminoethane (DMAE), tetrabutyl-ammonium fluoride (TBAF) and the deuterated solvents, such as CDCl3 were procured from Sigma-Aldrich, (Bangalore, India). Analytical grade hydrochloric acid and triethylamine (TEA) were procured from SRL, Mumbai. These reagents were used directly from the bottle. The organic solvents, such as benzene (PhH), p-xylene (p-Ph(Me)2), chlorobenzene (PhCl), nitrobenzene (PhNO2), were of highest purity commercially available and used directly from the bottle. Ethanol (EtOH), chloroform (CHCl3) and dichloromethane (DCM) were of good quality commercially available and were dried and distilled fresh before use. The synthesis and chemical identification of NCT has been described in our previous publication.30 All other amphiphiles employed in this study were synthesized in the laboratory as described below.
Synthesis of N-2-aminoethyl tetradecanamides (NAT). The compounds were synthesized according to the procedure described in the literature.31 Briefly, quantitative purified DAE mixed in DCM in presence of TEA (2 eq.) was placed in a round-bottomed flask, and then stirred efficiently (Scheme 1). The corresponding acyl chloride (1 eq.) mixed in DCM was dropped for 30 min at room temperature. After 6 h, the mixture was filtered. The white solid was recrystallized three times with 1
:
1 EtOH/water mixture. Chemical structure of the amphiphiles was determined by FT-IR, 1H-NMR spectra, and elemental analysis.
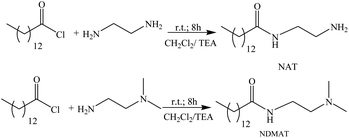 |
| Scheme 1 Reaction scheme for the synthesis of NAT and NDMAT amphiphiles. | |
N-2-Aminoethyl tetradecanamide (NAT). Yield 78%; m.p. 119–120 °C; FT-IR (KBr, cm−1) 3440 (primary NH2), 3290 (amide A), 1637 (amide-I), 1563 (amide-II); 1H-NMR: δH in ppm (400 MHz, CDCl3) 0.96 (3H, t, CH3), 1.30 (20H, m, alkyl chain), 1.57 (2H, m, CH2CH2CO), 2.18 (2H, t, CH2CH2CO), 2.91 (2H, t, CH2CH2NH2), 3.46 (2H, t, CH2CH2NH2); CHN Analysis: calcd. for C16H34N2O C: 71.06%, H: 12.67%, N: 10.36%; Found C: 71.20%, H: 12.50%, N: 10.30%.
N-2-N′,N′-Dimethylaminoethyl tetradecanamide (NDMAT). Yield 75%; FT-IR (KBr, cm−1) 3392 (amide A), 1698 (amide-I), 1585 (amide-II); 1H-NMR: δH in ppm (CDCl3, ppm) 0.84 (3H, t, CH3), 1.25 (20H, m, alkyl chain), 1.58 (2H, m, CH2CH2CO), 2.12 (2H, t, CH2CH2CO), 2.20 (6H, s, N(CH3)2), 2.41 (2H, t, CH2CH2N(CH3)2), 3.30 (2H, t, CONHCH2); CHN Analysis: calcd. for C18H38N2O C: 72.42%, H: 12.83%, N: 9.38%; Found C: 72.31%, H: 12.71%, N: 9.27%.
Synthesis of N-2-hydroxyethyl tetradecanamide (NHT). Amino ethanol (1 eq.) and TEA (2 eq.) were dissolved in dry CHCl3 and the mixture was cooled to 0–5 °C. After 10 min of cooling tetradecanoyl chloride (1 eq.) was added in dropwise fashion. After 16 h of stirring CHCl3 was evaporated to get a white mass that was purified by recystallisation from EtOH/water mixture. Chemical structure of the amphiphiles was determined by FT-IR and 1H-NMR spectra (Scheme 2).
 |
| Scheme 2 Reaction scheme for the synthesis of NHT amphiphile. | |
N-2-Hydroxyethyl tetradecanamide (NHT). Yield 81%, m.p. 73–76 °C; FT-IR (KBr, cm−1) 3337 (amide A), 1618 (amide-I), 1594 (amide-II); 1H NMR: δH in ppm (CDCl3, ppm) 0.87 (3H, t, CH3), 1.244 (20H, m, alkyl chain), 1.619 (2H, t, CH2CH2CO), 2.209 (2H, t, CH2CH2CO), 3.432 (2H, t, CH2CH2OH), 3.726 (2H, t, CH2CH2OH). CHN Analysis: calcd. for C16H33NO2 C: 70.80%, H: 12.25%, N: 5.16%; Found C: 70.91%, H: 12.70%, N: 5.27%.
2.2 Methods and instrumentation
The FTIR spectra were measured with a Perkin-Elmer (Model Spectrum Rx I) spectrometer. Melting point of solid compounds was measured using Instind (Kolkata) melting point apparatus with open capillaries. The 1H-NMR spectra were recorded on an AVANCE DAX-400 (Bruker, Sweden) 400 MHz NMR spectrometer. All measurements were done at 298 K unless otherwise mentioned.
For FESEM, a small amount of the hot sample solution was placed on the aluminium/copper foil. It was air dried at room temperature for 24 h. A layer of gold was sputtered on top to make conducting surface and finally the specimen was transferred into the Field Emission Scanning Electron Microscope (FESEM, Zeiss, Supra-40) operating at 5–10 kV for getting the micrograph.
The XRD experiment was performed on a Pan analytica X′ Pert pro X-ray diffractometer using Cu target (Cu-Kα) and Ni filter at a scanning rate of 0.001 s−1 between 2 and 12°, operating at a voltage of 40 kV and current 30 mA. XRD spectra were taken at room temperature for all air-dried organogel samples prepared on a glass slide.
Rheology measurements were performed on a Bohlin RS D-100 (Malvern, UK) rheometer using parallel-plate (PP-20) geometry. The gap between the plates was fixed at 100 μm. Organogel was placed on the rheometer and a stress-amplitude sweep experiment was performed at a constant oscillation frequency of 1.0 Hz at 25 °C. From this measurement, a linear viscoelastic region was identified and then a frequency sweep measurement was performed over a wide frequency range 0.01–100 Hz at a particular stress taken from the linear viscoelastic region at 298 K.
Gelation capacity of the amphiphiles was determined in terms of critical gelation concentration (CGC). CGC is defined as the minimum concentration of gelator required to gelate unit volume of solvent at a certain temperature. Gelation was studied by dissolving certain amount of solid gelator in a screw-caped vial in requisite volume of organic solvent by heating in a hot water bath (∼50–80 °C as per solvent's boiling point) and subsequently allowed to cool at 298 K in a temperature controlled water bath (Julabo, Model F12). The gelation was confirmed by the flow to “inversion test” method; it was considered to be a gel when the material did not flow due to gravity upon inversion of the vial.
3. Results and discussion
3.1 Gelation behaviour
The results of gelation studies are summarized in Table 1. It is observed that the NAT amphiphile can gelate aromatic solvents, but NDMAT, as it is a liquid, remained soluble in almost all solvents employed. This is because of the non-hydrogen-bonding ability and steric hindrance of the –N(CH3)2 which affect intermolecular H-bonding between the amide linkages as well as between the amine head-groups. This suggests that an H-bond donor functional group is necessary for gelation by this class of molecules. Indeed, when the CGC values of the gelators in p-xylene solvent are compared it is observed that both NAT and NHT have poor gelation ability compared to that of NCT. This can be attributed to weaker H-bond donating capacity of the –NH2 and –OH groups relative to the –COOH group which can participate in head-to-head and/or sidewise H-bonding interaction between two molecules (Fig. 2). However, it is surprising to see that despite having a –OH group which is a stronger H-bond donor as well as acceptor compared to –NH2 group, the gelation ability of NHT is poor in comparison to NAT. Therefore, it appears that not only gelator–gelator, but also gelator–solvent interactions are important factors for the gelation process. In order to understand the role of gelator–solvent interactions, we have modulated the solvent polarity and its H-bonding ability. Among the aromatic solvents employed, p-xylene is least polar (π* = 0.43) and nitrobenzene is the most polar (π* = 1.01).41 On the other hand, chlorobenzene is the weakest (β = 0.07) H-bond acceptor and nitrobenzene is the strongest (β = 0.39) H-bond acceptor solvent.41 The data in Table 1 suggest that for any of the gelators, gelation is facilitated more in less polar and nonhydrogen-bonding solvent, p-xylene in which gelator–gelator H-bonding interaction is most favoured. Thus, it clearly suggests that H-bonding interaction is the major driving force for gelation in these gelators. In the case of NHT, the H-bonding interaction with solvent molecules being stronger than that with NAT its CGC value is slightly higher than that of the latter.
Table 1 CGC (±0.1% w/v) values of the organogels of NAT, NCT and NHT amphiphiles in organic solvents at 298 K; quantities within parentheses represent corresponding and Tgs (±1 K) values at 0.05 M gelator concentration
Solvent |
NAT |
NCT |
NHT |
Tgs measured at 0.07 M gelator concentration. |
PhH |
1.7 |
1.2 |
2.5 |
p-Ph(Me)2 |
1.4 (313) |
1.1 (336) |
1.9 (315)a |
PhCl |
1.5 |
1.5 |
2.7 |
PhNO2 |
1.6 |
1.2 |
2.5 |
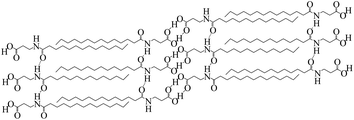 |
| Fig. 2 Head-to-head and sidewise H-bonding interaction between the NCT amphiphiles. | |
3.2 Morphology of the gels
Scanning electron microscopy. The FESEM images of the xerogels of the p-xylene organogels as shown in Fig. 3, clearly exhibit 3D self-assembled fibrillar networks confirming gelation. All the images show ribbon-like lamellar aggregate formation. As can be seen, the fibers formed by NAT are less continuous than those of NCT gelator. On the other hand, the FESEM image of NHT appears as a discreet flake-like structure with the smallest aspect ratio. In other words, the aspect ratio of the ribbons in the organogels of both NAT and NHT gelators are less compared to that of NCT gelator. Thus morphology of the organogels can be correlated with the CGC values of the gelators. The NCT having lowest CGC value the ribbon-like fibres forming 3D gel network have large aspect ratio. On the other hand, the flake-like morphology of NHT organogel is consistent with its poor gelation ability.
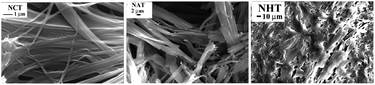 |
| Fig. 3 The FESEM images of the air-dried organogels of p-xylene solvent. | |
3.3 X-Ray diffraction
The X-ray diffraction spectra of the xerogels are shown in Fig. 3. The periodicity of the reflection peaks in the XRD spectrum confirms the presence of bilayer lamellar structures. The bilayer thicknesses taken to be equal to the interlayer distance of 100 planes were found to be smaller than twice the extended chain length of the hydrocarbon tail42 which means the hydrocarbon tails are interdigitated. The related data are collected in Table 2. Although the NAT, NHT, and NCT amphiphiles have the same hydrocarbon chain length (C14), the bilayer thickness increases in the order NCT < NAT < NHT. As reported earlier, the bilayer thickness of NCT is 3.13 nm and its extended tail length is 2.02 nm. Thus the extent of interdigitation in NAT is less (0.66 nm) than that in NCT (0. 91 nm) organogel, suggesting weaker van der Waals interactions among the hydrocarbon chains of NAT or NHT molecules. This is possibly because of tight packing of the hydrocarbon chains (due to stronger H-bonding between amide groups) of adjacent molecules in the self-assembly of NCT gelator. This explains formation of long ribbon-like aggregates in the case of NCT and short flake-like lamellar structures in the cases of NAT and NHT amphiphiles, respectively (Fig. 4).
Table 2 XRD data of the air-dried organogels of NAT and NCT in PhNO2 solvent
Gelator |
lc (nm) |
2θ (degree) |
d (nm) |
Plane |
NAT |
2.02 |
2.61, 7.71 |
3.38 |
100, 300 |
NCT |
2.02 |
2.84, 5.64, 8.41 |
3.14 |
100, 200, 300 |
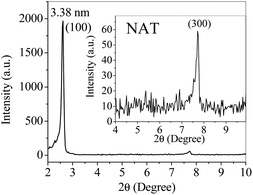 |
| Fig. 4 The X-ray diffraction spectra of the air dried NAT gel in p-Ph(Me)2 solvent. | |
3.4 Thermal stability
The organogels of the amphiphiles were observed to be thermoreversible for several heating–cooling cycles. The gel-to-sol transition temperature (Tgs) of the organogels in p-Ph(Me)2 solvent was determined and are listed in Table 1. For comparison purposes, we have also included the corresponding data for NCT organogel. The Tgs values of the NAT and NCT organogels in p-Ph(Me)2 at the same (0.05 M) gelator concentration are respectively, 313 K and 336 K. The Tgs value of the NHT organogel, even though measured at a higher concentration (0.07 M) is less that of NCT. This means thermal stability of the NCT organogel is much higher than the corresponding amine derivative. The –NHCO– group being common in all the three amphiphiles, the higher thermal stability NCT must be due to the stronger H-bonding capacity of its head-group. Clearly, with the increase of H-bonding strength of the amphiphile's head-group the thermal stability also increases which is consistent with the order (NCT > NAT > NHT) of increase of gelation abilities (CGC, Table 1) of the amphiphiles.
3.5 Mechanical stability
In order to compare the mechanical stability of the organogels, the rheological measurements were performed in a given solvent (p-Ph(Me)2) containing same concentration (0.15 M) of gelator at 298 K. σy values of the organogels of NCT has been compared. The rheological behavior of the p-Ph(Me)2 organogels were studied by measuring storage (G′) and loss (G′′) moduli. The frequency sweep measurements (Fig. 5) at a fixed (13 Pa for NAT and 2 Pa for NHT) amplitude stress showed that for the organogels of NAT and NHT, both G′ and G′′ values are nearly independent of frequency in the range of 0.1–10 Hz which is characteristic of gel structure. NAT amphiphile produced sufficiently stronger gel as indicated by the higher values of G′ compared to G′′. A small dependence of G′ and G′′ on frequency and small values of G′ compared to G′′ in the case of NHT gelator, is indicative of weak gel formation. The rheological response of the gels to the applied shear stress was also examined at a constant frequency. The plots of G′ and G′′ versus applied shear stress at a frequency of 1 Hz are presented in Fig. 6. As can be seen, above a critical stress value the G′-value exhibits a rapid fall indicating flow of the organogel. This critical stress is termed as yield stress (σy). The σy values as obtained from the corresponding plots are 4, 191, and 9445 Pa for NHT, NAT, and NCT organogels, respectively. The highest σy value of the NCT organogel compared to NAT and NHT indicates its highest mechanical strength. On the other hand, the NHT organogel has the lowest σy value and hence it is the weakest. This means that the mechanical strength of the organogels increases in the order of increasing H-bonding strength of the head-group of the amphiphiles. The trend is thus similar to that of thermal stability discussed in the previous section.
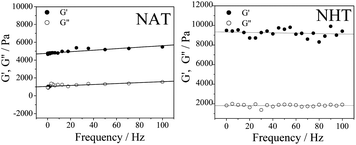 |
| Fig. 5 Variation of storage modulus (G′) and loss modulus (G′′) of the p-Ph(Me)2 organogels of NAT and NHT with the applied frequency (f) at a constant shear stress of 13 Pa for NAT and 2 Pa for NHT at 298 K. | |
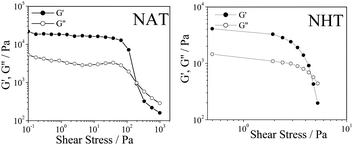 |
| Fig. 6 Variation of storage modulus (G′) and loss modulus (G′′) of the p-Ph(Me)2 organogels of NAT and NHT with the applied shear stress (σ) at a constant frequency of 1 Hz at 298 K. | |
4. Conclusions
In summary, we have investigated the role of intermolecular hydrogen-bonding interaction on gelation properties of four amphiphilic organogelators with amide linkage in the hydrocarbon chain of same length (C14) and with –COOH, –OH, –NH2 or –N(CH3)2 functionalities as head-group. It was surprising to observe that the –NH2 group terminated amide amphiphile NAT also produces gels in aromatic liquids. However, NAT was observed to have gelation abilities, thermal stabilities, and mechanical strengths much less than the corresponding amino acid derivative. This has been ascribed to the poor H-bond donating ability of the –NH2 group in comparison to the –COOH group, which not only acts as a strong H-bond acceptor but also acts as a weak H-bond donor group. The corresponding –OH group terminated amide amphiphile also has gelation capacity much less than the other two gelators as it is mainly strong H-bond donating group. The gelation ability of the amine-terminated amphiphile vanishes when the two H-atoms of the –NH2 group are substituted by the –CH3 groups as a consequence of steric hindrance and destruction of the amide–amide H-bond. It is thus concluded that –COOH group is not mandatory for the gelation to occur. However, the H-bond donor/acceptor ability of the head-group either assists or affects gelation behaviour of the amide amphiphiles. The manifestation of the role of H-bonding interactions can also be seen in the ribbon-like aggregate structures whose aspect ratio increases with the increase of strength of the intermolecular H-bond between head-groups of two gelator molecules. Relatively stronger H-bonding as with –COOH group favours 1D growth of the gel aggregates. The results of this investigation suggests that thermal stability as measured by Tgs value, mechanical strength as measured by σy value and morphology of the organogels are correlated with the gelator–gelator and gelator-solvent H-bonding interactions. In general, the thermal as well as mechanical stability and gel morphology are connected to strength of molecular interactions. However, strong molecular interactions lead to precipitation/crystallization of the gelator molecules. This means only noncovalent interactions of moderate strength are required for physical gelation.
Acknowledgements
The authors acknowledge Indian Institute of Technology, Kharagpur for financial support of this work. RDM thanks CSIR, India for a research fellowship. We thank Mr Kiran Patruni, Department of Agricultural Science, IIT Kharagpur, for his help with the rheological measurements.
Notes and references
- D. J. Abdallah and R. G. Weiss, Adv. Mater., 2000, 12, 1237 CrossRef CAS.
- Y. C. Lin, B. Kachar and R. G. Weiss, J. Am. Chem. Soc., 1989, 111, 5542 CrossRef CAS.
- K. Hanabusa, Y. Naka, T. Koyama and H. Shirai, J. Chem. Soc., Chem. Commun., 1994, 2683 RSC.
- R. Oda, I. Huc and S. J. Candau, Angew. Chem., Int. Ed., 1998, 37, 2689 CrossRef CAS.
- J. H. Jung, Y. Ono, K. Hanabusa and S. Shinkai, J. Am. Chem. Soc., 2000, 122, 5008 CrossRef CAS.
- J. H. Jung, Y. Ono and S. Shinkai, Angew. Chem., Int. Ed., 2000, 39, 1862 CrossRef CAS.
- J. H. Jung, M. Amaike and S. Shinkai, Chem. Commun., 2000, 2342 Search PubMed.
- C. M. Garner, P. Terech, J.-J. Allegraud, B. Mistrot, P. Nguyen, A. de. Geyer and D. Rivera, J. Chem. Soc., Faraday Trans., 1998, 94, 2173 RSC.
- H. T. Stock, N. J. Turner and R. McCague, J. Chem. Soc., Chem. Commun., 1995, 2063 RSC.
- S. Bhattacharya and A. Pal, J. Phys. Chem. B, 2008, 112, 4918 CrossRef CAS PubMed.
- A. Motulsky, M. Lafleur, A.-C. Couffin-Hoarau, D. Hoarau, F. Boury, J.-P. Benoit and J.-C. Leroux, Biomaterials, 2005, 26, 6242 CrossRef CAS PubMed.
- A. C. Couffin-Hoarau, P. D. Motulsky and J. C. Leroux, Pharm. Res., 2004, 21, 454 CrossRef CAS.
- K. Kubo and A. Mori, Chem. Lett., 2005, 1250 CrossRef CAS.
- D. Khatua and J. Dey, Langmuir, 2005, 21, 109 CrossRef CAS PubMed.
- K. G. Ragunathan and S. Bhattacharya, Chem. Phys. Lipids, 1995, 77, 13 CrossRef CAS.
- K. Hanabusa, R. Tanaka, M. Suzuki, M. Kimura and H. Shirai, Adv. Mater., 1997, 9, 1095 CrossRef CAS.
- M. Suzuki, T. Sato, H. Shirai and K. Hanabusa, New J. Chem., 2006, 30, 1184 RSC.
- J. G. Hardy, A. R. Hirst, I. Ashworth, C. Brennan and D. K. Smith, Tetrahedron, 2007, 63, 7397 CrossRef CAS PubMed.
- J. V. Esch, S. DeFeyter, R. M. Kellogg, S. DeSchryver and B. L. Feringa, Chem. – Eur. J., 1997, 3, 1238 CrossRef.
- J. V. Esch, F. Schoonbeek, M. de. Loos, H. Kooijman, A. L. Spek, R. M. Kellogg and B. L. Feringa, Chem. – Eur. J., 1999, 5, 937 CrossRef.
- M. de. Loos, J. Van Esch, R. M. Kellogg and B. L. Feringa, Angew. Chem., Int. Ed., 2001, 40, 613 CrossRef.
- N. Amanokura, K. Yoza, H. Shinmori, S. Shinkai and D. N. Reinhoudt, J. Chem. Soc., Perkin Trans. 2, 1998, 2585 RSC.
- R. J. H. Hafkamp, M. C. Feiters and R. J. M. Nolte, J. Org. Chem., 1999, 64, 412–426 CrossRef CAS.
- A. Srivastava, S. Ghorai, A. Bhattacharjya and S. Bhattacharya, J. Org. Chem., 2005, 70, 6574 CrossRef CAS PubMed.
- S. Bhattacharya and S. N. G. Acharya, Langmuir, 2000, 16, 87 CrossRef CAS.
- S. Bhattacharya and Y. K. Ghosh, Chem. Commun., 2009, 5892 Search PubMed.
- A. Pal, Y. K. Ghosh and S. Bhattacharya, Tetrahedron, 2007, 63, 7334 CrossRef CAS PubMed.
- A. Pal and J. Dey, Langmuir, 2011, 27, 3401–3408 CrossRef CAS PubMed.
- M. Lescanne, P. Grondin, A. d'Aléo, F. Fages, J.-L. Pozzo, O. Mondain Monval, P. Reinheimer and A. Colin, Langmuir, 2004, 20, 3032 CrossRef CAS.
- A. Pal, T. Patra and J. Dey, Chem. Phys. Lett., 2013, 556, 245 CrossRef CAS PubMed.
- X. Luo, Z. Li, W. Xiao, Q. Wang and J. Zhong, J. Colloid Interface Sci., 2009, 336, 803 CrossRef CAS PubMed.
- Y. A. Shchipunov and E. V. Shumilina, J. Colloid Interface Sci., 1993, 161, 125 CrossRef CAS.
- C. L. Zhan, P. Gao and M. H. Liu, Chem. Commun., 2005, 462 RSC.
- J. Becerril, M. I. Burguete, B. Escuder, S. V. Luis, J. F. Miravet and M. Querol, Chem. Commun, 2002, 738 RSC.
- C. Salmi, C. Loncle, N. Vidal, Y. Letourneux and J. M. Brunel, Eur. J. Med. Chem., 2008, 43, 540–547 CrossRef CAS PubMed.
- K. Benner, A. Granzhan, H. Ihmels and G. Viola, Eur. J. Org. Chem., 2007, 2007, 4721 CrossRef.
- H. Haruki, M. Takao and U. Shigeyuki, Jpn. Kokai Tokkyo Koho, 2001, 9 Search PubMed.
- P. T. Eak, B. Daniel, U. Tsunemasa and T. Takashi, Fr. Demande, 1998, 36 Search PubMed; P. T. Eak and S. Fouquay, US Patent, US6365100 B1, 2002.
- N. Yoshio, T. Takao and O. Nobuhiro, Jpn. Kokai Tokkyo Koho, 1994, 10 Search PubMed.
- F. Devinsky, I. Lacko, D. Mlynarcik and L. Krasnec, Chem. Zvesti, 1983, 37, 263 CAS.
- M. J. Kamlet, J.-L. M. Abboud, M. H. Abraham and R. W. Taft, J. Org. Chem., 1983, 48, 2877 CrossRef CAS.
- The hydrocarbon chain length of the amphiphiles were obtained from the energy minimized (using MM2 force field of ChemDraw Ultra 7 software) structures of the amphiphiles; the distance between the α-carbon and the –CH3 group was considered.
|
This journal is © The Royal Society of Chemistry 2014 |